ABSTRACT
The demethylating agent 5-azacytidine (AZA) has proven its efficacy in the treatment of myelodysplastic syndrome and acute myeloid leukemia. In addition, AZA can demethylate FOXP3 intron 1 (FOXP3i1) leading to the generation of regulatory T cells (Treg). Here, we investigated the impact of AZA on xenogeneic graft-vs.-host disease (xGVHD) and graft-vs.-leukemia effects in a humanized murine model of transplantation (human PBMCs-infused NSG mice), and described the impact of the drug on human T cells in vivo. We observed that AZA improved both survival and xGVHD scores. Further, AZA significantly decreased human T-cell proliferation as well as IFNγ and TNF-α serum levels, and reduced the expression of GRANZYME B and PERFORIN 1 by cytotoxic T cells. In addition, AZA significantly increased Treg frequency through hypomethylation of FOXP3i1 as well as increased Treg proliferation. The latter was subsequent to higher STAT5 signaling in Treg from AZA-treated mice, which resulted from higher IL-2 secretion by conventional T cells from AZA-treated mice itself secondary to demethylation of the IL-2 gene promoter by AZA. Importantly, Tregs harvested from AZA-treated mice were suppressive and stable over time since they persisted at high frequency in secondary transplant experiments. Finally, graft-vs.-leukemia effects (assessed by growth inhibition of THP-1 cells, transfected to express the luciferase gene) were not abrogated by AZA. In summary, our data demonstrate that AZA prevents xGVHD without abrogating graft-vs.-leukemia effects. These findings could serve as basis for further studies of GVHD prevention by AZA in acute myeloid leukemia patients offered an allogeneic transplantation.
Abbreviations
AZA | = | azacytidine |
BM | = | bone marrow |
DNMT | = | DNA methyltransferase |
FOXP3 | = | Forkhead box protein P3 |
GVHD | = | graft-vs.-host disease |
GZMB | = | granzyme B |
H&E | = | hematoxylin and eosin |
HCT | = | haematopoietic cell transplantation |
MLR | = | mixed lymphocyte reaction |
NSG | = | NOD-scid IL-2Rγnull |
PB | = | peripheral blood |
PBMC | = | peripheral blood mononuclear cells |
PRF1 | = | perforin-1 |
SPL | = | spleen |
TREG | = | regulatory T cells |
Introduction
Graft-vs.-host disease (GVHD) remains a major limitation of allogeneic haematopoietic cell transplantation (allo-HCT).Citation1 GVHD comprises two syndromes: acute GVHD, which is a deregulated inflammatory response causing skin, gastro-intestinal tract and/or liver damage, and chronic GVHD, which generally occurs beyond day 100 after transplantation, can affect virtually any tissue, and shares many features with autoimmune diseases.Citation2-5 Regulatory T cells (Tregs, defined as CD4+CD25+FOXP3+ T cells) are not only required for the maintenance of tolerance to self-antigens,Citation6-8 but also play a critical role in the control of GVHD.Citation9-14
Treg development and function requires the transcription factor FOXP3. Indeed, mutations in the FOXP3 gene causing Treg deficiency lead to a fatal autoimmune disorder known as the IPEX syndrome.Citation15 However, maintenance of Treg number and function in mouse has been shown to also require the demethylation of genes controlling the expression of molecules implicated in Treg function such as IL2RA (CD25), TNFRSF18 (GITR), CTLA4 or FOXP3 itself.Citation16 Notably, demethylation of a regulatory region located in FOXP3 intron 1 (FOXP3i1) greatly contributes to the stability of FOXP3 expression and to Treg phenotype and functions, both in mice and humans.Citation16-19
Demethylating agents such as 5-azacytidine (AZA) or decitabine are commonly used in the treatment of hematological malignancies characterized by aberrant DNA methylation, such as myelodysplastic syndromes or acute myeloid leukemia.Citation20-22 While AZA has cytostatic activity and induces apoptosis through double break strands in DNA when used at high doses, it incorporates to DNA and decreases DNA methyltransferases (DNMT) levels causing widespread hypomethylation in daughter cells when used at lower doses.Citation23 Importantly, methylation array analyses in patients treated with AZA have revealed a dramatic variability of demethylation responses from one patient to another and a partial restoration of methylation pattern 2 weeks after AZA discontinuation.Citation24
Previous studies have demonstrated that demethylating agents induce demethylation of FOXP3i1 and FOXP3 expression.Citation25-30 Further, AZA prevented acuteCitation25,26 and chronicCitation31 GVHD in mouse-to-mouse models of transplantation. However, in sharp contrast with these results, a study assessing the impact of AZA administration on T cell subsets in patients with myelodysplastic syndrome showed that, while AZA increased FOXP3 expression, it induced cells with a phenotype resembling Treg but that also produced IL-17.Citation32
We and others have studied xenogeneic GVHD (xGVHD) by infusing human peripheral blood mononuclear cells (huPBMC) into NOD-scid IL-2Rγnull (NSG) mice.Citation12,33-39 In that model, human donor T cells react against murine MHC (and particularly donor CD8+ T cells against murine MHC-class 1 molecules).Citation34 This model has many advantages in comparison to classical murine models of GVHD, including donor genetic diversity (which is critical for studying drugs with broad unspecific hypomethylating activity, given their different hypomethylating pattern in different patients), the use of human cells to induce (and control) xGVHD (given important divergences between murine and human immune systems in general and related to mechanisms of FOXP3 expression specifically (nicely reviewed by Ziegler in Ref.Citation40)), and the development of skin fibrosis mimicking chronic GVHD in mice that survive the acute phase of xGVHD.Citation33 Here, we assessed the impact of AZA on xGVHD and on graft-vs.-leukemia effects in NSG mice. Main observations were that AZA mitigated GVHD through multiple mechanisms including (1) anti-proliferative impact on human T cells, (2) reduced pro-inflammatory environment characterized by lower Th1 cytokines and lower expression of each PERFORIN 1 (PRF1) and GRANZYME B (GZMB) by cytotoxic T cells, and (3) promotion of highly suppressive Treg. Treg promotion in AZA-treated animals was due to both demethylation of FOXP3i1 and higher secretion of IL-2 by conventional T cells (Tconv), subsequent to hypomethylation of IL2 promoter site 1.
Results
Impact of AZA on human T cells in vitro
We first investigated the impact of AZA on human T cells in vitro. We observed that AZA significantly reduced T-cell proliferation on day 3, and the frequency of KI67+ T cells on day 4 (92.9 vs. 82.6%, p = 0.0087) [ and ]. However, on day 8, frequencies of KI67+ T cells were increased with AZA (43.8 vs. 57.5%, p = 0.0448), while percentages of KI67+ T cells were low in the two conditions on day 12. Interestingly, treated cells presented a higher activation status (assessed by the co-expression of CD25 and HLA-DR) on days 8 and 12, while MFI of CD25 was significantly higher in AZA-treated than in untreated cells throughout the experiment [ and ]. Altogether, these data suggest that AZA, while initially decreasing T-cell proliferation, later induced an activation-promoting effect.
Figure 1. AZA reduces proliferation but increases activation of T cells and increases Treg frequency in vitro. Fresh T cells from three healthy volunteers were incubated for 12 d in technical duplicates in the presence or not of 1 µM of AZA. (A) CFSE loaded T cells were incubated for 3 d in presence of AZA (bold) or PBS (dashed) and analyzed by flow cytometry (FCM). (B–D) FCM comparisons of KI67+ (B), CD25+HLA-DR+ (C) and CD25 MFI (D) of T cells on days +4, +8, +12 in presence or not of AZA (given from day 0 to day 8). (E) Evolution of CD4+CD25+FOXP3+ Treg cells frequency among cultured CD4+ T cells receiving AZA for 4 d (red), 8 d (green) or PBS for 8 d (blue) on days +4, +8, +12, analyzed by FCM (p-values report the comparison of each time point with day +4 from the corresponding condition). Data show median values of biologic triplicates and technical duplicates (n = 6) with interquartile range (*p < 0.05, **p < 0.005, ***p < 0.0005).
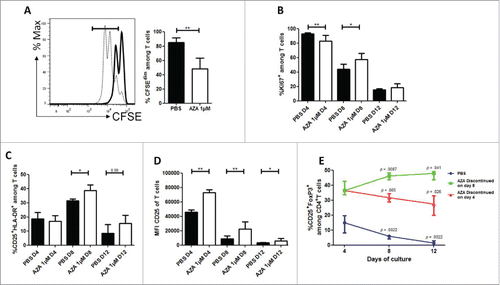
Next, we assessed the impact of AZA on Treg differentiation by using the same experimental setting. As previously reported by other groups of investigators,Citation25,26,30 we observed that AZA dramatically increased the frequencies of CD25 and FOXP3 expressing cells among CD4+ T cells []. To assess the stability of these Tregs, we cultivated T cells without AZA, or with AZA from day 0 to 4, or from day 0 to 8. Interestingly, we observed that the Treg frequency slowly, but significantly, decreased after 4 and 8 d post-discontinuation of AZA (day 4 vs. 8: p = 0.065 and day 4 vs. 12: p = 0.026), although their frequency remained higher than in untreated cells. In contrast, cells treated with AZA during 8 d showed a stable Treg frequency after AZA discontinuation.
