Abstract
Cynomolgus macaques were vaccinated by intramuscular electroporation with DNA plasmids expressing codon-optimized glycoprotein (GP) genes of Ebola virus (EBOV) or Marburg virus (MARV) or a combination of codon-optimized GP DNA vaccines for EBOV, MARV, Sudan virus and Ravn virus. When measured by ELISA, the individual vaccines elicited slightly higher IgG responses to EBOV or MARV than did the combination vaccines. No significant differences in immune responses of macaques given the individual or combination vaccines were measured by pseudovirion neutralization or IFN-γ ELISpot assays. Both the MARV and mixed vaccines were able to protect macaques from lethal MARV challenge (5/6 vs. 6/6). In contrast, a greater proportion of macaques vaccinated with the EBOV vaccine survived lethal EBOV challenge in comparison to those that received the mixed vaccine (5/6 vs. 1/6). EBOV challenge survivors had significantly higher pre-challenge neutralizing antibody titers than those that succumbed.
Introduction
Members of the family Filoviridae are enveloped RNA viruses with nonsegmented, negative-sense genomes. They are classified into 3 serologically distinct genera: Marburgvirus, Ebolavirus, and Cuevavirus.Citation1 The Marburgvirus genus currently includes a single viral species, Marburg marburgvirus, and 2 defined viruses, Marburg virus (MARV) and Ravn virus (RAVV).Citation1 The Ebolavirus genus includes 5 serologically distinct viral species: Zaire ebolavirus (EBOV), Sudan ebolavirus (SUDV), Reston ebolavirus (RESTV), Taï Forest virus (TAFV), and Bundibugyo ebolavirus (BDBV). The Cuevavirus genus has one viral species, Lloviu cuevavirus, and one defined virus, Lloviu virus (LLOV).Citation1 Phylogenetic analysis of full-length genomic nucleotide sequences of viruses in each genus revealed that they have roughly similar identity to one another; i.e., MARV:EBOV (≥50%); MARV:LLOV (˜56%); EBOV:LLOV (˜51%).Citation1 MARV and all of the viruses in the Ebolavirus genus except Reston virus have caused severe hemorrhagic fevers in humans characterized by fever, anorexia, diarrhea, hemorrhaging, and a classic petechial rash, which typically disseminates from the head to the entire body as infection progresses.Citation2 Epidemiological studies have not been able to clearly identify the natural reservoir(s) of filoviruses, but both MARV and EBOV have been detected in fruit bats in Africa, and MARV has been isolated from Rousettus bats in Uganda.Citation3,4
The approximately 19-kb filovirus genome encodes 7 viral structural proteins with a gene order of NP-VP35-VP40-GP-VP30-VP24-L.Citation5,6 For EBOV, the primary GP gene product of complementary-sense mRNA is a soluble form of GP (sGP), which is not a structural protein. The structural protein GP is generated through transcriptional editing, which causes a shift in the gene's reading frame.Citation7,8 Mature GP is a highly glycosylated type 1 membrane protein. It is generated by posttranslational proteolytic cleavage of a precursor by a cellular furin-like enzyme.Citation9 This cleavage results in a large amino-terminal fragment (GP1) and a smaller C-terminal fragment (GP2) that reassociate by disulfide bonding. Trimers of GP1,2 form the virion spikes, thus GP is the main target of antibody responses.Citation2 Filoviruses are listed as Category A priority pathogens by the National Institutes of Allergy and Infectious Diseases (NIAID), indicating that they pose the highest risk to national security and public health. In addition, EBOV and MARV are categorized as Tier 1 Biological Select Agents by the Centers for Disease Control and Prevention, because of the risk of their deliberate misuse resulting in significant potential impact to public health and safety. To date, there are no filovirus vaccines or therapeutics licensed by the Food and Drug Administration, although several candidate vaccines have shown promise in animal models.Citation10-16 However, vaccine efforts are still hindered by a poor understanding of the correlates of protective immunity. In general, both strong humoral and cell-mediated immune responses have been shown to be important for survival from filovirus infections.Citation17-21
In earlier studies, we showed that DNA vaccines expressing the GP genes of MARV delivered by gene gun elicited partially protective immunity in NHP.Citation22 Similarly, we showed that an EBOV GP DNA vaccine delivered to guinea pigs by gene gun provided partial protection against EBOV challenge.Citation23 Toward the goal of improving the protective efficacy of these DNA vaccines, we designed gene-optimized DNA vaccine constructs and used a more potent delivery method, intramuscular electroporation (IM-EP). In addition, because a major advantage of DNA vaccines over other types of vaccines is the ability to mix together several plasmids to generate combination vaccines, we also sought to determine if a mixture of filovirus DNA vaccines could elicit immunity against more than one filovirus. In a preliminary study in mice, we showed that IM-EP delivery of an optimized EBOV GP DNA vaccine, or a mixture of optimized EBOV, SUDV, MARV, and RAVV GP DNA vaccines, protected mice from challenge with mouse-adapted EBOV.Citation24 Similarly, DNA vaccines expressing the optimized GP genes of MARV GP, RAVV GP, or a combination of all 4 DNA vaccines given by IM-EP protected mice from challenge with mouse-adapted RAVV.Citation24 Here we report the evaluation of these same DNA vaccines in cynomolgus macaques, which is the most relevant model available for disease in humans.
Results
Total IgG antibody responses of DNA-vaccinated cynomolgus macaques
In two separate studies, groups of 6 cynomolgus macaques were vaccinated by IM-EP with 500 μg of the individual MARV-GP or EBOV-GP DNA vaccines (MARV study or EBOV study, respectively) or with a combination of 500 μg of each (2 mg total) of the MARV-GP, RAVV-GP, EBOV-GP, and SUDV-GP DNA vaccines (both studies). For each study, an additional control macaque was vaccinated with the plasmid vector without an insert (pWRG7077). Each macaque was vaccinated 3 times at 28-day intervals with a total of 500 μg of the individual vaccines, or with a total of 2 mg of the DNA combination. To assess the development of antibody responses to the vaccines, we tested serum samples collected at days 0, 28, 56 and 84 by ELISA against MARV, EBOV () and SUDV (data not shown) expression products that represented full-length GP minus the transmembrane region of GP2 (ΔTM) or full-length GP minus both the transmembrane region and the mucin domain (ΔMuc). The use of both types of antigens allowed us to indirectly compare differences in antibody responses to the poorly conserved, but functionally important, mucin domain in samples from macaques vaccinated with single versus combination vaccines.
Figure 1. Comparison of GP-specific IgG responses of macaques vaccinated within individual or combination DNA vaccine over time. Groups of 6 cynomolgus macaques were vaccinated 3 times at 4 week intervals with individual optimized MARV (A-B) or EBOV (C-D) DNA vaccines or with a combination of all 4 filovirus vaccines (E-L) as part of 2 separate challenge studies (MARV study, EBOV study). Sera were collected from macaques prior to each vaccination (days 0, 28, 56) and 28 d after the final vaccination (day 84). IgG antibodies were assayed by indirect ELISA using HEK 293T-expressed and purified, soluble antigen representing full length MARV or EBOV GP minus the transmembrane regions (MARV GPΔTM antigen: A, E, I; EBOV GPΔTM antigen: C, G, K) or minus both the transmembrane region and the mucin domain (MARV GPΔMuc antigen: B, F, J; EBOV GPΔMuc antigen: D, H, L). Sera from single agent vaccine groups were tested against the homologous GP antigens only (A-D). To determine the antibody units/ml (Ab U/ml), each serum sample was compared to a standard reference curve of pooled cynomolgus macaque immune sera. The Ab U/ml for each dilution of the test NHP sera were interpolated from this standard curve. Black horizontal bars indicate the mean titers for each group. To evaluate differences in ELISA titers within vaccine groups over time, a repeated measures 2-way ANOVA with Tukey's multiple comparison test was used. Significant differences between study days are indicated (*) and P values for comparisons between days are: 0 vs 56 P < 0.0001; 56 vs 84, P < 0.0001 (A). 0 vs 28 P = 0.0086; 28 vs 56 P = 0.0056; 56 vs 84 P < 0.0001 (B). 0 vs 56 P = 0.0007 (C). 0 vs 56 P = 0.0015; 56 vs 84 P = 0.0048 (D). 0 vs 84 P = 0.0030 (E). 0 vs 56 P = 0.0140 (F). 0 vs 56 P = 0.0123; 56 vs 84 P = 0.0009 (G). 0 vs 56 P = 0.0048; 56 vs 84 P < 0.0001 (H). 0 vs 56 P < 0.0001 (I). 0 vs 56 P = 0.0003 (J). 0 vs 56 P = 0.0484 (K). 0 vs 56 P = 0.0167 (L).
