ABSTRACT
Among all natural and synthetic toxins, botulinum neurotoxins (BoNTs), produced by Clostridium botulinum in an anaerobic environment, are the most toxic polymer proteins. Currently, the most effective modalities for botulism prevention and treatment are vaccination and antitoxin use, respectively. However, these modalities are associated with long response time for active immunization, side effects, and donor limitations. As such, the development of more promising botulism prevention and treatment modalities is warranted. Here, we designed an mRNA encoding B9-hFc – a heavy-chain antibody fused to VHH and human Fc that can neutralize BoNT serotype B (BoNT/B) effectively – and assessed its expression in vitro and in vivo. The results confirmed that our mRNA demonstrates good expression in vitro and in vivo. Moreover, a single mRNA lipid nanoparticle injection effectively prevents BoNT/B intoxication in vivo, with effects comparable to those of protein antibodies. In conclusion, we explored and clarified whether mRNA drugs encoding neutralizing antibodies prevent BoNT/B intoxication. Our results provide an efficient strategy for further research on the prevention and treatment of intoxication by botulinum toxin.
Introduction
Botulinum neurotoxins (BoNTs), produced by anaerobic bacteria of the genus Clostridium, cause flaccid paralysis of muscles by inhibiting acetylcholine release from nerve endings and are among the most toxic proteins in nature. Six phylogenetically distinct groups of Clostridium produce seven serotypes of BoNTs (BoNT/A – BoNT/G;Citation1,Citation2 of them, BoNT/A, BoNT/B, BoNT/E, and BoNT/F (rare) are the main causes of botulism in humans.Citation3,Citation4 Botulism is a rare but serious motor nerve-paralyzing disease with rapid onset and high mortality; botulism types are classified into six main categories: food botulism, infant botulism, wound botulism, adult intestinal colonization, iatrogenic botulism, and inhalation botulism.Citation5
BoNT consists of a heavy chain (HC; molecular weight ≈ 100 kDa) and a light chain (LC; molecular weight ≈ 50 kDa). The BoNT-LC is BoNT’s catalytic domain composed of a metalloproteinase, which inhibits neurotransmitter release from peripheral nerve endings, thus resulting in botulism. The BoNT-HC contains a receptor-binding domain (Hc domain) and a transporter structural domain (HN domain), which enable toxin absorption in neurons.Citation6,Citation7 Neutralizing antibodies targeting the Hc domain inhibit the binding of BHc to receptors on nerve ending membranes, thereby neutralizing the toxin and effectively protecting against BoNT/B intoxication.
In general, vaccination is the most effective means of preventing botulism; however, current pentavalent vaccines against BoNT/A, BoNT/B, BoNT/C, BoNT/D, and BoNT/E have not been approved for human use because their efficacy and side effects remain unconfirmed.Citation8 Unlike antibody-based passive immunization, vaccines do not swiftly generate protective immunity, which limits their application. Particularly during acute intoxication, antibodies confer a critical advantage to a certain extent.Citation9 Equine anti-botulinum serum is currently the only specific therapy for clinical use in patients who have been intoxicated; however, equine immune serum may cause serum sickness and anaphylactic shock.Citation10,Citation11 This necessitates research on modalities for clinical prevention and treatment of BoNT intoxication.
In recent years, mRNAs have been an emerging class of therapeutic drugs for the prevention and treatment of various diseases. mRNA delivery of neutralizing antibody genes has also become an attractive disease prevention strategy. In the early 1990s, exogenous mRNA was reported to direct protein expression in vivo.Citation12 Since then, mRNA technology has emerged as a promising drug platform, showcased by the success of mRNA vaccines which demonstrated its potential and developmental prospects.Citation13 Another rapidly growing area of mRNA technology is in therapeutic antibodies, now one of the fastest-growing pharmaceutical sectors in the market. Compared with antibody drugs, mRNA-encoded antibodies may have some advantages: simpler production process, higher efficiency, easier emergency preparation ability, low overall and reserve costs, more sustained neutralizing antibody levels, and more long-lasting protective efficacy. Currently, mRNA-encoded antibodies have been applied in the treatment of diseases including viral infections, toxin exposure and malignant tumors.Citation9,Citation14,Citation15 Previous studies reported that formulated RNA encoding VHH heteromultimers confers prevention and therapeutic protection against botulinum intoxication.Citation9,Citation16 These studies highlighted RNA therapy as an established method for rapidly developing and delivering botulinum toxin-neutralizing antibodies.
Our previous study has obtained a heavy chain antibody B9-hFc (the fusion protein of camel-derived VHH and Fc region of human antibody), which has BoNT/B-potent neutralizing activity.Citation17 In this study, we designed and synthesized molecules of mRNA encoding B9-hFc, which established rapid and long-lasting serum antibody levels in vivo and demonstrated preventive effects against lethal doses of BoNT/B. This underscored the potential of mRNA-based therapies in combating type B botulism. Our results provide a feasible reference for exploring alternative protein therapies with reduced cost and increased convenience, which is significant in enhancing prevention and treatment of type B botulism.