Impact of AZA on xGVHD
To investigate the impact of AZA on xGVHD, NSG mice were infused with huPBMC (20 × 106 PBMC i.v.) on day 0 and were then administered with PBS, or with 2 or 5 mg/kg of AZA every 48 h from day +1 to day +21 after transplantation. As shown in , survival was significantly longer in mice receiving 2 or 5 mg/kg of AZA compared with control mice, while mice treated with the 5 mg/kg dose benefited from the best survival, highlighting a dose-response effect (median survival: 100 d (p < 0.0001) vs. 74 d (p = 0.04) vs. 44 d). In agreement with survival data, xGVHD scores were also lower in AZA-treated mice []. Similar observations were made in a second set of experiment using the same experimental design with different donors of PBMCs than in the first experiment but with AZA administered only at 5 mg/kg [ and ]. Histological evaluations were performed on mice killed systematically on day 28 (n = 10). These analyses demonstrated drastically reduced histopathological score in lungs and liver (median lung score 6.75 vs. 0 and median liver score 6.75 vs. 0) of AZA-treated animals compared with controls [ and Table S1]. Further, anti-human CD3+ staining in these organs confirmed strikingly less abundant infiltrates of human T cells in the organs of AZA-treated mice. Similar observations were made in the skin in a separate experiment [Fig. S1].
Figure 2. AZA mitigates xGVHD. NSG mice were injected with 2 × 107 human PBMC i.v. and received or not AZA subcutaneously every 48 h at doses of either 2 or 5 mg/kg starting on day +1 until day +21. (A, B) Survival and GVHD scoring curves of two groups of mice transplanted with two different healthy donors (n = 12/donor) receiving PBS, 2 or 5 mg/kg of AZA (n = 8/condition). (C, D) Pooled survival and scoring curves of two groups of mice transplanted with two different healthy donors (n = 9–10/donor) receiving PBS or 5 mg/kg of AZA (n = 9–10/condition). Mice that reached a score of 6 were estimated to have terminal GVHD and were killed. Final score for dead animals reaching the ethical limit score were kept in the data set for the remaining time points (last observation carried forward). (E) Histological evaluation of tissue lesions (hematoxylin and eosin) and human CD3+ infiltrates in liver and lungs was performed at day 28 post-transplantation. In the control group, lymphoid infiltrates and endotheliitis were observed in the lungs, and portal tract expansion, endotheliitis and parenchymal alterations were observed in the liver (hematoxylin and eosin). In treated mice, both lungs and liver showed only focal infiltrates and overall subnormal histology. Immunostainings with anti-human-CD3 showed a dense infiltrate of donor lymphocytes in both organs in control animals while a very low burden of infiltrate was observed in treated mice.
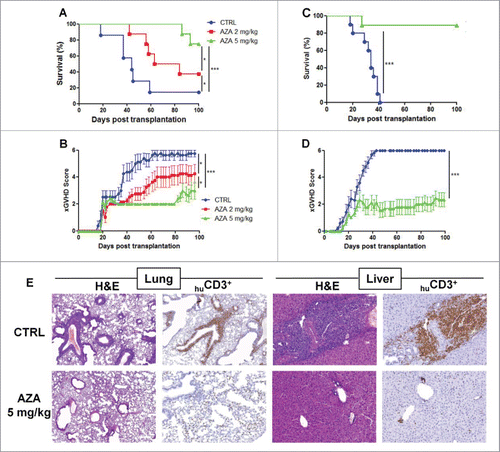
Impact of AZA on human PBMC engraftment
One possible mechanism by which AZA could delay xGVHD development would be to reduce huPBMC engraftment. To assess this hypothesis, immunophenotyping was performed on PB on day 14, as well as on PB, SPL and BM on day 28. Surprisingly, human PB chimerism levels were significantly higher in AZA-treated mice than in control animals on both days 14 and 28 []. These apparently surprising results were due to a greater deleterious impact of AZA on murine CD45+ cells count (because of myelotoxic effects, see Fig. S2) than on human CD45+ cells count []. Interestingly, and while AZA decreased human cells proliferation on day 4 (assessed by CFSE dilution) and 14 (assessed by KI67 expression), the frequency of huCD45+KI67+ in the spleen (SPL) on day 28 was significantly higher in AZA than in control mice [Fig. S3 and ]. This observation is consistent with our in vitro data showing that AZA-treated cells presented a higher proliferation than control cells at late time points.
Table 1. Impact of AZA on engraftment, proliferation and immunomodulation. NSG mice were transplanted with 2 × 107 human PBMC, treated or not with AZA, and FACS was performed on blood cells on day 14 or FACS and RT-qPCR were performed on spleen, blood and bone marrow cells on day 28 after sacrifice. Human chimerism was calculated as the ratio of human CD45+ cells (huCD45+) vs. murine+human CD45+ cells. CFSE dilution of huCD45+ cells was analyzed in spleen, 4 d after transplantation of 4 mice/condition with 2 × 107 CFSE-loaded human PBMCs. Blood absolute counts were calculated based on white blood cell count performed with hematological cell counter (Sysmex) and hu/muCD45+ cell frequency determined by FACS. Transcript levels measured by RT-qPCR were normalized on human housekeeping gene (TBP). Data show median values of 8–10 mice/condition with interquartile range (IQR).
In light of the deleterious impact of AZA on mouse CD45+ cell counts and on previous studies showing that AZA affected human dendritic cells (DC),Citation41 we investigated whether AZA affected murine DC in our model. As showed in Figs. S4 and 5, flow cytometry analysis showed that AZA reduced the frequency of monocytes and DC while did not interfere with their expression of CD86 and MHCII.
Immunomodulatory impact of AZA
To further determine the mechanisms of xGVHD prevention by AZA, immunophenotyping by flow cytometry was performed on day 28 on SPLs from transplanted mice and given or not AZA (5 mg/kg s.c.). To avoid inter-donor variability, this experiment was conducted three times (with three different donors) and only results confirmed in the three experiments were considered as significant (results from the first experiment are shown hereafter as representative example).
In light of the proliferation differences of huCD45+ cells between control and treated mice on day 28, we examined whether the activation state of human T cells was affected by AZA. CD4+ and CD8+ were assayed for their expression of HLA-DR and CD25. Supporting our observation of an activation-promoting effect in vitro, a significantly higher proportion of cells co-expressing HLA-DR and CD25 was observed both in CD4+ and CD8+ T cell subsets from AZA-treated than control mice []. Further, the proportions of CD4+IL-2+ T cells as well as IL2 mRNA levels in splenocytes and serum IL-2 concentration were higher in AZA-treated mice. These data support the hypothesis that AZA promotes T-cell activation.
We next investigated the impact of AZA on specific molecules of helper and cytotoxic T cells. We observed a reduced frequency of IFNγ+ CD4+ T cells, a lower expression of IFNG mRNA as well as a diminished expression of TBET mRNA in AZA-treated mice []. Furthermore, serum IFNγ and TNF-α concentrations were also significantly lower in AZA than in control mice. Interestingly, while GATA3 mRNA expression was also decreased, suggesting decreased Th2-cell frequency, IL-17 as well as RORC mRNA expressions were comparable between control and treated mice.
GZMB secretion by CD8+ T cells has been shown to have a pivotal function in GVHD as GZMB deficiency was found to alleviate cytotoxic T-cell-mediated GVHD in mouse-to-mouse models of GVHD.Citation42 Therefore, we assessed whether AZA could impact the expression of GZMB and PRF1 by CD8+ T cells and we observed lower frequencies of GZMB- and PRF1-secreting CD8+ T cells in mice given AZA compared with control animals [].
Altogether, these data suggest that GVHD prevention by AZA might also involve an immunomodulatory effect on both effector CD4+ and CD8+ T cells.
Impact of AZA on Treg
To assess the impact of AZA on Treg in vivo, we measured FOXP3 mRNA levels by RT-qPCR and proportions of CD4+CD25+FOXP3+ cells by flow cytometry on day 28 in SPL and/or PB of transplanted mice given or not AZA (5 mg/kg). As hypothesized, FOXP3 mRNA levels were increased 2- to 4-folds in AZA-treated mice []. Accordingly, frequencies of CD4+CD25+FOXP3+ T cells in PB and SPL of AZA-treated mice were significantly higher than in control mice [ and ]. Importantly, we also measured the proportions of cells with a demethylated FOXP3i1 region in the SPLs of control and AZA mice on day 28 and observed significantly higher proportions of these cells in AZA-treated than in control mice [].