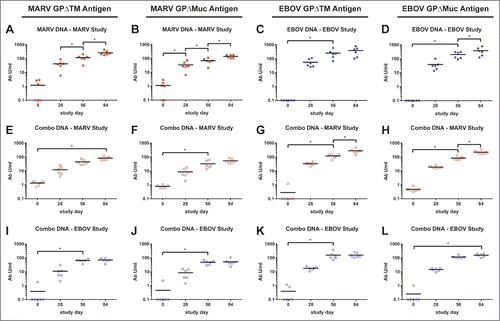
Macaques that received the MARV-GP vaccine developed IgG antibody responses against MARV GPΔTM that were significantly elevated above background after 2 vaccinations and against MARV GPΔMuc that were significantly elevated above background after a single vaccination (). These IgG responses significantly increased against both antigens after additional vaccinations. For the EBOV-GP vaccine, 2 vaccinations resulted in significant IgG responses against both antigens. A third vaccination significantly boosted the response when assayed with the EBOV GPΔMuc antigen, but not the EBOV GPΔTM ELISA antigen (). In both the EBOV and MARV studies, the combination vaccines elicited significant IgG titers against EBOV and MARV after 2 or 3 vaccinations when assayed with either antigen (). In both the MARV and EBOV studies, macaques vaccinated twice with the combination of vaccines developed significant antibody responses to EBOV GP, MARV GP () and SUDV GP (data not shown) after 2 or 3 vaccinations. Notably, in both the MARV and EBOV studies, the mean GP-specific antibody concentrations of macaques in the single vaccine groups were significantly higher than those in the combination groups after the third vaccination (day 84; MARV GPΔTM, 259.8 vs 82.7 Ab U/ml, P < 0.0001; MARV GPΔMuc, 142.4 vs 53.7 Ab U/ml, P < 0.0001; EBOV GPΔTM, 381.4 vs 159.6 Ab U/ml, P = 0.0088; EBOV GPΔMuc, 380.0 vs 155.3 Ab U/ml, P = 0.0006) (). These results indicate that significantly lower antibody levels were elicited to MARV and EBOV when the DNA vaccines were given in combination as compared to when they were given alone, but that this did not reflect a bias toward mucin domain-specific IgG responses.
Figure 2. Comparison of GP-specific IgG responses of macaques between vaccine groups over time. These are the same indirect ELISA data shown in , but with individual and combination vaccine groups for within the (A-B) MARV and (C-D) EBOV studies plotted together. Black horizontal bars indicate the mean titers for each group. To evaluate differences in ELISA titers between vaccine groups over time, a repeated measures 2-way ANOVA with Tukey's multiple comparison test was used. Significant differences between vaccine groups are indicated (*) and P values for comparisons between days are: (A) day 56, P = 0.0170; day 84, P < 0.0001. (B) day 56, P = 0.0118; day 84, P < 0.0001. (C) day 84, P = 0.0088. (D) day 84, P = 0.0006.
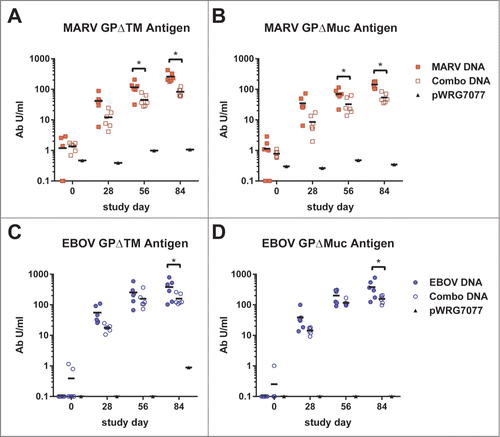
In support of this finding, when we performed ELISA on overlapping peptides representing the complete GP of EBOV or MARV using methods identical to those we reported earlier for examining the contribution of GP glycosylation to protection in mice 25, we saw no obvious or consistent differences in antibody responses to this or any other region of GP1,2 of EBOV or MARV or to any other region, including to 5 peptides specific for sGP of EBOV, among macaques that survived EBOV or MARV challenges as compared to those that succumbed (data not shown).
Neutralizing antibody responses of DNA-vaccinated cynomolgus macaques
We measured neutralizing antibody responses in macaque sera collected after the third vaccination (day 84) using pseudovirions (PsVs) based on a VSVΔG*rLuc core which displayed GPs of the individual filoviruses. Neutralizing antibody responses were expressed as the log-transformed titer that resulted in reduction of the PsV infection luminescence signal by 50 percent relative to controls without antisera (PsVNA50). In the MARV study, macaques vaccinated with MARV-GP developed neutralizing antibodies to MARV (). There was no cross-neutralization of RAVV or SUDV, although one sample did cross-neutralize EBOV. All of the macaques in the combination group developed PsV-neutralizing antibodies to all 4 filoviruses, and there was no significant difference in the mean log10 PsVNA50 titers against MARV PsVs between the MARV-GP and combination vaccine groups. In the EBOV study, all macaques vaccinated with EBOV-GP vaccine developed pre-challenge neutralizing antibodies to EBOV PsVs (). No cross-reactive antibodies to MARV or RAVV were detected, while 1 of 6 macaques developed a PsV-neutralizing response to SUDV. All macaques in the combination vaccine group developed neutralizing antibodies toward all 4 filovirus PsVs, and there was no significant difference in the mean log10 PsVNA50 titers against EBOV PsVs between the EBOV-GP and combination vaccine groups. Between the 2 study groups, only the SUDV-specific mean titers were significantly different, with the MARV study group's mean being higher (log10 PsVNA50 titer 2.850 vs 2.654, P = 0.0093).
Figure 3. Neutralizing antibody responses elicited by filovirus DNA vaccines in macaques. A dilution series of heat-inactivated sera (day 84) supplemented with human complement was incubated with VSVΔG*rLuc pseudovirions decorated with the glycoproteins of each filovirus and then used to infect Vero cells. At 18–24 hrs post-infection, the cells were lysed, luciferase substrate was added, and the resulting signal was measured using a luminometer. The data were fit to a sigmoidal dose/response curve with variable slope to determine the serum titer required to neutralize infection by 50% relative to untreated controls (PsVNA50), and the limit of quantitation for this assay was 1.3 logs. The log10 PsVNA50 titers are shown for the MARV (A) and EBOV (B) studies. There was no significant difference (ns) in the mean log10 PsVNA50 titers against MARV PsVs between the MARV-GP and combination vaccine groups as determined by an unpaired 2 tailed t-test, P = 0.0702. Similarly, there was no significant difference in the mean log10 PsVNA50 titers against EBOV PsVs between the EBOV-GP and combination vaccine groups as determined by an unpaired 2 tailed t-test, P = 0.3210.
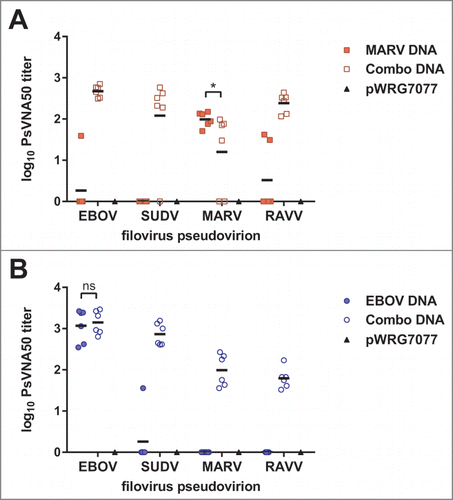
Cellular immune responses of DNA-vaccinated cynomolgus macaques
Cryopreserved PBMCs collected after the second vaccination (day 56) were recovered and restimulated using pools of overlapping peptides spanning the complete amino acid sequences of either MARV GP or EBOV GP, and then filovirus-specific T cell responses were measured by interferon gamma (IFN-γ) ELISpot. In the MARV study, we were able to detect T cell responses in macaques vaccinated with either MARV-GP or the combination vaccine (). As with the neutralizing antibody antibody responses, there was no significant difference in the mean spot-forming units of PBMCs from macaques vaccinated with either the MARV-GP (624.3) or combination (544.5) vaccines (unpaired 2 tailed t-test, P = 0.8263). Similarly, in the EBOV study, we were able to detect T cell responses in macaques vaccinated with either EBOV-GP or the combination vaccine (). Again, there was no significant difference in the mean number of spot forming units of PBMCs from macaques vaccinated with either the EBOV-GP (283.5) or combination (262.0) vaccines (unpaired 2 tailed t-test, P = 0.8171).
Figure 4. IFN-γ producing cellular responses elicted by filovirus DNA vaccines in macaques. Cryopreserved peripheral blood mononuclear cells collected on day 56 were revived and stimulated with (A) MARV- or (B) EBOV-derived peptides spanning their respective GP proteins for 48 hrs. Peptide-specific memory T cell responses were assayed by IFN-γ ELISpot. Black horizontal bars indicate the means for each group. There was no significant difference (ns) between single and combination vaccine groups in either study unpaired 2 tailed t-test (MARV study, P = 0.8263, EBOV study, P = 0.8171)
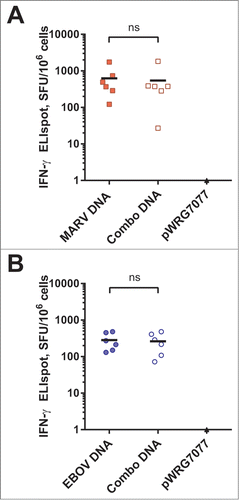
We also attempted to perform intracellular cytokine staining of banked, restimulated PBMCs, by staining for CD3, CD4, CD8, TNF-α, IL2 and IFN-γ. We were unsuccessful in obtaining meaningful results, in that the percentages of positive cells for production of the cytokines were not significantly higher than background (data not shown). We were constrained by the number of events we could collect as we had very few PBMCs banked, thus we were unable to collect sufficient events to provide adequate sensitivity in the assays.