Figure 3. AZA increases Treg frequency and IL-2-mediated proliferation. Spleen from NSG mice transplanted with 2 × 107 human PBMC and receiving or not AZA 5 mg/kg every 48 h from day +1 to +21 were harvested on day 28 and were homogenized for either flow cytometry or PCR analyses. (A) RT-qPCR comparison of FOXP3 transcript level, normalized on human housekeeping gene (TBP). (B, C) FACS comparison of CD25+FOXP3+ (in spleen and blood) and CD25+CD127lowFOXP3+ (in spleen) cell frequency within the CD4+ T cell population. (D) MS-qPCR assessment of demethylated FOXP3 intron 1 sequences performed on genomic DNA extracted from spleen homogenate of mice treated or not with AZA. (E) FACS comparison of KI67+ cell frequency within regulatory (Treg: CD4+CD25+FOXP3+, in blue) and conventional T cell (Tconv: CD4+FOXP3−, in red) populations. (F) Comparison of ratio, for each mouse treated or not with AZA, of KI67+Treg frequency vs. KI67+ Tconv frequency, based on frequencies found in . (G) FACS comparison of phosphorylated-STAT5 mean fluorescence intensity (MFI) between Treg (in blue) and Tconv (in red) in spleen. (H) Comparison of ratio, for each mouse treated or not with AZA, of pSTAT5 MFI of Treg vs. pSTAT5 MFI of Tconv, based on MFI's found in . Data show median values of 8–10 mice/condition with interquartile range (*p < 0.05, **p < 0.005, ***p < 0.0005).
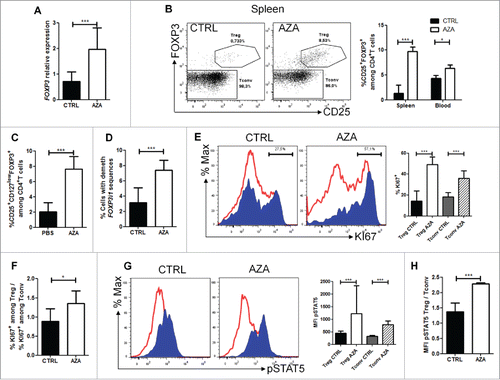
To investigate whether the increased Treg frequency in AZA mice originates only from the hypomethylating effect of the drug or also from a promotion of Treg over Tconv proliferation, we compared the frequency of KI67+ Tconv and Treg in control and AZA-treated mice. As shown in , while the frequencies of KI67+Tconv and KI67+Treg were both significantly higher in AZA-treated than in control mice, the KI67+ Treg:KI67+Tconv ratio was also significantly higher in AZA-treated mice []. Since Treg proliferation is mediated mainly by signaling through the STAT5 pathway after IL-2 binding to its receptor,Citation43 we investigated the STAT5 phosphorylation level in both Treg and Tconv, as described previously.Citation44 In agreement with the higher secretion of IL-2 observed in AZA-treated mice, we observed significantly higher STAT5 phosphorylation levels in Tconv and Treg populations in AZA-treated mice, and to a greater extent in the latter [ and ]. Because the demethylation of a unique site (site 1) in the IL2 promoter is essential for inducing IL2 expression and because AZA has been reported to induce IL2 expression through hypomethylation of this region,Citation45 we investigated the methylation status of this region in genomic DNA extracted from splenocytes of one mouse from each condition. We found that 73.6% vs. 15.7% of sequences were demethylated in AZA-treated vs. control mice. Altogether, these data suggest that AZA mediates Treg proliferation through the promotion of IL-2 secretion by Tconv subsequent to IL2 gene promoter site 1 demethylation.
To determine the suppressive ability of Treg from AZA-treated mice, we sorted CD4+CD25+CD127low T cells (Treg) and CD4+CD25− T cells (Tconvs) from SPL of control and AZA-treated mice killed at day 28. Then, the capacity of Treg to inhibit CD3/CD28-triggered proliferation of CFSE loaded Tconvs was assessed. As shown in and , Treg sorted from AZA-treated mice presented a similar or higher suppressive ability than Treg from control animals. In accordance with these data and in addition to the greater FOXP3 expression and pSTAT5 signaling, a higher expression of HLA-DR, ICOS and IL-35 as well as a diminished expression of GITR were observed in AZA-treated mice [Supplemental Results, Fig. S6 and Table S2].
Figure 4. AZA promotes Treg suppressive activity. (A, B) Representative histograms (A) and comparative panels (B) of the suppression of CD4+CD25+CD127low Treg sorted from spleen of transplanted NSG mice, treated or not with AZA 5 mg/kg, on day 28. Treg were added to autologous CD3/CD28-stimulated Tconvs at a ratio of either 1:1, 1:4 or 1:16 (Treg:Tconv) and proliferation was measured by CFSE dilution, in triplicates. Dotted curve: Tconv alone; tinted curve: Tconv+Treg. Data show mean values of three wells/condition with SEM.
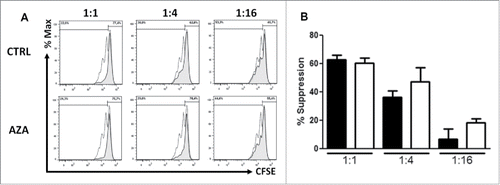
Finally, we assessed the long-term stability of AZA-induced Treg by measuring their frequency in blood of mice included in the second survival curve 60 d post-transplantation. This analysis showed that 3 out of 8 treated mice (all control mice had succumbed before day 60) still presented a Treg frequency in the range observed at day 28 (5–10% of CD4+ T cells). Interestingly, we noticed that on day 60 several mice started or had developed chronic GVHD symptoms (i.e., hair loss and skin fibrosis). Therefore, we classified these eight remaining mice in three groups according to chronic GVHD symptoms: no signs of chronic GVHD, mild signs of GVHD (ruffled fur), or severe signs of chronic GVHD (hair loss and skin sclerosis) and compared the Treg frequency between the three groups. Interestingly, we observed that the mice presenting the highest Treg frequency remained free of chronic GVHD symptoms [ and ]. In contrast, mice with signs of chronic GVHD had either very few or no Treg in their blood on day 60. Altogether, these data suggest that AZA is capable of inducing or promoting long-living Treg, although this was not observed in all treated mice. Further, our data suggest also that Treg persistence might be associated with protection from chronic GVHD.
Figure 5. Absence of « chronic » GVHD development in AZA-treated mice is associated with high Treg frequency. NSG mice transplanted with 2 × 107 human PBMC and receiving or not AZA 5 mg/kg were monitored for survival and GVHD score ( and ). On day 60 post-transplantation, blood was harvested from surviving mice (only AZA-treated animals) and flow cytometry was performed to evaluate Treg frequency. (A) Mice were segregated in three groups according to « chronic » GVHD symptoms and Treg frequency was compared between the groups. (B) Frequency of Treg in each mouse of the three groups.
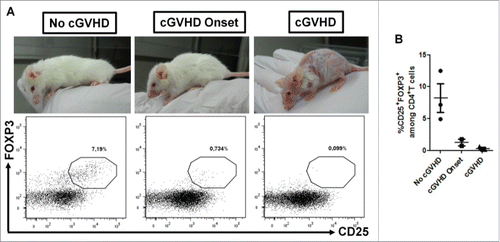
AZA promotes long-term tolerance
To determine the impact of AZA on long-term tolerance, NSG mice, irradiated with 2.5 Gy on day −1, received a secondary transplant with 4 × 106 human cells obtained from the SPL of control or AZA-treated mice, killed at day 28. AZA was not administered after second transplantation. As shown in , all mice given human cells from AZA mice survived beyond day 100, while all mice given human cells from control mice died of GVHD within 40 d. Further, in comparison to mice given human cells from control mice, those given cells from AZA mice had significantly lower GVHD clinical scores and much higher Treg frequencies, demonstrating that Treg induced by AZA were stable and kept mostly a Treg phenotype [ and ].
Figure 6. AZA induces long-term tolerance. Spleen from NSG mice transplanted with 2 × 107 human PBMC and receiving or not AZA 5 mg/kg were harvested on day 28, were homogenized and pooled (CTRL with CTRL and AZA with AZA). Spleen homogenates were either infused per se to 2.5Gy-irradiated NSG mice (4 × 106 huCD45+ cells/mouse), or were depleted of human CD25+ cells before infusion (4 × 106 huCD45+ cells/mouse). (A, B) Survival and GVHD scoring curves (n = 6/condition) of mice transplanted with total spleen homogenates. (C) FACS comparison of CD25+FOXP3+ cell frequency within the CD4+ T cell population in blood at 14 and 40 d post-transplantation. (D) Survival curve (n = 6–7/condition) of mice transplanted with either total or CD25+-depleted spleen homogenates. (E) FACS comparison of CD25+FOXP3+ cell frequency within the CD4+ T cell population in blood at 14 d post-transplantation. Data show median values of 6–7 mice/condition with interquartile range (*p < 0.05, **p < 0.005, ***p < 0.0005).
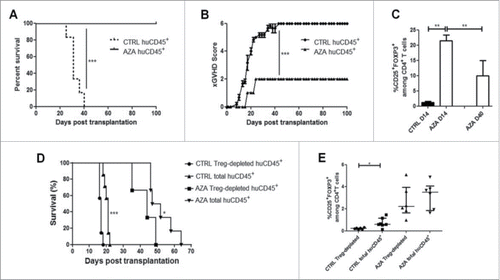
We next examined the implication of Treg in this long-term tolerance by depleting CD25+ cells from human CD45+ cells before secondary transplant. In agreement with previous reports,Citation46 we found that the depletion of CD25+ cells from the secondary graft increased xGVHD lethality in comparison with the undepleted group, demonstrating that Treg play a protective role in this setting. In line with this observation, a significant reduction of survival was also observed in mice transplanted with CD25+-depleted cells from AZA-treated mice, in comparison with mice receiving total CD45+ cells []. However, and surprisingly, the Treg frequency at day 14 was not significantly different between CD25-depleted and total CD45+ groups in the AZA condition, while it was reduced in CD25-depleted group in control condition []. Altogether, these data indicate that AZA-generated Tregs play a protective role in xGVHD and favor long-term tolerance and that AZA-induced genomic hypomethylations preserve a long-term Treg-promoting effect, even when Treg were depleted from the secondary graft.
AZA impact on graft-vs.-leukemia effect
We next investigated whether the immunomodulatory effects of AZA impacted graft-vs.-leukemia effect. Using THP-1 leukemia cells (a cell line sensitive to AZACitation47), transfected to express luciferase, we evaluated the elimination of leukemia cells after transplantation of NSG mice with PBMCs in combination or not with AZA administration, by in vivo bioluminescent imaging. Better survival rates were found in mice receiving AZA treatments (reflecting the inhibition of THP-1 growth by AZA on the one hand and xGVHD prevention by AZA on the other hand), regardless of PBMC administration, while all mice receiving PBMCs without AZA died from GVHD and mice receiving THP-1 cells without AZA or PBMC died from leukemia []. Accordingly, at day 28, tumors were observed only in mice having received THP-1 cells alone []. At day 49, we observed a relapse of leukemia in mice treated with AZA alone while no tumors were found in mice receiving PBMCs+AZA, demonstrating that AZA does not abrogate the capacity of immune cells to clear tumor cells (graft-vs.-leukemia effects) and that the anti-leukemic effects of AZA were not sustained after AZA discontinuation. Specifically, 6/6 mice transplanted with THP-1 cells and treated with AZA without PBMC infusion presented important tumor burden in BLI examination within the last 7 d before their sacrifice for terminal AML and 7/7 mice having received THP-1 + PBMCs + AZA presented no signs of tumors before sacrifice for terminal chronic GVHD or at the end of the experiment [Fig. S7A]. Further, PBMCs + AZA mice had significantly better survival than AZA mice (86 vs. 114 days, p = 0.047) []. To assess whether PBMCs injected in mice having received THP-1+PBMCs+AZA were still capable to mediate graft-vs.-leukemia effect at the end of experiment (day 130), we transplanted these mice, together with untreated age-matched NSG mice (n = 3), with 1 × 106 THP-1-luc cells in matrigel. BLI showed that tumor progression was either inhibited or decreased in PBMCs+AZA mice while a rapid progression was observed in control animals [Fig. S7B]. This clearly indicates that T cells from AZA-treated mice kept the possibility to mediate graft-vs.-leukemia effects a long time after AZA discontinuation.
Figure 7. AZA treatment maintains graft-vs.-leukemia effect. NSG mice were transplanted with 2 × 107 human PBMC together or not with 3 × 106 THP-1-luc cells. Mice were either treated or not with AZA 5 mg/kg and survival (A) and bioluminescence (B) were monitored. Actual images of one representative mouse from each group are shown with Y-axis indicating photon flux (photons/sec) measured from the ventral view with a region of interest drawn over the entire body of each mouse. Right panels show the comparison of bioluminescence between groups. Data show median values of 6–7 mice/condition with interquartile range (*p < 0.05, **p < 0.005, ***p < 0.0005).
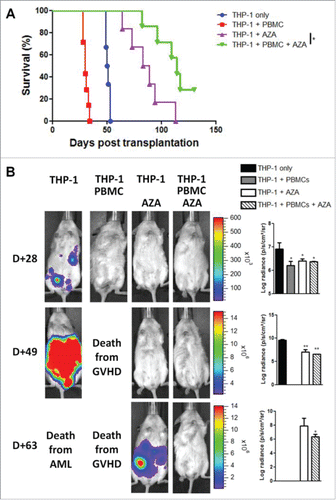
To confirm these results and better mimic the clinical setting of allo-HSCT (in which the GVHD and graft-vs.-leukemia reactions are often directed toward similar antigens), we assessed the impact of AZA on xGVHD and graft-vs.-leukemia effects in NSG mice expressing the human HLA-A0201 antigen (NSG-HLA-A2/HHD), which is also expressed by THP-1 cells. First, we assessed whether AZA ameliorated xGVHD in that model and observed that indeed AZA decreased xGVHD also in NSG-HLA-A2/HHD mice injected with human PBMC (from a human donor non HLA-A2) [ and ]. Then, we repeated the experiment performed in (by using identical experimental design) and found that NSG-HLA-A2/HHD given the combination of AZA and PBMCs presented a reduced tumor burden and significantly higher survival rates than mice given AZA only [ and ]. While tumors were not eradicated by AZA in this experiment, these data are in agreement with observations done in the classical NSG mice, showing that AZA maintained at least partly the graft-vs.-leukemia activity of T cells.
Figure 8. AZA treatment maintains graft-vs.-leukemia effect in NSG-HLA-A2/HHD mice. (A, B) NSG-HLA-A2/HHD mice were transplanted with 2 × 107 human PBMC i.v. and were treated or not with AZA subcutaneously every 48 h at doses of 5 mg/kg starting on day +1 until day +21. Survival (A) and xGVHD scores (B) were monitored (n = 5–6 / condition). (C, D) NSG-HLA-A2/HHD mice were transplanted with 3 × 106 THP-1-luc cells together or not with 2 × 107 human PBMC. Mice were either treated or not with AZA 5 mg/kg and survival (C) and bioluminescence (D) were monitored. Actual images of one representative mouse from each group are shown with Y-axis indicating photon flux (photons/sec) measured from the ventral view with a region of interest drawn over the entire body of each mouse. Right panels show the comparison of bioluminescence between groups. Data show median values with interquartile range (*p < 0.05, **p < 0.005, ***p < 0.0005).
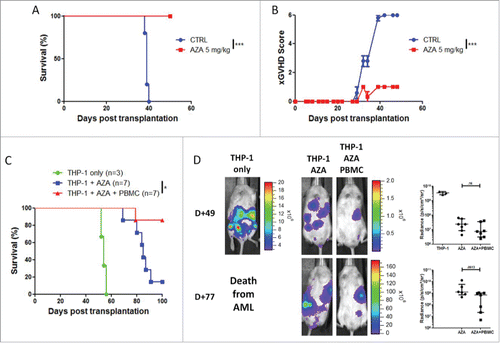
Given that NK cells were previously reported to play an important role in GVL effects,Citation48,49 we sought to investigate whether these cells played a significant role in THP-1 cells elimination in NSG mice injected with human PBMCs and whether AZA may have an impact on these cells in this setting. We first investigated the susceptibility of THP-1 cells to NK cells-mediated lysis by performing cytotoxicity and degranulation assays with the NK92 cell line. We found that THP-1 cells were poorly susceptible to cell lysis compared with the K562 cell line (used as positive control of NK cytotoxicity) [Fig. S8A]. Next, we assessed whether AZA treatment of THP-1 cells could impact the capacity of NK cells to mediate their lysis and found no significant effect of AZA [Fig. S8B]. Finally, we treated the NK92 cells with AZA or not and assessed their cytotoxicity toward K562 [Fig. S8C] and THP-1 [Fig. S8D] cells and found no significant impact of AZA on their lysis capacity. Finally, and while NK cells poorly engraft in PBMCs-infused NSG mice,Citation34,46 we evaluated the impact of AZA on the expression of three NK cell genes: KLRC4 (encoding NKG2D), NCR1 (encoding NKp46) and NCR3 (encoding NKp30) in SPLs of mice, treated or not with AZA, at day 28. As showed in Fig. S9, the expression of KLRC4 and NCR1 were decreased by AZA, while the expression of NCR3 was unchanged, suggesting that AZA decreased the engraftment of NK cells. Altogether, these results suggest that NK cells probably play a minor role in GVL effects against THP-1 cells in AZA-treated NSG mice.
Finally, we examined the impact of AZA on graft-vs.-leukemia effect independently of leukemia cells exposure to AZA. NSG mice, transplanted with PBMCs and treated or not with AZA, were killed at day 28 and human CD45+ cells were sorted from their SPL. Then, 4 × 106 of these cells were injected to irradiated NSG mice, co-injected with THP-1-luc cells [Fig. S10A]. AZA was not administered after this second transplantation. Survival and in vivo luciferase bioluminescence were monitored during the experiment. As showed in Figs. S10B and C, no significant difference of either survival or bioluminescence were found between mice receiving THP-1 alone or THP-1 in combination with AZA-conditioned human CD45+ cells. Altogether, these results highlight the important tolerogenic effects of AZA in secondary transplant experiment but may indicate, at least in part, a deleterious impact of AZA on graft-vs.-leukemia effect in that artificial transplantation setting.