Protective efficacy of the filovirus DNA vaccines
At 56 d following the third vaccination (day 112), macaques in each study were challenged by IM injection of a lethal dose (1 × 103 plaque forming units) of MARV or EBOV, respectively. All macaques were observed daily for fever, petechial rash, gastrointestinal distress, and changes in behavior (Table S1). Clinical observations were consistent with EBOV- and MARV-induced disease in cynomolgus macaques, and the severity of symptoms generally correlated with survival outcome (). All surviving macaques were euthanized at the conclusion of the study, which was 28 d post-challenge.
In the MARV study, 5 macaques (83%) that received the MARV-GP vaccine survived lethal MARV challenge, as did all macaques that received the combination vaccine (). The macaque vaccinated with the control plasmid and a single MARV-GP vaccinated macaque met the clinical severity threshold for euthanasia on days 9 and 10 post-challenge, respectively. Inclusion of historical survival data from 3 macaques, which all succumbed to MARV infection after IM challenge, yielded a mean time to death of 9.67 d post-challenge. Using these data, we generated Kaplan-Meier survival curves and evaluated them using a log-rank test for homogeneity, which indicated that there was a significant difference among the 3 treatment groups (P = 0.0007). A Fisher's exact test with multiple comparison adjustment based on permutation was used to confirm the increased survival of MARV-GP and combination-vaccinated macaques in comparison to control macaques (P = 0.0212 and 0.0026, respectively). There was no significant difference in mean time to death among the 3 vaccine groups.
Figure 5. Protective efficacy of filovirus DNA vaccines and clinical observations in MARV-challenged macaques. (A) Kaplan-Meier survival curves were generated for the MARV vaccination study. A Fisher's exact test with multiple comparison adjustment based on permutation was used to evaluate pairwise differences (P < 0.05) relative to the control group (*). Historical controls (†) that received the same MARV challenge dose and route of administration were included for statistical analyses (n = 3). (B-D) After MARV challenge, macaques were scored twice daily for clinical signs typical of hemorrhagic fever disease such as weight loss, changes in body temperature, petechial rash, ischuria, gastrointestinal distress, labored breathing, lack of responsiveness, recumbency, edema, and anorexia. Personnel performing observations were blinded to the treatment groups.
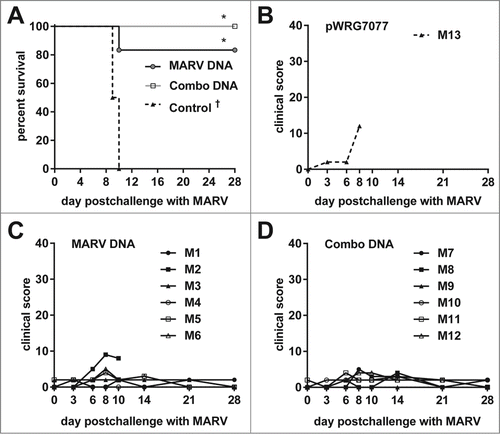
In the EBOV study, the individual vaccine yielded the same results seen with MARV vaccine in that 5 of 6 macaques that received the EBOV-GP vaccine survived lethal EBOV challenge with the non-survivor meeting euthanasia criteria on day 6 postchallenge (). One other macaque developed moderate signs of disease between days 6–10, but had recovered by day 14 postchallenge. However, in contrast to macaques in the MARV study that received the combination vaccine, in which all showed few to no clinical signs of disease, only one animal that received the combination vaccine survived EBOV challenge, with death or euthanasia occurring between days 3–8 postchallenge (mean time to death, 7 days). The macaque vaccinated with pWRG7077 met euthanasia criteria on day 7. Inclusion of historical data from 15 macaques that all succumbed to EBOV after IM challenge yielded a mean time to death of 7.56 d postchallenge. Using these data, we generated Kaplan-Meier survival curves and evaluated them using a log-rank test for homogeneity, which indicated that there was a significant difference among the 3 treatment groups (P = 0.0074). A Fisher's exact test with multiple comparison adjustment based on permutation was used to confirm the increased survival of EBOV-GP vaccinated macaques in comparison to macaques receiving the combination vaccine (P = 0.0455) and to control macaques (P = 0.0002). There was no significant survival advantage conferred by the combination vaccine in comparison to control macaques (P = 0.3414). There was also no significant difference in mean time to death among the 3 vaccine groups.
Figure 6. Protective efficacy of filovirus DNA vaccines and clinical observations in EBOV-challenged macaques. (A) Kaplan-Meier survival curves were generated for the EBOV vaccination study. A Fisher's exact test with multiple comparison adjustment based on permutation was used to evaluate pairwise differences (P < 0.05) relative to the control group (*) or to both the control and combination groups (∴). Historical controls (†) that received the same EBOV challenge dose and route of administration were included for statistical analyses (n = 15). (B-D) After EBOV challenge, macaques were scored twice daily for clinical signs typical of hemorrhagic fever disease such as weight loss, changes in body temperature, petechial rash, ischuria, gastrointestinal distress, labored breathing, lack of responsiveness, recumbency, edema, and anorexia. Personnel performing observations were blinded to the treatment.
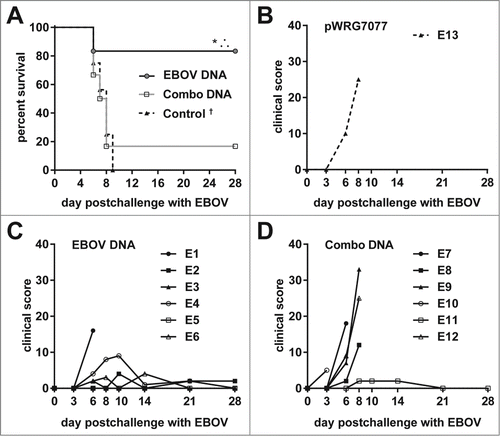
Serum viremia, clinical chemistries and pathological findings in cynomolgus macaques after EBOV or MARV challenge
We measured serum viremia in samples collected on days 0, 3, 6, 8, 10, 14, 21 and 28 after challenge by standard filovirus plaque assay, and the data were analyzed using a mixed model repeated measures statistical framework. In the MARV study, the control macaque (M13) and the macaque in the combination vaccine group that was euthanized due to disease severity (M2), both displayed a high viremia prior to death (, Table S1). One additional macaque in the GP-MARV group (M3) and 3 of the macaques in the combination group (M7, M8, M9) had a transient viremia on one of the assay dates. Day 8 was the only time where there was a significant difference in the mean log10 viremia between vaccine groups, with the MARV GP group being higher (P = 0.0023).
Figure 7. Viremia, AST levels, and platelet counts of MARV-challenged macaques. Whole blood was collected from nonhuman primates at the indicated time points post challenge with MARV. The measured parameters for each animal are shown grouped by vaccine that was administered: (A, D, G) EBOV-GP; (B, E, H) Combination; (C, F, I) pWRG7077. Serum viremia was determined by standard filovirus plaque assays.
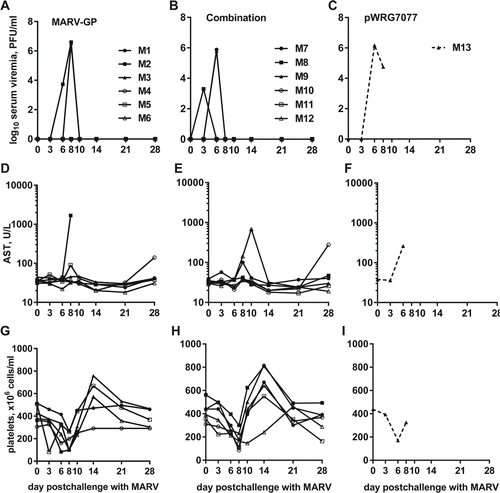
In the EBOV study, the control and all of the macaques vaccinated with the combination vaccine that succumbed to EBOV infection or were euthanized because of disease severity displayed viremia at one or more days after challenge (, Table S1). The one surviving macaque in the combination group (E11) had a transient viremia that peaked on day 3 post-challenge. The single macaque that was euthanized due to clinical severity in the group vaccinated with EBOV-GP (E1) had no detectable viremia; however, 2 of the surviving macaques (E4, E5) in the EBOV-GP vaccine group had a transient viremia on day 8 post-challenge. On day 6 post-challenge, the mean log10 viremia titer was greater for the combination vaccine group compared to the EBOV-GP group (P = 0.0002).