Discussion
Hypomethylating agents, such as AZA, are highly active against acute myeloid leukemia or myelodysplastic cells,Citation20 and, in addition, prevented GVHD by favoring FOXP3 demethylation and the generation of Treg in mouse-to-mouse models of GVHD.Citation25,26 In the present report, we investigated the impact of AZA on xGVHD in a humanized mouse model of GVHD in which xGVHD is induced by transplanting human PBMC in NSG mice.Citation12,13,35 This model tackles several important limitations of mouse to mouse models of GVHD since it allows (1) a genetic diversity of effector cells (this is a crucial point for studying agents with a wide demethylating activity), (2) the use of human cells to induce (and control) GVHD (this is particularly relevant in this study given the important difference of FOXP3 regulation in mice and humansCitation40), (3) the use of effectors immune cells from adults that have been previously exposed to pathogens, (4) to induce GVHD without the need of intense conditioning regimen and (5) the development of chronic GVHD in mice that survive the acute phase of the disease (as observed in humans). Previous studies using that model demonstrated that blockade of TNF-α,Citation34,46 blockade of T-cell proliferation,Citation46 JAK2 and aurora kinase 2 inhibition,Citation50 as well as administration of TregCitation12,13,35 each prevented or ameliorated xGVHD. In the current study, we first demonstrated that AZA mitigated GVHD and promoted long-term tolerance. Further, we identified several mechanisms probably explaining these findings: (1) partial inhibition of human T-cell proliferation by AZA, (2) partial inhibition of Th1 differentiation by AZA, (3) reduced expression of cytotoxic effector molecules by CD8+ T cells in AZA mice, and last but not least (4) Treg promotion by AZA. In addition, and most importantly, we demonstrated that AZA did not abrogate graft-vs.-leukemia effects.
A phase II clinical study of AZA administration after alemtuzumab-based reduced-intensity allo-HCT has suggested that AZA might decrease the incidence of acute GVHD through Treg promotion.Citation27 However, patients included in that study also received 50 mg of alemtuzumab in the conditioning regimen (which is associated with a very low incidence of cGVHD by itselfCitation51), making difficult to draw definitive conclusions on the impact of AZA on GVHD in humans. Treg induction by AZA in that phase II study was not durable, perhaps due to the schedule of AZA administration or to the concomitant use of cyclosporine. A first important observation of the present study was thus that AZA prevented GVHD in a humanized mouse model. The impact of AZA was impressive with very significantly higher survival and lower clinical and pathological xGVHD scores among AZA-treated mice. These data are in line with previous observations made in mouse-to-mouse models of GVHD.Citation25,26,31
A first mechanism possibly explaining xGVHD protection by AZA might be an AZA-induced T-cell proliferation inhibition. Indeed, we observed significant lower counts of huCD45+ cells on day 14 after transplantation, suggesting an anti-proliferative impact of AZA, that was further confirmed by in vivo and in vitro CFSE dilution experiments and KI67 expression.
AZA has also been previously shown to reduce T-cell activation in vitro after 48 h of culture but not at later time points when AZA was found to increase T-cell activation.Citation25,30,52 In line with these findings, we observed that AZA had a pro-activation activity both in vitro after 8 d of culture and in vivo on day 28 after huPBMC injection (7 d after last AZA administration). Interestingly, this higher activation status of T cells in treated mice was not characterized by a pro-inflammatory environment but rather by decreased Th1 differentiation, decreased expression of cytotoxic effector molecules (PRF1 and GZMB) and reduced serum level of proinflammatory cytokines such as IFNγ and TNF-α. Furthermore, we found that AZA did not affect Th17 cells while dramatically increasing Treg frequency, therefore increasing the Treg/Th17 ratio that has been shown to correlate with GVHD improvement.Citation53
As hypothesized, we observed higher Treg frequencies in AZA-treated mice. Interestingly, our data suggest that the cause of this increased Treg frequency was probably multifactorial. Indeed, while a higher frequency of cells with a demethylated FOXP3i1 was observed in AZA mice (suggesting conversion of Tconv into Treg), we also demonstrated higher Treg proliferation in AZA-treated mice together with higher levels of pSTAT5. Given the thigh link between IL-2 and Treg STAT5-dependent proliferation/survival,Citation54-57 these observations suggest higher IL-2 levels in AZA-treated mice. Accordingly, we could demonstrate that AZA-treated mice presented a higher secretion of IL-2 by helper T cells than control animals, concomitant with a higher demethylation status of IL2 gene promoter. This later observation is consistent with previous reports demonstrating that IL2 gene expression is upregulated by its promoter hypomethylation and that AZA promotes IL-2 secretion.Citation31,45,58,59 Importantly, we observed that Treg sorted from AZA-treated mice presented a higher suppressive activity in MLR than Treg from control mice perhaps as a result of higher STAT5 phosphorylation level (highlighting higher IL-2 signaling), and a higher expression of both HLA-DR and ICOS, two markers of activation expressed by highly suppressive Treg.Citation60,61
Very few data are currently available about the long-term stability of AZA-induced Treg. In the present report, we observed that AZA-induced Treg frequency slowly decreased after AZA discontinuation in vitro, in accordance with previously published data in allo-HCT recipients.Citation27 However, Treg levels remained stable in 3 out of 8 mice more than 30 d after discontinuation of AZA administration. Further, and interestingly, in contrast to the five remaining mice that lost the majority, or all, human Treg, the three mice with high Treg levels remained free of chronic GVHD. We also observed the persistence of a high frequency of Treg (and a protection from xGVHD) in secondary transplant performed with huPBMC harvested from the SPLs of AZA-treated mice. Further, the depletion of Tregs before secondary transplantation highlighted that Treg play a protective role from GVHD in that model as previously demonstrated. Interestingly, their high frequency in animals receiving Treg-depleted CD45+ cells from AZA-treated animals suggested that AZA induced long-term hypomethylation signature promoting Treg differentiation.
Finally and most importantly, we investigated the impact of AZA on graft-vs.-leukemia effects. Indeed, while several approaches allowed separating graft-vs.-leukemia effects from GVHD in mouse-to-mouse models of GVHD, very few of them have been successfully translated in the clinic. Although prophylactic AZA administration has been studied in phase II studies after allo-HCT, its net impact on graft-vs.-leukemia effects in humans has remained unsolved. On the one hand, AZA induces the expression of tumor antigens by AML cells leading to the generation of donor-derived tumor-specific cytotoxic T cells that have been shown to protect from AML relapse.Citation27,62 On the other hand, the tolerogenic effects of AZA might predict a negative impact of AZA on graft-vs.-leukemia effects given the well-known link between GVHD and graft-vs.-leukemia effects in humans.Citation1,63,64 In support of this hypothesis, post-transplant AZA administration was associated with a higher proportion of patients with persistent mixed chimerism in the study by Goodyear et al.Citation27 In the current study, using an AZA-sensitive AML cell line, we observed that the best survival was achieved in mice treated with AZA and human PBMC, this approach allowing efficient GVHD prevention by AZA without (the complete) loss of the graft-vs.-leukemia effects induced by human PBMC. However, we found a complete abrogation of graft-vs.-leukemia effects in secondary transplants as mice transplanted with AZA-conditioned CD45+ cells failed to eliminate tumor cells. In light of the almost complete absence of GVHD and the very high Treg frequencies in these mice, we assume that this absence of graft-vs.-leukemia effects reflect the very high tolerogenic impact of AZA in that transplantation setting.
In conclusion, our findings indicate that AZA might be a promising option for separating GVHD from graft-vs.-leukemia effects in humans in patients with AZA-sensitive leukemic cells.
Material and methods
All experimental procedures and protocols used in this investigation were reviewed and approved by the Institutional Animal Care and Use Committee of the University of Liège, Belgium. The “Guide for the Care and Use of Laboratory Animals,” prepared by the Institute of Laboratory Animal Resources, National Research Council, and published by the National Academy Press, was followed carefully as well as European and local legislation. Animal welfare was assessed at least once per day with humane endpoints applied when necessary as described in the ethical form.
Induction of xenogeneic GVHD in NSG mice and AZA administration
NOD-scid IL-2Rγnull (NSG) mice (The Jackson laboratory, Bar Harbor, ME) and NSG mice expressing the HHD construct designed for the expression of human HLA-A0201 covalently bound to human β2 microglobuline (NSG-HLA-A2/HHD) miceCitation65 (The Jackson laboratory), aged from 8 to 10 weeks, were given an i.v. injection of 2 × 107 huPBMC (obtained from buffy coats from healthy volunteers) to induce xGVHD. GVHD severity was assessed by a scoring system that incorporates four clinical parameters: weight loss, posture (hunching), mobility and anemia. Each parameter received a score of 0 (minimum) to 2 (maximum). Mice were assessed for GVHD score thrice weekly and monitored daily during the experiments. Mice reaching a GVHD score of 6/8 or losing 20% of body weight were euthanized in agreement with the recommendation of our ethical committee. Final scores for dead animals reaching the ethical limit score were kept in the data set for the remaining time points (last value carried forward). AZA (Sigma Aldrich), dissolved and diluted in sterile PBS, was administered subcutaneously every 48 h at doses of either 2 or 5 mg/kg starting on day +1 until day +21.