Figure 8. Viremia, AST levels, and platelet counts of EBOV-challenged macaques. Whole blood was collected from nonhuman primates at the indicated time points post challenge with EBOV. The measured parameters for each animal are shown grouped by vaccine that was administered: (A, D, G) EBOV-GP; (B, E, H) Combination; (C, F, I) pWRG7077. Serum viremia was determined by standard filovirus plaque assays.
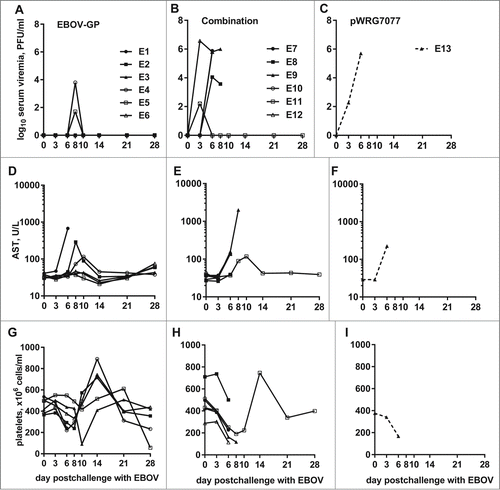
Abnormal liver function and decreased platelets are hallmarks of filovirus infections.Citation25 To assess liver function, we measured aspartate aminotransferase (AST) levels in serum samples collected on days 0, 3, 6, 8, 10, 14, 21 and 28 after challenge. In the MARV study, AST levels in the control macaque and the macaque vaccinated with MARV-GP that was euthanized due to disease severity peaked prior to death (). Likewise, in the EBOV study, the control macaque and most (5/6) of the vaccinated macaques that succumbed to EBOV infection had AST levels that peaked prior to death (). In both studies, all other macaques either had no marked changes in serum AST levels or had increased values that returned to baseline by the end of the study. In both studies, platelet numbers followed a biphasic pattern in response to infection with an initial decrease between days 3–10 followed by a sharp increase between days 14–21 (). In general, platelet numbers in non-surviving macaques did not rebound following the initial depletion. Both the AST levels and platelet numbers observed in these studies are consistent with typical filovirus infections in cynomolgus macaques.
In both studies, complete necropsies and histological analyses were performed on all macaques and associated specimens. Gross abnormalities and histologic lesions in non-surviving macaques were consistent with those previously described in EBOV- or MARV-infected cynomolgus macaques. In general, survivors merely displayed follicular lymphoid hyperplasia indicative of chronic antigen stimulation caused either by vaccination, filovirus challenge, or an unrelated antigen (Supplementary Data).
Correlation of antibody titers and survival from EBOV challenge
Given the differences in survival outcomes between the MARV and EBOV challenge studies, we reexamined the PsV-neutralizing antibody data in the context of survival (). Although we did not detect statistical differences in mean neutralizing antibody responses to EBOV between the single and combination groups (), when we compared pre-challenge PsV-neutralizing antibody titers of macaques that survived from both groups to those that succumbed in both groups, we found that survivors had a statistically higher mean neutralizing antibody titer (log10 PsVNA50 titer 2.817 vs 2.548, P = 0.0331) (). Similarly, surviving NHPs had higher EBOV GP-specific IgG titer as compared to nonsurvivors (402.6 vs 138.4 Ab U/ml, P = 0.0010) (). Because all but one macaque survived the MARV challenge, we could not do similar statistical comparisons for MARV.
Figure 9. Survival from EBOV challenge correlates with increased antibody responses in cynomolgous macaques. These are the same PsVNA data shown in , but with the data regrouped by individual NHP in the (A) MARV or (B) EBOV study groups. Animals which succumbed to challenge are highlighted in gray (“Nonsurvivor”). (C) EBOV PsVNA data for the EBOV study group were additionally regrouped by survival. (D) These are the same EBOV GPΔTM-specific ELISA data shown in regrouped by survival. Black horizontal bars indicate the mean of each group. There was a significant difference in the mean log10 PsVNA50 titers against EBOV PsVs between the survivor and nonsurvivor groups as determined by an unpaired 2 tailed t-test, p = 0.0331. There was also a significant difference in the mean ELISA titers between these groups as determined by a repeated measures 2-way ANOVA with Sidak's multiple comparison test.
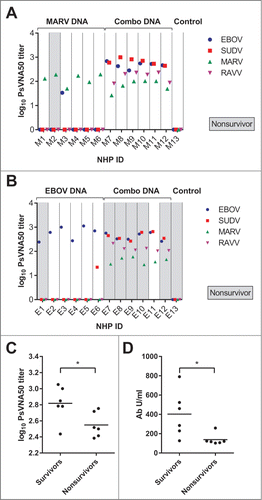
Discussion
DNA vaccines are safe, easy to design and can be made quickly in response to an epidemic, such as the one ongoing in Africa, to target the vaccine to the specific strain of circulating virus. DNA vaccines have advantages over other filovirus vaccine candidates in that they can be quickly altered, readily manufactured, and are more thermostable than competitive platforms. Thermal stability may be essential for a successful filovirus vaccine as outbreaks typically occur sporadically and in remote locations that lack refrigeration. Further, DNA vaccines lack pre-existing vector immunity and because of their increased safety profile can be administered to immunocompromised individuals. The latter being extremely important as HIV is endemic in countries where filovirus outbreaks historically occur.
In a previous study, we showed that a DNA vaccine expressing the GP gene of MARV delivered by gene gun elicited protective immunity to MARV challenge in 4 of 6 cynomolgus macaques.Citation22 We also tested a similar EBOV DNA vaccine, using a prime-boost regimen with a DNA prime and a baculovirus-expressed EBOV GP boost. Although this approach was successful for MARV in guinea pigs and nonhuman primates (NHP), it was not successful for conferring protective immunity against EBOV.Citation23,26 Here, we report the results of studies aimed at improving upon those findings by using both a more effective delivery method as well as codon-optimized DNA vaccine constructs.
To improve DNA vaccine delivery, we used IM-EP, which we have found to be superior in terms of the magnitude and rate of the immune response to all other methods we have tested to date for eliciting strong humoral and cell-mediated immune responses in a variety of animal models.Citation24,27-29 We also have found IM-EP delivery to be safe for humans in Phase 1 clinical studies of DNA vaccines for 2 hantavirusesCitation30 and for Venezuelan equine encephalitis virus (VEEV) (unpublished data). In the hantavirus clinical study, we demonstrated improved immunogenicity as compared to gene gun delivery of these same vaccines.Citation30,31 The immunogenicity assessments of sera from subjects in the VEEV study are in progress.
In addition to our primary goal of testing these methods for improving the efficacy of the DNA vaccines for EBOV and MARV in a relevant animal model for human disease, we also sought to determine if a mixed vaccine formulation could induce protective immunity against both EBOV and MARV. The mixture included the GP genes of the 2 member viruses in the genus Marburgvirus, MARV and RAVV, which have an amino acid sequence identity of 78.3% and 2 virus species in the genus Ebolavirus, EBOV and SUDV, which have a sequence identity of 56.6%. In contrast, amino acid sequence identity between the genera was only 27–28%.
To enhance the immunogenicity of the filovirus DNA vaccine constructs, we optimized the GP genes to reflect the codon bias of Homo sapiens and to remove gene elements known to impact mRNA stability or expression. For both the hantavirus and VEEV DNA vaccines, gene optimization was found to increase expression and to improve antibody responses in animals and humans.Citation28,30-35 For the 2 hantavirus vaccines, optimization also mitigated immune interference, which we observed when using non-optimized constructs.Citation27,29 Although we did not have non-optimized constructs for the SUDV or RAVV DNA vaccines, we were able to show strong expression in cell culture with all 4 optimized constructs and we found improved gene expression for the EBOV optimized construct as compared to the non-optimized gene.Citation24
The outcome of our challenge studies indicated that the optimized MARV and EBOV constructs delivered by IM-EP offered better protective immunity than we observed previously. This was most notable for EBOV, in that all but one macaque vaccinated with the EBOV DNA vaccine survived challenge as compared to none previously. Also, the EBOV stock used here is currently designated by the Filovirus Animal Non-Clinical Group (FANG) as the challenge material that will be used for FDA Animal Rule studies.
An unexpected outcome of the challenge studies was the ability of the combination vaccine to completely protect against MARV challenge but to have decreased protection against EBOV as compared to the monovalent EBOV vaccine. For both the EBOV and MARV studies, there was a trend toward higher IgG antibody levels measured for the macaques in the individual vaccine groups vs. those in the combination groups. We considered whether the poorer protection from EBOV challenge in macaques given the combination vaccines as compared to only the EBOV vaccine might relate to a skewing of the response toward or against the GP mucin domains. Although correlates of protective immunity with regard to responses to the mucin domain are poorly defined, it has been shown that removal of the mucin domain reveals additional neutralizing epitopesCitation36,37 and that antibodies to the mucin domain can be protective in mice.Citation38 Of interest, the genome sequences reported for the EBOV strain causing the recent epidemic of Ebola hemorrhagic fever in West Africa were reported to be ˜97% homologous with earlier strains from the Democratic Republic of Congo and Gabon.Citation39 However, aligning GP genes of several circulating strains to that of our DNA vaccine reveals that almost all of the amino acid variations fall in the mucin domain. Although the significance of those observations remains to be determined, it is possible that antibody responses biased either toward or against this region might result in overall immunity differences. Nevertheless, we did not observe any statistically different antibody responses in ELISA conducted with pre-challenge sera against antigen with or without the mucin region, for either MARV or EBOV. Consequently, we found no correlation between antibody responses to the mucin domain and protection by the single or combination vaccines.
We also considered whether the difference in protection from EBOV vs MARV challenge in the combination vaccine groups was related to the fact that EBOV, but not MARV produces sGP. The EBOV and SUDV GP codon optimized DNA vaccines used in this study are unable to produce sGP. The 7U/8U genomic editing motif was removed by the optimization process. Thus, these plasmids only produce GP1,2. Consequently, the first exposure the NHP would have to sGP was after the live virus challenge. In an earlier study, Mohan et al. gave mice a priming vaccination with a construct similar to ours, which only generated GP1,2, and subsequently boosted the mice with a construct that only produced sGP.Citation40 Using competition ELISA, they demonstrated that sGP could compete for GP1,2 antibodies very effectively, and that pseudovirion neutralization titers dropped after boosting with the sGP construct. They theorized that their findings might suggest that the sGP produced during an EBOV infection might subvert the antibody response to GP1,2 in individuals receiving a vaccine that produces only GP1,2 unless the virus is rapidly cleared. This theory was not directly addressed in their studies, in that the mice were not challenged. In our earlier studies, we did not we did not see reduced protection in mice vaccinated with the same combination of filovirus vaccines that we tested here in NHP.Citation24 Nevertheless, it is possible that the sGP produced by EBOV, but not by MARV, after challenge contributed to the observed differences in protective immunity in the groups vaccinated with the combination vaccine, if the antibodies produced in NHP vaccinated with all 4 vaccines were less effective at clearing virus quickly than those produced in NHP vaccinated with only the EBOV GP vaccine. This might have occurred due to a dilution of the memory B-cells, which would be engaged in responses to additional filovirus proteins in the multiagent vaccine groups. Further targeted studies to address this possibility would be required to fully explore this possibility.
The responses that we found that did correlate with protection were the slightly higher GP-specific IgG responses of macaques vaccinated with the single vs the combination vaccines as determined by ELISA, and the higher GP-specific IgG and PsV-neutralizing antibody responses of macaques that survived EBOV challenge as compared to those that succumbed. The importance of neutralizing and non-neutralizing antibodies in protection from filovirus infections is incompletely understood. Several earlier studies have correlated antibodies with survival in both humans and animal models. For example, in laboratory studies, treatment of guinea pigs with multiple doses of a single neutralizing human monoclonal antibody (mAb) derived from an EBOV survivor prevented EBOV disease in rodentsCitation17; however, follow up studies with the same antibody failed to offer protection to NHP.Citation41 More recently, polyclonal IgG was found to provide complete protection against challenge with filoviruses in NHP when given up to 48 hours post exposure.Citation18 In addition, 2 groups showed partial protection of NHP following treatment with mAbs up to 24 hr post infection,Citation42,43 and another group reported complete protective immunity with a cocktail of mAbs.Citation19 In past human outbreaks, convalescent sera were used to treat infected patients of sporadic outbreaks of filoviruses with suggestive evidence for increased survival.Citation44,45 In the current outbreak, a cocktail of 3 MAbs (ZMapp, Mapp Biopharmaceutical, Inc., San Diego, CA), which is produced in tobacco plants,Citation46 has been used to treat infected medical workers.
Our ELISA results showed that the final total IgG antibody titers achieved against EBOV and MARV were slightly lower in macaques vaccinated with all 4 vaccines as compared to the individual vaccines. This finding may indicate dilution of antigen expression and concomitant immune response rather than specific immunodominance among the vaccine antigens. The lower titers did not negatively impact protection from MARV challenge as they did from EBOV challenge, which might relate to the closer relationship between MARV and RAVV than EBOV and SUDV. More importantly, in studies by others, cynomolgus macaques vaccinated with a recombinant vesicular stomatitis vectored vaccine expressing the GP gene of MARV were protected from challenge with RAVV.Citation47 Therefore, it is possible that the combination DNA vaccine that we used offered additive protection against MARV challenge due to the presence of both MARV and RAVV GP. In contrast, there is no reported cross protection between EBOV and SUDV, as illustrated in our earlier mouse studies in which vaccination with our SUDV GP DNA vaccine did not protect against challenge with mouse adapted EBOV.Citation24 Interestingly, our neutralizing antibody titers were comparable between the individual and combination vaccine groups. Regardless of the absence of overall differences in neutralizing antibody levels achieved in macaques given the single versus combination vaccines, our finding that the EBOV survivors had higher neutralizing titers than the non-survivors suggests that neutralizing antibodies probably contributed to the superior protection achieved with the single vaccine.
Certainly there are many other immunological responses that we were not able to measure that could either be equally or more important than antibody responses for vaccine efficacy, particularly cell-mediated immune responses. The only measure of cell-mediated immunity that we were able to obtain came from IFN-γ ELISpot assays, and in those assays we detected no differences in the responses between the single and combination groups.
In summary, the DNA vaccines that we have tested in this small study show great promise for further development and testing in humans. Further improvements that could be explored include modifications of the plasmid vector to promote specific immune responses, for example by co-expressing genes for cytokines or other immune modulators. It is also possible, that vaccination of the mixed vaccines would provide better protection against EBOV if each vaccine were delivered to a separate site rather than to a single site. This would eliminate the possibility that a large bolus of DNA (2 mg in our studies) is simply too much to process for the limited number of cells transfected at a single site. Finally, further work is clearly needed to define the correlates of protection for multiagent filovirus vaccines, which may include studies targeted to multiple ebolaviruses or multiple marburgviruses.
Methods
Vaccines
Synthesis and cloning of codon-optimized cDNAs encoding GP genes of EBOV-Kikwit 1995 (EBOV GP), SUDV-Gulu (SUDV GP), MARV-Musoke (MARV GP), and RAVV (RAVV GP) into the pWRG7077 expression plasmid were described previously.Citation24 Research grade plasmids used in these studies were manufactured by Aldevron (Fargo, ND). The codon optimization process resulted in ablation of the 7U/8U genomic editing motif in the GP of EBOV and SUDV. Thus, these plasmids do not express sGP.
Animal procurement and husbandry
All prechallenge animal husbandry, vaccinations, and specimen collections were performed by the University of Louisiana at Lafayette New Iberia Research Center (NIRC; Lafayette, LA). Healthy adult male and female (weight range: 3.64–5.39 kg; age range: 4.2–5.0 years) cynomolgus macaques (Macaca fascicularis) were procured from Covance Research Products (Princeton, NJ). Once vaccinations were complete, the animals were transferred to a biosafety level 4 containment laboratory at USAMRIID for EBOV or MARV challenges and subsequent housing. Research was conducted under an IACUC-approved protocol in compliance with the Animal Welfare Act, PHS Policy, and other Federal statutes and regulations relating to animals and experiments involving animals. The NIRC and USAMRIID facilities where this research was conducted are accredited by the Association for Assessment and Accreditation of Laboratory Animal Care, International and adhere to principles stated in the Guide for the Care and Use of Laboratory Animals, National Research Council, 2011.
Nonhuman primate study designs and vaccinations
Two studies were performed, each consisting of 13 cynomolgus macaques that were statistically randomized into treatment groups based on gender and weight (Table S1). In each study, 6 macaques received the individual MARV-GP or EBOV-GP DNA vaccine and 6 macaques received a mixture of the EBOV-GP, SUDV-GP, MARV-GP, and RAVV-GP DNA vaccines. Prior to each vaccination, animals were anesthetized by injection of a ketamine (5–15 mg/kg) and xylazine (0.5–2 mg/kg) mixture into the buttocks and approximately 10–20 ml of blood was collected from the femoral artery for cryopreservation of peripheral blood mononuclear cells (PBMCs) and serum isolation. The macaques were vaccinated in the vastus lateralis muscle with 500 μl of DNA plasmid solution (1 μg/μl of each plasmid; 500 μg or 2 mg total DNA for single and combination vaccines, respectively) diluted in calcium- and magnesium-free phosphate buffered saline using Ichor Medical Systems' TriGrid Delivery System intramuscular electroporation (IM-EP) device as previously described.Citation28 One control macaque in each study was vaccinated with the empty vector under the same dose and conditions as the animals receiving the DNA vaccine candidates. Each animal was vaccinated at 28-day intervals, for a total of 3 vaccinations. Each vaccination was administered in an alternating limb from the previous one. Sera and PBMCs were collected prior to each vaccination and 28 d after the final vaccination to examine the prechallenge vaccine-induced humoral and cellular immune responses. Blood collected prior to and following filovirus challenge was assessed for viremia, hematology, and blood chemistry analyses.