Flow cytometry
At the time of necropsy, peripheral blood (PB), SPL and bone marrow (BM) were harvested and analyzed by flow cytometry. Splenocytes were obtained by crushing the SPL, and BM cells by flushing femurs and tibiae. Cells were counted with a Sysmex XS-800i®. PB was depleted of erythrocytes using RBC lysis buffer (eBioscience, San-Diego, CA) according to manufacturer's instructions. The following antibodies specific for human antigens were used: anti-CD3-V500 (SP34–2, BD); anti-CD4-efluor 450 (RPA-T4); anti-CD4-PerCP (SK3, BD); anti-CD8-FITC (HIT8a); anti-CD8-APC-Cy7 (SK1, BD); anti-CD45-PerCP (2D1, BD); anti-CD45-BV510 (HI30, BD); anti-CD45-PE-Cy7 (HI30); anti-CD25-BV421 (M-A251, BD); anti-CD25-PE-Cy7 (BC96); anti-CD127-biotin (RDR5); anti-HLA-DR-APC-efluor780 (LN3), anti-GITR-APC (eBioAITR), anti-ICOS-APC (ISA-3), anti-FOXP3-AlexaFluor488 (206D and 259D, Biolegend, San-Diego, CA), anti-KI67-APC (B56, BD), anti-CTLA-4-PE (14D3), anti-phosphoSTAT5-APC (pY694, BD), anti-GRANZYME B-PE (GB11, BD), anti-PERFORIN-1-PerCP-Cy5.5 (δG9, BD), anti-IL-2-V450 (MQ1–17H12), anti-IL10-PE (JES3–9D7), anti-IL-17-APC (eBio64DEC17), anti-IFNg-PE-Cy7 (4S.B3) and the following antibodies specific for mouse antigens were used: anti-CD45-PerCP-Cy5.5 (30-F11), CD11b-APC (M1/70), Ly-6G-FITC (RB6–8C5), CD11c-eFluor450 (N418), CD86-biotin (GL1) and MHCII-PE (M5/114.15.2) (all eBioscience unless indicated otherwise). Cells (1.5–2 × 106 cells/sample) were incubated with surface antibodies for 20 min at 4°C in the dark and washed twice with PBS/3% FBS (Lonza). Intracellular staining for FOXP3 (206D), KI67, CTLA-4, PRF1, GZMB and cytokines was performed by using the Transcription Factor Buffer Set (Becton-Dickinson, Bedford, MA). For pSTAT5 and FOXP3 (259D) staining, the PerFix EXPOSE reagent kit (Beckman Coulter, Fullerton, CA) was used as previously reported.Citation44 For intracellular cytokine staining, SPL homogenates were stimulated for 6 h in RPMI supplemented with 10% FBS, penicillin (100 U/mL), streptomycin (10 mg/mL) and in presence of PMA/ionomycin, brefeldin A and monensin (Cell Stimulation Cocktail + Protein Transport Inhibitors, eBioscience). Data were acquired on a FACS Canto II flow cytometer (Becton Dickinson) and analyzed with the Flowjo software 7.0 (Tree Star Inc., Ashland, OR).
Methods for cell culture, mixed lymphocytes reactions, RT-qPCR, methylation analyzes, histology, assessment of serum cytokine levels, bioluminescence, and statistical analyses are described in the supplemental material and method section of the article. The data sets supporting the conclusions of this article are included within this article and its additional files.
Disclosure of potential conflicts of interest
No potential conflicts of interest were disclosed.
Author contributions
Study design: FBa, GE, SHB; Data analyses: GE; Mouse experiments: GE, GF, MH, LD, SD, PD; Flow cytometry design/analyses: GE, SHB; Cell culture, RT-qPCR and IL2 promoter methylation sequencing: GE, LD, GF. FOXP3i1 sequences methylation: SDH, SL; Histology: LdL; Ms writing: GE, FBa, and SHB; Manuscript editing and approval of the manuscript: all authors.
Supplementary_materials.docx
Download MS Word (8 MB)Acknowledgments
We are very grateful to Sandra Ormenese and Raafat Stephan from the Imaging and Flow Cytometry Platform of the GIGA for help with flow cytometry analyses, to the Genomics platform of the GIGA for sequencing and to Emmanuel Di Valentin from the Viral Vectors Platform of the GIGA for help with THP-1 cells transfections. The authors are also grateful to Coline Daulne, for excellent technical assistance. GE, GF and LD are Télévie Research Assistants, and SL and FB are senior research associates of the National Fund for Scientific Research (FNRS) Belgium. SHB is funded by the Fonds Wetenschappelijk Onderzoek (FWO).
Funding
This work was supported by the National Fund for Scientific Research (FNRS) under grant T.0069.15; the Leon Fredericq fund and Anti-Cancer Center at the University of Liège.
References
- Baron F, Labopin M, Niederwieser D, Vigouroux S, Cornelissen JJ, Malm C, Vindelov LL, Blaise D, Janssen JJ, Petersen E et al. Impact of graft-versus-host disease after reduced-intensity conditioning allogeneic stem cell transplantation for acute myeloid leukemia: A report from the acute leukemia working party of the European group for blood and marrow transplantation. Leukemia 2012; 26:2462-8; PMID:22699419; https://doi.org/10.1038/leu.2012.135
- Blazar BR, Murphy WJ, Abedi M. Advances in graft-versus-host disease biology and therapy. Nat Rev Immunol 2012; 12:443-58; PMID:22576252; https://doi.org/10.1038/nri3212
- Socie G, Ritz J. Current issues in chronic graft-versus-host disease. Blood 2014; 124:374-84; PMID:24914139; https://doi.org/10.1182/blood-2014-01-514752
- Servais S, Beguin Y, Delens L, Ehx G, Fransolet G, Hannon M, Willems E, Humblet-Baron S, Belle L, Baron F. et al. Novel approaches for preventing acute graft-versus-host disease after allogeneic hematopoietic stem cell transplantation. Expert Opin Investig Drugs 2016; 25(8)957-72; PMID:27110922; https://doi.org/10.1080/13543784.2016.1182498
- Teshima T, Reddy P, Zeiser R. Acute graft-versus-host disease: Novel biological insights. Biol Blood Marrow Transplant 2016; 22:11-6; PMID:26453971; https://doi.org/10.1016/j.bbmt.2015.10.001
- Sakaguchi S, Sakaguchi N, Shimizu J, Yamazaki S, Sakihama T, Itoh M, Kuniyasu Y, Nomura T, Toda M, Takahashi T. Immunologic tolerance maintained by CD25+ CD4+ regulatory T cells: Their common role in controlling autoimmunity, tumor immunity, and transplantation tolerance (review). Immunol Rev 2001; 182:18-32; PMID:11722621; https://doi.org/10.1034/j.1600-065X.2001.1820102.x
- Miyara M, Ito Y, Sakaguchi S. TREG-cell therapies for autoimmune rheumatic diseases. Nat Rev Rheumatol 2014; 10:543-51; PMID:24980140; https://doi.org/10.1038/nrrheum.2014.105
- Kuswanto W, Burzyn D, Panduro M, Wang KK, Jang YC, Wagers AJ, Benoist C, Mathis D. Poor repair of skeletal muscle in aging mice reflects a defect in local, interleukin-33-dependent accumulation of regulatory T cells. Immunity 2016; 44:355-67; PMID:26872699; https://doi.org/10.1016/j.immuni.2016.01.009
- Cohen JL, Trenado A, Vasey D, Klatzmann D, Salomon BL. CD4(+)CD25(+) immunoregulatory T Cells: New therapeutics for graft-versus-host disease. J Exp Med 2002; 196:401-6; PMID:12163568; https://doi.org/10.1084/jem.20020090
- Edinger M, Hoffmann P, Ermann J, Drago K, Fathman CG, Strober S, Negrin RS. CD4+CD25+ regulatory T cells preserve graft-versus-tumor activity while inhibiting graft-versus-host disease after bone marrow transplantation. Nature Medicine. 2003 (9):1144-50; PMID:12925844; https://doi.org/10.1038/nm915
- Nguyen VH, Zeiser R, Dasilva DL, Chang DS, Beilhack A, Contag CH, Negrin RS. In vivo dynamics of regulatory T-cell trafficking and survival predict effective strategies to control graft-versus-host disease following allogeneic transplantation. Blood 2007; 109:2649-56; PMID:17095616; https://doi.org/10.1182/blood-2006-08-044529
- Hannon M, Lechanteur C, Lucas S, Somja J, Seidel L, Belle L, Bruck F, Baudoux E, Giet O, Chantillon AM et al. Infusion of clinical-grade enriched regulatory T cells delays experimental xenogeneic graft-versus-host disease. Transfusion 2014; 54:353-63; PMID:23772685; https://doi.org/10.1111/trf.12666
- Martelli MF, Di Ianni M, Ruggeri L, Falzetti F, Carotti A, Terenzi A, Pierini A, Massei MS, Amico L, Urbani E et al. HLA-haploidentical transplantation with regulatory and conventional T cell adoptive immunotherapy prevents acute leukemia relapse. Blood 2014; 124:638-44; PMID:24923299; https://doi.org/10.1182/blood-2014-03-564401