Filovirus challenges
Eight weeks after the final vaccination (day 112), animals received intramuscular injections of 1 × 103 plaque forming units of Ebola virus H.sapiens-tc/COD/1995/Kikwit or Marburg virus H.sapiens-tc/KEN/1980/Musoke-MV6 diluted in Dulbecco's Modified Eagle Medium (DMEM; Life Technologies) supplemented with 1% (vol/vol) heat-inactivated fetal bovine serum (ΔFBS; Life Technologies, Carlsbad, CA).
The EBOV challenge stock was a Biological Reference Material produced by the Critical Reagents Program with the designation “Zaire ebolavirus, Strain: Kikwit (aka Zaire 95), Lot: R4368 Manufacturing Date: 07/24/2011.” This stock was the fourth Vero E6 cell passage of clinical material (USAMRIID P2). The virus population consisted predominantly of 8U genomes. The EBOV challenge stock had a titer of 4.56 × 106 PFU/ml and there were 2.35 × 1010 genome equivalents/ml, resulting in a genome equivalent to PFU ratio of 5,144. Supernatant was collected from cultures at 3+ cytopathic effect (4 d post-inoculation). The supernatant virus was clarified by centrifugation (3,000 xg for 10 min). The MARV challenge stock used was passaged 6 times in Vero 76 cells as described previously.Citation15
Quantitative indirect ELISA
Filovirus GP-specific IgG serum antibody concentrations were determined by indirect ELISA using HEK 293T-expressed soluble, trimeric EBOV-Mayinga 1976, SUDV-Boniface, and MARV-Musoke GPs without the transmembrane domain, and with or without the mucin domain, as antigens. Expression plasmids containing GP constructs were kindly provided to Integrated BioTherapeutics by Dr. Erica Ollmann Saphire (The Scripps Research Institute). The GP genes without the transmembrane domains (GPΔTM) correspond to amino acids 32–637 (EBOV and SUDV) or 1–636 (MARV). For the mucin-like domain deleted antigens (ΔMuc), additional nucleotides were removed encoding amino acid positions 312–462 (EBOV), 314–472 (SUDV), or 279–422 (MARV) (Citation48 and unpublished data). An optimized quantity of each purified antigen (40–250 ng/well) was immobilized on 96-well MaxiSorp plates (Thermo Fisher Scientific) overnight at 2–8°C. Plates were blocked using StartingBlock T20 (PBS) Blocking Buffer (Thermo Fisher Scientific). Bound antibodies were detected using various dilutions of goat anti-human IgG-HRP (1:4000–1:8000 dilution as optimized for each assay; KPL). The ELISAs were developed using 3,3′,5,5′-tetramethylbenzidine substrate (KPL) for 30 min at room temperature and then absorbance at λ = 650 nm was measured using a VersaMax plate reader (Molecular Devices). In this ELISA, the antibodies in unknown (test) samples are determined based on a standard curve (using a 4PL fit in SoftMax Pro v5.4; Molecular Devices) of a positive control reference detection antibody (RDA) derived from pooled sera or plasma of cynomolgus macaques that were immunized with filovirus VLPs. The dilution of the positive control sera or plasma, at the inflection point of each 4PL curve, was used to establish the number of Antibody Units/mL (Ab U/mL) for the RDA. The RDA with an assigned Ab U/mL was tested as a standard curve on every ELISA plate to quantify the Ab U/mL of the unknown (test) samples. The historically assigned values for these RDA were 2302 Ab U/ml (EBOV), 1661 Ab U/ml (SUDV), and 2389 Ab U/ml (MARV). Each test sample was analyzed at 2 dilutions (1:100 and 1:1000) as single replicates.
Pseudovirion neutralization assay (PsVNA)
Luciferase-expressing pseudovirions (PsVs) were prepared using a modified version of previously described methodsCitation34,49 using the pWRG7077 plasmid-based DNA vaccines to express the filovirus GPs.Citation24 Macaque sera were heat-inactivated at 56°C for 30 min and then an initial 1:10 dilution was prepared followed by five-fold serial dilutions in Eagle's Minimum Essential Medium (Life Technologies) supplemented with 10% (vol/vol) ΔFBS, 100 IU/ml penicillin, and 100 μg/ml streptomycin (cEMEM) (1:10–1:781,250). Each sample was analyzed in triplicate. Each type of filovirus GP-bearing VSVΔG*rLuc PsV was diluted in cEMEM containing 10% (vol/vol) human complement (Sigma-Aldrich). An equal volume of each filovirus PsV solution containing 4 × 103 focus-forming units was added to the sera dilutions and then incubated overnight at 4°C. Vero cell monolayers seeded in clear-bottom black 96-well plates (Corning) were infected with 50 μl of each PsV-serum mixture and then incubated at 37°C for an additional 18–24 hrs. The medium was discarded, the cells were lysed, and luciferase substrate was added according to the Renilla Luciferase Assay System protocol (Promega #E2820). The flash luciferase signal was measured using a Tecan M200 microplate reader. The data were exported to GraphPad Prism version 6.04, where the serum dilutions (x-axis) were log-transformed and the luminescence data (y-axis) were baseline-corrected to the untreated PsV signal. The data were fit to 4 parameter logistic nonlinear regression models using GraphPad Prism and then PsVNA 50% (PsVNA50) neutralization titers were interpolated from the curves for each sample.
Interferon-γ ELISpot
For ELISpot assays, the Mabtech macaque IFN-γ ELISpotPRO kit was used according to the manufacturer's recommendations. In brief, cryopreserved macaque PBMCs were thawed at 37°C in Roswell Park Memorial Institute Medium (Life Technologies) supplemented with 10% (vol/vol) ΔFBS, 2 mM L-glutamine, 2 mM sodium pyruvate, 20 mM 4-(2-Hydroxyethyl)piperazine-1-ethanesulfonic acid, 55 μM β-mercaptoethanol, 100 IU/ml penicillin, and 100 μg/ml streptomycin (cRPMI) and plated in T25 flasks. Pools of overlapping EBOV- or MARV-GP derived peptides (final concentration 10 μg/ml) were combined with approximately 2 × 105 PBMCs per well, added to precoated ELISpot plates, and incubated at 37°C for approximately 48 hrs. The cells and medium were removed, and IFN-γ spots were detected using kit-provided primary antibody, alkaline phosphatase-conjugated secondary antibody, and a substrate mixture of 5-bromo-4-chloro-3′-indolyphosphate p-toluidine salt and nitro-blue tetrazolium chloride (BCIP/NBT). Spots were imaged and quantified by Zellnet Consulting (Fort Lee, NJ).
Statistical analyses
ELISA titers were analyzed over time and by type of vaccine using a repeated measures, 2-way ANOVA with Tukey's or Sidak's multiple comparison test, as appropriate. The following comparisons were made: (1) between vaccination groups, (2) across time points, and (3) between vaccination groups, at each time point. To compare PsVNA titers and ELISpot data between groups at a single time point, unpaired 2-tailed t tests were employed. Kaplan-Meier curves were used to determine if there were statistically significant differences in survival among the 3 vaccine groups in each study. The main purpose of the pWRG7077-vaccinated control arms in these studies was to verify lethality of the challenges. Secondarily, with 6 macaques in each of the treatment arms, it would be possible to declare that the treatment arms differ significantly from the control arm if all 6 macaques survived while the control macaque succumbed. This did not occur for both treatment arms in either study, and so, in order to be able to provide a more conclusive analysis in comparing treatment to controls, macaques from previous studies were included as controls (15 for EBOV and 3 for MARV). These animals were chosen because they matched the control animals in this study in species, challenge route, and virus. Specifically, they were cynomolgus macaques that were inoculated with EBOV or MARV through the intramuscular route. One difference in the EBOV study is that the historical controls were challenged with older virus stocks. For the MARV study, historical controls received the same virus stock as in the present study. The Kaplan-Meier curves were evaluated based on the log-rank test for overall homogeneity. Pairwise comparisons were also made using the log-rank test with a post hoc Tukey-Kramer adjustment for multiple testing. Due to small sample size, differences in overall survival between groups were evaluated using Fisher's exact test with multiple testing adjusted by permutation. Statistical significance was defined as α = 0.05 in all tests.
Disclosure of Potential Conflicts of Interest
D.H. is Vice President, Research and Development of Ichor Medical Systems. H.A.V is employed by Integrated BioTherapeutics, Inc. K.L.W. was formerly Vice President, Vaccine Development, for Integrated BioTherapeutics, Inc. C.S.S, J.W.H, and Ichor Medical Systems have a variety of ongoing collaborative research and development agreements for vaccine development. The authors declare no other competing interests.
Supplemental_Material.docx
Download MS Word (23.8 KB)Acknowledgments
The authors thank Joshua Moore, James Fiallos, Darrell Wetzel, Eugene Blue, and William Agee for performing the sample collection and blinded animal health assessments in BSL-4. Further, the authors thank Dr. Jason Goetzman for his coordination of the vaccinations at NIRC; LTC Bridget S. Lewis, LTC Paul R. Facemire, and LTC Shelley Honnold for pathology support (USAMRIID); Dr. Scott Marshall (BioStat Solutions, Inc.) and Dr. Samuel P. Dickson (USAMRIID) for statistical analysis; and Angela Silva, Audra Gravatt, and Stephanie McNulty (The Geneva Foundation) for administrative support.
Funding
The studies described here were supported by Grant Number U01AI082069 from the National Institute of Allergy And Infectious Diseases. Production of the EBOV challenge stock used here was funded by the Joint Program Executive Office for Chemical and Biological Defense, Medical Countermeasures Systems Joint Project Management Office. Opinions, interpretations, conclusions, and recommendations contained within this presentation are those of the authors and are not necessarily endorsed by the United States Army, the National Institute of Allergy and Infectious Diseases, or the National Institutes of Health.
Supplemental Material
Supplemental data for this article can be accessed on the publisher's website.
References
- Kuhn JH, Becker S, Ebihara H, Geisbert TW, Johnson KM, Kawaoka Y, Lipkin WI, Negredo AI, Netesov SV, Nichol ST, et al. Proposal for a revised taxonomy of the family Filoviridae: classification, names of taxa and viruses, and virus abbreviations. Arch Virol 2010; 155:2083-103; PMID:21046175; http://dx.doi.org/10.1007/s00705-010-0814-x
- Kuhn JH. Filoviruses. A compendium of 40 years of epidemiological, clinical, and laboratory studies. Arch Virol Suppl 2008; 20:13-360; PMID:18637412; http://dx.doi.org/10.1007/978-3-211-69495-4_1
- Towner JS, Amman BR, Sealy TK, Carroll SA, Comer JA, Kemp A, Swanepoel R, Paddock CD, Balinandi S, Khristova ML, et al. Isolation of genetically diverse Marburg viruses from Egyptian fruit bats. PLoS Path 2009; 5:e1000536; PMID:19649327; http://dx.doi.org/10.1371/journal.ppat.1000536
- Leroy EM, Kumulungui B, Pourrut X, Rouquet P, Hassanin A, Yaba P, Délicat A, Paweska JT, Gonzalez JP, Swanepoel R. Fruit bats as reservoirs of Ebola virus. Nature 2005; 438:575-6; PMID:16319873; http://dx.doi.org/10.1038/438575a
- Feldmann H, Klenk HD, Sanchez A. Molecular biology and evolution of filoviruses. Arch Virol Suppl 1993; 7:81-100; PMID:8219816; http://dx.doi.org/10.1007/978-3-7091-9300-6_8
- Sanchez A, Kiley MP, Holloway BP, Auperin DD. Sequence analysis of the Ebola virus genome: organization, genetic elements, and comparison with the genome of Marburg virus. Virus Res 1993; 29:215-40; PMID:8237108; http://dx.doi.org/10.1016/0168-1702(93)90063-S
- Sanchez A, Trappier SG, Mahy BW, Peters CJ, Nichol ST. The virion glycoproteins of Ebola viruses are encoded in two reading frames and are expressed through transcriptional editing. Proc Natl Acad Sci U S A 1996; 93:3602-7; PMID:8622982; http://dx.doi.org/10.1073/pnas.93.8.3602
- Volchkov VE, Becker S, Volchkova VA, Ternovoj VA, Kotov AN, Netesov SV, Klenk HD. GP mRNA of Ebola virus is edited by the Ebola virus polymerase and by T7 and vaccinia virus polymerases. Virology 1995; 214:421-30; PMID:8553543; http://dx.doi.org/10.1006/viro.1995.0052
- Volchkov VE, Feldmann H, Volchkova VA, Klenk HD. Processing of the Ebola virus glycoprotein by the proprotein convertase furin. Proc Natl Acad Sci U S A 1998; 95:5762-7; PMID:9576958; http://dx.doi.org/10.1073/pnas.95.10.5762
- Jones SM, Stroher U, Fernando L, Qiu X, Alimonti J, Melito P, Bray M, Klenk HD, Feldmann H. Assessment of a vesicular stomatitis virus-based vaccine by use of the mouse model of Ebola virus hemorrhagic fever. J Infect Dis 2007; 196 Suppl 2:S404-12; PMID:17940977; http://dx.doi.org/10.1086/520591
- Bukreyev A, Yang L, Zaki SR, Shieh WJ, Rollin PE, Murphy BR, Collins PL, Sanchez A. A single intranasal inoculation with a paramyxovirus-vectored vaccine protects guinea pigs against a lethal-dose Ebola virus challenge. J Virol 2006; 80:2267-79; PMID:16474134; http://dx.doi.org/10.1128/JVI.80.5.2267-2279.2006
- Swenson DL, Wang D, Luo M, Warfield KL, Woraratanadharm J, Holman DH, Dong JY, Pratt WD. Vaccine to confer to nonhuman primates complete protection against multistrain Ebola and Marburg virus infections. Clin Vaccine Immunol 2008; 15:460-7; PMID:18216185; http://dx.doi.org/10.1128/CVI.00431-07
- Sullivan NJ, Sanchez A, Rollin PE, Yang ZY, Nabel GJ. Development of a preventive vaccine for Ebola virus infection in primates. Nature 2000; 408:605-9; PMID:11117750; http://dx.doi.org/10.1038/35046108
- Sullivan NJ, Geisbert TW, Geisbert JB, Xu L, Yang ZY, Roederer M, Koup RA, Jahrling PB, Nabel GJ. Accelerated vaccination for Ebola virus haemorrhagic fever in non-human primates. Nature 2003; 424:681-4; PMID:12904795; http://dx.doi.org/10.1038/nature01876
- Hevey M, Negley D, Pushko P, Smith J, Schmaljohn A. Marburg virus vaccines based upon alphavirus replicons protect guinea pigs and nonhuman primates. Virology 1998; 251:28-37; PMID:9813200; http://dx.doi.org/10.1006/viro.1998.9367
- Warfield KL, Posten NA, Swenson DL, Olinger GG, Esposito D, Gillette WK, Hopkins RF, Costantino J, Panchal RG, Hartley JL, et al. Filovirus-like particles produced in insect cells: immunogenicity and protection in rodents. J Infect Dis 2007; 196 Suppl 2:S421-9; http://dx.doi.org/10.1086/520612
- Parren PW, Geisbert TW, Maruyama T, Jahrling PB, Burton DR. Pre- and postexposure prophylaxis of Ebola virus infection in an animal model by passive transfer of a neutralizing human antibody. J Virol 2002; 76:6408-12; PMID:12021376; http://dx.doi.org/10.1128/JVI.76.12.6408-6412.2002
- Dye JM, Herbert AS, Kuehne AI, Barth JF, Muhammad MA, Zak SE, Ortiz RA, Prugar LI, Pratt WD. Postexposure antibody prophylaxis protects nonhuman primates from filovirus disease. Proc Natl Acad Sci U S A 2012; 109:5034-9; PMID:22411795; http://dx.doi.org/10.1073/pnas.1200409109
- Qiu X, Audet J, Wong G, Pillet S, Bello A, Cabral T, Strong JE, Plummer F, Corbett CR, Alimonti JB, et al. Successful treatment of ebola virus-infected cynomolgus macaques with monoclonal antibodies. Sci Transl Med 2012; 4:138ra81; PMID:22700957; http://dx.doi.org/10.1126/scitranslmed.3003876
- Marzi A, Engelmann F, Feldmann F, Haberthur K, Shupert WL, Brining D, Scott DP, Geisbert TW, Kawaoka Y, Katze MG, et al. Antibodies are necessary for rVSV/ZEBOV-GP-mediated protection against lethal Ebola virus challenge in nonhuman primates. Proc Natl Acad Sci U S A 2013; 110:1893-8; PMID:23319647; http://dx.doi.org/10.1073/pnas.1209591110
- Sullivan NJ, Hensley L, Asiedu C, Geisbert TW, Stanley D, Johnson J, Honko A, Olinger G, Bailey M, Geisbert JB, et al. CD8+ cellular immunity mediates rAd5 vaccine protection against Ebola virus infection of nonhuman primates. Nat Med 2011; 17:1128-31; PMID:21857654; http://dx.doi.org/10.1038/nm.2447
- Riemenschneider J, Garrison A, Geisbert J, Jahrling P, Hevey M, Negley D, Schmaljohn A, Lee J, Hart MK, Vanderzanden L, et al. Comparison of individual and combination DNA vaccines for B. anthracis, Ebola virus, Marburg virus and Venezuelan equine encephalitis virus. Vaccine 2003; 21:4071-80; PMID:12922144; http://dx.doi.org/10.1016/S0264-410X(03)00362-1
- Mellquist-Riemenschneider JL, Garrison AR, Geisbert JB, Saikh KU, Heidebrink KD, Jahrling PB, et al. Comparison of the protective efficacy of DNA and baculovirus-derived protein vaccines for EBOLA virus in guinea pigs. Virus Res 2003; 92:187-93; PMID:12686428; http://dx.doi.org/10.1016/S0168-1702(02)00338-6