- Brunstein CG, Miller JS, Cao Q, McKenna DH, Hippen KL, Curtsinger J, Defor T, Levine BL, June CH, Rubinstein P et al. Infusion of ex vivo expanded T regulatory cells in adults transplanted with umbilical cord blood: Safety profile and detection kinetics. Blood 2011; 117:1061-70; PMID:20952687; https://doi.org/10.1182/blood-2010-07-293795
- Bennett CL, Christie J, Ramsdell F, Brunkow ME, Ferguson PJ, Whitesell L, Kelly TE, Saulsbury FT, Chance PF, Ochs HD. The immune dysregulation, polyendocrinopathy, enteropathy, X-linked syndrome (IPEX) is caused by mutations of FOXP3. Nat Genet 2001; 27:20-1; PMID:11137993; https://doi.org/10.1038/83713
- Ohkura N, Kitagawa Y, Sakaguchi S. Development and maintenance of regulatory T cells. Immunity 2013; 38:414-23; PMID:23521883; https://doi.org/10.1016/j.immuni.2013.03.002
- Baron U, Floess S, Wieczorek G, Baumann K, Grutzkau A, Dong J, Thiel A, Boeld TJ, Hoffmann P, Edinger M et al. DNA demethylation in the human FOXP3 locus discriminates regulatory T cells from activated FOXP3(+) conventional T cells. Eur J Immunol 2007; 37:2378-89; PMID:17694575; https://doi.org/10.1002/eji.200737594
- Stockis J, Fink W, Francois V, Connerotte T, de Smet C, Knoops L, van der Bruggen P, Boon T, Coulie PG, Lucas S. Comparison of stable human Treg and Th clones by transcriptional profiling. EurJ Immunol 2009; 39:869-82; PMID:19224638; https://doi.org/10.1002/eji.200838807
- Zheng Y, Josefowicz S, Chaudhry A, Peng XP, Forbush K, Rudensky AY. Role of conserved non-coding DNA elements in the Foxp3 gene in regulatory T-cell fate. Nature 2010; 463:808-12; PMID:20072126; https://doi.org/10.1038/nature08750
- Navada SC, Steinmann J, Lubbert M, Silverman LR. Clinical development of demethylating agents in hematology. J Clin Invest 2014; 124:40-6; PMID:24382388; https://doi.org/10.1172/JCI69739
- Scott BL, Deeg HJ. Myelodysplastic syndromes. Annu Rev Med 2010; 61:345-58; PMID:20059342; https://doi.org/10.1146/annurev.med.051308.132852
- Woo J, Deeg HJ, Storer B, Yeung C, Fang M, Mielcarek M, Scott BL. et al. Factors determining responses to azacitidine in patients with MDS and AML with early post-transplant relapse: A prospective trial. Biol Blood Marrow Transplant 2016; 23:176-9; PMID:27789363; https://doi.org/10.1016/j.bbmt.2016.10.016
- Jasielec J, Saloura V, Godley LA. The mechanistic role of DNA methylation in myeloid leukemogenesis. Leukemia 2014; 28:1765-73; PMID:24913729; https://doi.org/10.1038/leu.2014.163
- Stresemann C, Bokelmann I, Mahlknecht U, Lyko F. Azacytidine causes complex DNA methylation responses in myeloid leukemia. Mol Cancer Ther 2008; 7:2998-3005; PMID:18790780; https://doi.org/10.1158/1535-7163.MCT-08-0411
- Sanchez-Abarca LI, Gutierrez-Cosio S, Santamaria C, Caballero-Velazquez T, Blanco B, Herrero-Sanchez C, García JL, Carrancio S, Hernández-Campo P, González FJ et al. Immunomodulatory effect of 5-azacytidine (5-azaC): Potential role in the transplantation setting. Blood 2010; 115:107-21; PMID:19887673; https://doi.org/10.1182/blood-2009-03-210393
- Choi J, Ritchey J, Prior JL, Holt M, Shannon WD, Deych E, Piwnica-Worms DR, DiPersio JF. In vivo administration of hypomethylating agents mitigate graft-versus-host disease without sacrificing graft-versus-leukemia. Blood 2010; 116:129-39; PMID:20424188; https://doi.org/10.1182/blood-2009-12-257253
- Goodyear OC, Dennis M, Jilani NY, Loke J, Siddique S, Ryan G, Nunnick J, Khanum R, Raghavan M, Cook M et al. Azacitidine augments expansion of regulatory T cells after allogeneic stem cell transplantation in patients with acute myeloid leukemia (AML). Blood 2012; 119:3361-9; PMID:22234690; https://doi.org/10.1182/blood-2011-09-377044
- Schroeder T, Frobel J, Cadeddu RP, Czibere A, Dienst A, Platzbecker U, Bug G, Uharek L, Fenk R, Germing U et al. Salvage therapy with azacitidine increases regulatory T cells in peripheral blood of patients with AML or MDS and early relapse after allogeneic blood stem cell transplantation. Leukemia 2013; 27:1910-3; PMID:23519388; https://doi.org/10.1038/leu.2013.64
- Polansky JK, Kretschmer K, Freyer J, Floess S, Garbe A, Baron U, Olek S, Hamann A, von Boehmer H, Huehn J. DNA methylation controls Foxp3 gene expression. Eur J Immunol 2008; 38:1654-63; PMID:18493985; https://doi.org/10.1002/eji.200838105
- Stubig T, Badbaran A, Luetkens T, Hildebrandt Y, Atanackovic D, Binder TM, Fehse B, Kröger N. 5-azacytidine promotes an inhibitory T-cell phenotype and impairs immune mediated antileukemic activity. Mediators Inflamm 2014; 2014:418292; PMID:24757283; https://doi.org/10.1155/2014/418292
- Fransolet G, Ehx G, Somja J, Delens L, Hannon M, Muller J, Dubois S, Drion P, Caers J, Humblet-Baron S et al. Azacytidine mitigates experimental sclerodermic chronic graft-versus-host disease. J Hematol Oncol 2016; 9:53; PMID:27377819; https://doi.org/10.1186/s13045-016-0281-2
- Costantini B, Kordasti SY, Kulasekararaj AG, Jiang J, Seidl T, Abellan PP, Mohamedali A, Thomas NS, Farzaneh F, Mufti GJ. The effects of 5-azacytidine on the function and number of regulatory T cells and T-effectors in myelodysplastic syndrome. Haematologica 2013; 98:1196-205; PMID:23242597; https://doi.org/10.3324/haematol.2012.074823
- Bruck F, Belle L, Lechanteur C, De LL, Hannon M, Dubois S, Castermans E, Humblet-Baron S, Rahmouni S, Beguin Y et al. Impact of bone marrow-derived mesenchymal stromal cells on experimental xenogeneic graft-versus-host disease. Cytotherapy 2013; 15:267-79; PMID:23265769; https://doi.org/10.1016/j.jcyt.2012.09.003
- King MA, Covassin L, Brehm MA, Racki W, Pearson T, Leif J, Laning J, Fodor W, Foreman O, Burzenski L et al. Human peripheral blood leucocyte non-obese diabetic-severe combined immunodeficiency interleukin-2 receptor gamma chain gene mouse model of xenogeneic graft-versus-host-like disease and the role of host major histocompatibility complex. Clin Exp Immunol 2009; 157:104-18; PMID:19659776; https://doi.org/10.1111/j.1365-2249.2009.03933.x
- Hippen KL, Merkel SC, Schirm DK, Sieben CM, Sumstad D, Kadidlo DM, McKenna DH, Bromberg JS, Levine BL, Riley JL et al. Massive ex vivo expansion of human natural regulatory T cells (T(regs)) with minimal loss of in vivo functional activity. Sci Transl Med 2011; 3:83ra41; PMID:21593401; https://doi.org/10.1126/scitranslmed.3001809
- Hippen KL, Bucher C, Schirm DK, Bearl AM, Brender T, Mink KA, Waggie KS, Peffault de Latour R, Janin A, Curtsinger JM et al. Blocking IL-21 signaling ameliorates xenogeneic GVHD induced by human lymphocytes. Blood 2012; 119:619-28; PMID:22077059; https://doi.org/10.1182/blood-2011-07-368027
- Kanakry CG, Ganguly S, Zahurak M, Bolanos-Meade J, Thoburn C, Perkins B, Fuchs EJ, Jones RJ, Hess AD, Luznik L. Aldehyde dehydrogenase expression drives human regulatory T cell resistance to posttransplantation cyclophosphamide. Sci Transl Med 2013; 5:211ra157; PMID:24225944; https://doi.org/10.1126/scitranslmed.3006960
- Janikashvili N, Trad M, Gautheron A, Samson M, Lamarthee B, Bonnefoy F, Lemaire-Ewing S, Ciudad M, Rekhviashvili K, Seaphanh F et al. Human monocyte-derived suppressor cells control graft-versus-host disease by inducing regulatory forkhead box protein 3-positive CD8 T lymphocytes. J Allergy Clin Immunol 2015; 135:1614-24; PMID:25630940; https://doi.org/10.1016/j.jaci.2014.12.1868
- Cuende J, Lienart S, Dedobbeleer O, van der Woning B, De Boeck G, Stockis J, Huygens C, Colau D, Somja J, Delvenne P et al. Monoclonal antibodies against GARP/TGF-beta1 complexes inhibit the immunosuppressive activity of human regulatory T cells in vivo. Sci Transl Med 2015; 7:284ra56; PMID:25904740; https://doi.org/10.1126/scitranslmed.aaa1983