- Grant-Klein RJ, Van Deusen NM, Badger CV, Hannaman D, Dupuy LC, Schmaljohn CS. A multiagent filovirus DNA vaccine delivered by intramuscular electroporation completely protects mice from ebola and Marburg virus challenge. Hum Vacc Immunother 2012; 8:1703-6; PMID:22922764; http://dx.doi.org/10.4161/hv.21873
- Nakayama E, Saijo M. Animal models for Ebola and Marburg virus infections. Front Microbiol 2013; 4:267; PMID:24046765; http://dx.doi.org/10.3389/fmicb.2013.00267
- Hevey M, Negley D, VanderZanden L, Tammariello RF, Geisbert J, Schmaljohn C, Smith JF, Jahrling PB, Schmaljohn AL. Marburg virus vaccines: comparing classical and new approaches. Vaccine 2001; 20:586-93; PMID:11672925; http://dx.doi.org/10.1016/S0264-410X(01)00353-X
- Schmaljohn CS, Spik KW, Hooper JW. DNA vaccines for HFRS: laboratory and clinical studies. Virus Res 2014; 187:91-6; PMID:24370868; http://dx.doi.org/10.1016/j.virusres.2013.12.020
- Dupuy LC, Richards MJ, Ellefsen B, Chau L, Luxembourg A, Hannaman D, Livingston BD, Schmaljohn CS. A DNA vaccine for venezuelan equine encephalitis virus delivered by intramuscular electroporation elicits high levels of neutralizing antibodies in multiple animal models and provides protective immunity to mice and nonhuman primates. Clin Vaccine Immunol 2011; 18:707-16; PMID:21450977; http://dx.doi.org/10.1128/CVI.00030-11
- Spik KW, Badger C, Mathiessen I, Tjelle T, Hooper JW, Schmaljohn C. Mixing of M segment DNA vaccines to Hantaan virus and Puumala virus reduces their immunogenicity in hamsters. Vaccine 2008; 26:5177-81; PMID:18482782; http://dx.doi.org/10.1016/j.vaccine.2008.03.097
- Hooper JW, Moon JE, Paolino KM, Newcomer R, McLain DE, Josleyn M, Hannaman D, Schmaljohn C. A Phase 1 clinical trial of Hantaan virus and Puumala virus M-segment DNA vaccines for haemorrhagic fever with renal syndrome delivered by intramuscular electroporation. Clin Microbiol Infect 2014; 20 Suppl 5:110-7; PMID:24447183; http://dx.doi.org/10.1111/1469-0691.12553
- Boudreau EF, Josleyn M, Ullman D, Fisher D, Dalrymple L, Sellers-Myers K, Loudon P, Rusnak J, Rivard R, Schmaljohn C, et al. A Phase 1 clinical trial of Hantaan virus and Puumala virus M-segment DNA vaccines for hemorrhagic fever with renal syndrome. Vaccine 2012; 30:1951-8; PMID:22248821; http://dx.doi.org/10.1016/j.vaccine.2012.01.024
- Hooper JW, Josleyn M, Ballantyne J, Brocato R. A novel Sin Nombre virus DNA vaccine and its inclusion in a candidate pan-hantavirus vaccine against hantavirus pulmonary syndrome (HPS) and hemorrhagic fever with renal syndrome (HFRS). Vaccine 2013; 31:4314-21; PMID:23892100; http://dx.doi.org/10.1016/j.vaccine.2013.07.025
- Brocato RL, Josleyn MJ, Wahl-Jensen V, Schmaljohn CS, Hooper JW. Construction and nonclinical testing of a Puumala virus synthetic M gene-based DNA vaccine. Clin Vaccine Immunol 2013; 20:218-26; PMID:23239797; http://dx.doi.org/10.1128/CVI.00546-12
- Kwilas S, Kishimori JM, Josleyn M, Jerke K, Ballantyne J, Royals M, Hooper JW. A Hantavirus Pulmonary Syndrome (HPS) DNA Vaccine Delivered Using a Spring-powered Jet Injector Elicits a Potent Neutralizing Antibody Response in Rabbits and Nonhuman Primates. Curr Gene Ther 2014; 14:200-10; PMID:24867065; http://dx.doi.org/10.2174/1566523214666140522122633
- Dupuy LC, Richards MJ, Reed DS, Schmaljohn CS. Immunogenicity and protective efficacy of a DNA vaccine against Venezuelan equine encephalitis virus aerosol challenge in nonhuman primates. Vaccine 2010; 28:7345-50; PMID:20851089; http://dx.doi.org/10.1016/j.vaccine.2010.09.005
- Hood CL, Abraham J, Boyington JC, Leung K, Kwong PD, Nabel GJ. Biochemical and structural characterization of cathepsin L-processed Ebola virus glycoprotein: implications for viral entry and immunogenicity. J Virol 2010; 84:2972-82; PMID:20053739; http://dx.doi.org/10.1128/JVI.02151-09
- Shedlock DJ, Bailey MA, Popernack PM, Cunningham JM, Burton DR, Sullivan NJ. Antibody-mediated neutralization of Ebola virus can occur by two distinct mechanisms. Virology 2010; 401:228-35; PMID:20304456; http://dx.doi.org/10.1016/j.virol.2010.02.029
- Wilson JA, Hevey M, Bakken R, Guest S, Bray M, Schmaljohn AL, Hart MK. Epitopes involved in antibody-mediated protection from Ebola virus. Science 2000; 287:1664-6; PMID:10698744; http://dx.doi.org/10.1126/science.287.5458.1664
- Baize S, Pannetier D, Oestereich L, Rieger T, Koivogui L, Magassouba N, Soropogui B, Sow MS, Keïta S, De Clerck H, et al. Emergence of Zaire Ebola Virus Disease in Guinea – Preliminary Report. N Engl J Med 2014; 371(15):1418-25; PMID:24738640
- Mohan GS, Li W, Ye L, Compans RW, Yang C. Antigenic subversion: a novel mechanism of host immune evasion by Ebola virus. PLoS Path 2012; 8:e1003065; PMID:23271969; http://dx.doi.org/10.1371/journal.ppat.1003065
- Oswald WB, Geisbert TW, Davis KJ, Geisbert JB, Sullivan NJ, Jahrling PB, Parren PW, Burton DR. Neutralizing antibody fails to impact the course of Ebola virus infection in monkeys. PLoS Path 2007; 3:e9; PMID:17238286; http://dx.doi.org/10.1371/journal.ppat.0030009
- Marzi A, Yoshida R, Miyamoto H, Ishijima M, Suzuki Y, Higuchi M, Matsuyama Y, Igarashi M, Nakayama E, Kuroda M, et al. Protective efficacy of neutralizing monoclonal antibodies in a nonhuman primate model of Ebola hemorrhagic fever. PloS One 2012; 7:e36192; PMID:22558378; http://dx.doi.org/10.1371/journal.pone.0036192
- Olinger GG, Jr., Pettitt J, Kim D, Working C, Bohorov O, Bratcher B, Hiatt E, Hume SD, Johnson AK, Morton J, et al. Delayed treatment of Ebola virus infection with plant-derived monoclonal antibodies provides protection in rhesus macaques. Pro Nat Acad Sci U S A 2012; 109:18030-5; PMID:23071322; http://dx.doi.org/10.1073/pnas.1213709109
- Emond RT, Evans B, Bowen ET, Lloyd G. A case of Ebola virus infection. Br Med J 1977; 2:541-4; PMID:890413; http://dx.doi.org/10.1136/bmj.2.6086.541
- Mupapa K, Massamba M, Kibadi K, Kuvula K, Bwaka A, Kipasa M, Colebunders R, Muyembe-Tamfum JJ. Treatment of Ebola hemorrhagic fever with blood transfusions from convalescent patients. International Scientific and Technical Committee. J Infect Dis 1999; 179 Suppl 1:S18-23; PMID:9988160; http://dx.doi.org/10.1086/514298
- Qiu X, Wong G, Audet J, Bello A, Fernando L, Alimonti JB, Fausther-Bovendo H, Wei H, Aviles J, Hiatt E, et al. Reversion of advanced Ebola virus disease in nonhuman primates with ZMapp. Nature 2014; 514(7520):47-53; PMID:25171469
- Daddario-DiCaprio KM, Geisbert TW, Geisbert JB, Stroher U, Hensley LE, Grolla A, Fritz EA, Feldmann F, Feldmann H, Jones SM. Cross-protection against Marburg virus strains by using a live, attenuated recombinant vaccine. J Virol 2006; 80:9659-66; PMID:16973570; http://dx.doi.org/10.1128/JVI.00959-06
- Lee JE, Fusco ML, Hessell AJ, Oswald WB, Burton DR, Saphire EO. Structure of the Ebola virus glycoprotein bound to an antibody from a human survivor. Nature 2008; 454:177-82; PMID:18615077; http://dx.doi.org/10.1038/nature07082
- Ogino M, Ebihara H, Lee BH, Araki K, Lundkvist A, Kawaoka Y, Yoshimatsu K, Arikawa J. Use of vesicular stomatitis virus pseudotypes bearing hantaan or seoul virus envelope proteins in a rapid and safe neutralization test. Clin Diag Lab Immunol 2003; 10:154-60; PMID:12522053