- Ziegler SF. FOXP3: Of mice and men. Annu Rev Immunol 2006; 24:209-26; PMID:16551248; https://doi.org/10.1146/annurev.immunol.24.021605.090547
- Frikeche J, Clavert A, Delaunay J, Brissot E, Gregoire M, Gaugler B, Mohty M. Impact of the hypomethylating agent 5-azacytidine on dendritic cells function. Exp Hematol 2011; 39:1056-63; PMID:21856273; https://doi.org/10.1016/j.exphem.2011.08.004
- Bian G, Ding X, Leigh ND, Tang Y, Capitano ML, Qiu J, McCarthy PL, Liu H, Cao X. Granzyme B-mediated damage of CD8+ T cells impairs graft-versus-tumor effect. J Immunol 2013; 190:1341-50; PMID:23264653; https://doi.org/10.4049/jimmunol.1201554
- Barron L, Dooms H, Hoyer KK, Kuswanto W, Hofmann J, O'Gorman WE, Abbas AK. Cutting edge: Mechanisms of IL-2-dependent maintenance of functional regulatory T cells. J Immunol 2010; 185:6426-30; PMID:21037099; https://doi.org/10.4049/jimmunol.0903940
- Ehx G, Hannon M, Beguin Y, Humblet-Baron S, Baron F. Validation of a multicolor staining to monitor phosphoSTAT5 levels in regulatory T-cell subsets. Oncotarget 2015; 6:43255-66; PMID:26657728; https://doi.org/10.18632/oncotarget.6486
- Murayama A, Sakura K, Nakama M, Yasuzawa-Tanaka K, Fujita E, Tateishi Y, Wang Y, Ushijima T, Baba T, Shibuya K et al. A specific CpG site demethylation in the human interleukin 2 gene promoter is an epigenetic memory. EMBO J 2006; 25:1081-92; PMID:16498406; https://doi.org/10.1038/sj.emboj.7601012
- Sondergaard H, Kvist PH, Haase C. Human T cells depend on functional calcineurin, tumour necrosis factor-alpha and CD80/CD86 for expansion and activation in mice. Clin Exp Immunol 2013; 172:300-10; PMID:23574326; https://doi.org/10.1111/cei.12051
- Sripayap P, Nagai T, Uesawa M, Kobayashi H, Tsukahara T, Ohmine K, Muroi K, Ozawa K. Mechanisms of resistance to azacitidine in human leukemia cell lines. Exp Hematol 2014; 42:294-306 e2; PMID:24368162; https://doi.org/10.1016/j.exphem.2013.12.004
- Baron F, Petersdorf EW, Gooley T, Sandmaier BM, Malkki M, Chauncey TR, Maloney DG, Storb R. What is the role for donor natural killer cells after nonmyeloablative conditioning? Biology of Blood and Marrow Transplantation 2009; 15:580-8; PMID:19361750; https://doi.org/10.1016/j.bbmt.2009.01.018
- Meinhardt K, Kroeger I, Bauer R, Ganss F, Ovsiy I, Rothamer J, Büttner M, Atreya I, Waldner M, Bittrich M et al. Identification and characterization of the specific murine NK cell subset supporting graft-versus-leukemia- and reducing graft-versus-host-effects. Oncoimmunology 2015; 4:e981483; PMID:25949862; https://doi.org/10.4161/2162402X.2014.981483
- Betts BC, Veerapathran A, Pidala J, Yang H, Horna P, Walton K, Cubitt CL, Gunawan S, Lawrence HR, Lawrence NJ et al. Targeting aurora kinase A and JAK2 prevents GVHD while maintaining treg and antitumor CTL function. Sci Transl Med 2017; 9; PMID:28077684; https://doi.org/10.1126/scitranslmed.aai8269
- Baron F, Labopin M, Blaise D, Lopez-Corral L, Vigouroux S, Craddock C, Attal M, Jindra P, Goker H, Socié G et al. Impact of in vivo T-cell depletion on outcome of AML patients in first CR given peripheral blood stem cells and reduced-intensity conditioning allo-SCT from a HLA-identical sibling donor: A report from the acute leukemia working party of the European group for blood and marrow transplantation. Bone Marrow Transplant 2014; 49:389-96; PMID:24419525; https://doi.org/10.1038/bmt.2013.204
- Bontkes HJ, Ruben JM, Alhan C, Westers TM, Ossenkoppele GJ, van de Loosdrecht AA. Azacitidine differentially affects CD4(pos) T-cell polarization in vitro and in vivo in high risk myelodysplastic syndromes. Leuk Res 2012; 36:921-30; PMID:22503132; https://doi.org/10.1016/j.leukres.2012.03.026
- Ratajczak P, Janin A, Peffault de Latour R, Leboeuf C, Desveaux A, Keyvanfar K, Robin M, Clave E, Douay C, Quinquenel A et al. Th17/Treg ratio in human graft-versus-host disease. Blood 2010; 116:1165-71; PMID:20484086; https://doi.org/10.1182/blood-2009-12-255810
- Malek TR, Bayer AL. Tolerance, not immunity, crucially depends on IL-2. Nat Rev Immunol 2004; 4:665-74; PMID:15343366; https://doi.org/10.1038/nri1435
- Pierson W, Cauwe B, Policheni A, Schlenner SM, Franckaert D, Berges J, Humblet-Baron S, Schönefeldt S, Herold MJ, Hildeman D et al. Antiapoptotic Mcl-1 is critical for the survival and niche-filling capacity of Foxp3(+) regulatory T cells. Nat Immunol 2013; 14:959-65; PMID:23852275; https://doi.org/10.1038/ni.2649
- Humblet-Baron S, Franckaert D, Dooley J, Bornschein S, Cauwe B, Schonefeldt S, Bossuyt X, Matthys P, Baron F, Wouters C et al. IL-2 consumption by highly activated CD8 T cells induces regulatory T-cell dysfunction in patients with hemophagocytic lymphohistiocytosis. J Allergy Clin Immunol 2016; 138:200-9; PMID:26947179; https://doi.org/10.1016/j.jaci.2015.12.1314
- Zhao XY, Zhao XS, Wang YT, Chen YH, Xu LP, Zhang XH, Han W, Chen H, Wang Y, Yan CH et al. Prophylactic use of low-dose interleukin-2 and the clinical outcomes of hematopoietic stem cell transplantation: A randomized study. Oncoimmunology 2016; 5:e1250992; PMID:28123892; https://doi.org/10.1080/2162402X.2016.1250992
- Ballas ZK. The use of 5-azacytidine to establish constitutive interleukin 2-producing clones of the EL4 thymoma. J Immunol 1984; 133:7-9; PMID:6202793.
- Bruniquel D, Schwartz RH. Selective, stable demethylation of the interleukin-2 gene enhances transcription by an active process. Nat Immunol 2003; 4:235-40; PMID:12548284; https://doi.org/10.1038/ni887
- Dong S, Maiella S, Xhaard A, Pang Y, Wenandy L, Larghero J, Becavin C, Benecke A, Bianchi E, Socié G et al. Multiparameter single-cell profiling of human CD4+FOXP3+ regulatory T-cell populations in homeostatic conditions and during graft-versus-host disease. Blood 2013; 122:1802-12; PMID:23818545; https://doi.org/10.1182/blood-2013-02-482539
- Sim GC, Martin-Orozco N, Jin L, Yang Y, Wu S, Washington E, Sanders D, Lacey C, Wang Y, Vence L et al. IL-2 therapy promotes suppressive ICOS+ Treg expansion in melanoma patients. J Clin Invest 2014; 124:99-110; PMID:24292706; https://doi.org/10.1172/JCI46266
- Craddock C, Jilani N, Siddique S, Yap C, Khan J, Nagra S, Ward J, Ferguson P, Hazlewood P, Buka R. et al. Tolerability and clinical activity of post-transplantation azacitidine in patients allografted for acute myeloid leukemia treated on the RICAZA trial. Biol Blood Marrow Transplant 2015; 22:385-90; PMID:26363443; https://doi.org/10.1016/j.bbmt.2015.09.004
- Baron F, Maris MB, Sandmaier BM, Storer BE, Sorror M, Diaconescu R, Woolfrey AE, Chauncey TR, Flowers ME, Mielcarek M et al. Graft-versus-tumor effects after allogeneic hematopoietic cell transplantation with nonmyeloablative conditioning. J Clin Oncol 2005; 23:1993-2003; PMID:15774790; https://doi.org/10.1200/JCO.2005.08.136
- Storb R, Gyurkocza B, Storer BE, Sorror ML, Blume K, Niederwieser D, Chauncey TR, Pulsipher MA, Petersen FB, Sahebi F et al. Graft-versus-host disease and graft-versus-tumor effects after allogeneic hematopoietic cell transplantation. J Clin Oncol 2013; 31:1530-8; PMID:23478054; https://doi.org/10.1200/JCO.2012.45.0247
- Shultz LD, Saito Y, Najima Y, Tanaka S, Ochi T, Tomizawa M, Doi T, Sone A, Suzuki N, Fujiwara H et al. Generation of functional human T-cell subsets with HLA-restricted immune responses in HLA class I expressing NOD/SCID/IL2r gamma(null) humanized mice. Proc Natl Acad Sci USA 2010; 107:13022-7; PMID:20615947; https://doi.org/10.1073/pnas.1000475107