Materials and methods
mRNA preparation
The mRNA sequence, containing the phage promoter (T7), 5′ UTR, ORF, and 3′UTR, was designed, synthesized, and cloned into the vector pUC57 to construct a DNA transcription template. The 5′UTR comprised a sequence with high ribosome loading from the NCBI Gene Expression Omnibus database (UTR4; original library ID: 317915).Citation18 The 3′UTR comprised mRNA sequences derived from mitochondrial 12S rRNA-related sequences and amino-terminal enhancer of split.Citation19 The mRNA molecules were synthesized through transcription in an in vitro cell-free system by using T7 RNA polymerase (Vazyme, DD4202) using the linearized plasmid template obtained through XbaI (NEB, R0145V) digestion. To reduce immunogenicity, we substituted uridine in the RNA molecules with pseudouridine. The transcribed samples were purified using magnetic beads (Vazyme, N412) for enzyme, free nucleotide, residual DNA, and exogenous RNA fragment removal. The cap structure (m7Gppp, Cap0) was added to the 5′ end of the mRNAs by using the Vaccinia Capping System (Vazyme, DD4109); next, a methyl group was added to the 2′-O site of the first nucleotide immediately adjacent to the cap structure (Cap0) at the 5′ end of the mRNA by using Cap 2′-O-Methyltransferase, enabling the formation of mRNAs with a Cap1 structure. The poly(A) tail was added at the 3′ end by E. coli poly(A) polymerase (NEB, M0276).
Cell culture and antibody expression
Fresh Dulbecco’s Modified Eagle Medium (Gibco 11,965–092) supplemented with 10% fetal bovine serum (Gibco, A5669401), 100 U/mL penicillin, and 100 mg/mL streptomycin (Gibco 15,140,122) was prepared for the culture of CHO and COS-7 cells (maintained in our laboratory). HEK-293F cells were cultured in FreeStyleTM 293 Expression medium (Gibco 12,338–018). The cells were incubated at 37°C in a 5% CO2 constant temperature cell incubator.
HEK-293F, COS-7, and CHO cells were seeded into 24-well plates at 200,000 cells per well and incubated for 24 h. The cell attachment and growth level were observed, with 70%–90% confluency considered optimal. In each well, the cells were transfected with 1.5 μL of the transfection reagent LipofectamineTM MessengerMAXTM (Thermo Fisher Scientific, LMRNA008) with 0.5 μg of mRNA. Cell supernatants were collected 48 h after transfection, and the antibody expression levels were quantified through ELISA.
The expression vector for the recombinant VHH-hFc protein was transfected into HEK-293F cells and cultured for 3–4 days. Subsequently, the recombinant VHH-hFc protein was purified from the supernatant using the AKTA Pure purification system and HiTrapTM MabSelect column. The obtained recombinant protein B9-hFc was used in ELISA experiments to plot a standard curve to quantify the antibody concentration in the supernatant.
ELISA
The mRNA expression levels in vitro and in vivo were quantified by ELISA. The target antigen was encapsulated in a 96-well enzyme-linked plate (Corning 42,592) at 200 ng/well incubated at 4°C overnight. The plates were blocked with phosphate-buffered saline (PBS) containing 2.5% skimmed milk powder, while the blocking buffer (diluent) for the cell supernatants, mouse sera, recombinant B9-Fc as standard sample or second antibody was added extra 0.1% Tween 20. The initial concentration of recombinant B9-hFc protein was set at 0.25 μg/ml, and it was serially diluted in 12 twofold gradients to plot a standard curve. The cell supernatants were initially diluted 10-fold and then serially diluted in 6 twofold gradients. Similarly, mouse serum was diluted 40-fold and then serially diluted in 6 threefold gradients before being incubated at 37°C for 1 hour.
Then, the diluted recombinant protein, cell supernatant or mouse serum were added to the plates at 100 µL/well, allowed to bind at 37°C for 1 h, and finally, washed. We added dilute HRP-labeled goat anti-human immunoglobulin G(ZSGB-Bio, ZB-2304) at a 1:5,000 ratio. Next, this mixture was added at 100 µL/well to the ELISA plates and allowed to bind at 37°C for 45 min. Next, the plates were washed, and TMB colorimetric substrate was added at 100 µL/well. The color development process was terminated after the plates were allowed to stand at room temperature for 5–10 min. The absorbance values were read at 450 nm, with 595 nm as the reference wavelength (Thermo Multiskan MK3). We plotted the resulting curves by using GraphPad Prism (version 9.0).
LNP production process
SM-102, distearoylphosphatidylcholine, cholesterol, and mPEG-DMG-2K were dispersed in anhydrous ethanol, at molar ratios of 50:10:38.5:1.5; after mixing on a shaker, the resulting solution was stored at 4°C. mRNA-B9-hFc was mixed with sodium acetate buffer; then, the mixed organic phase and RNA-sodium acetate solution were each added to two inlets of INano L Nanoformulation Equipment (Micro&Nano) at a volume ratio of 3:1. The flow rate of LNP preparation was controlled at approximately 12 mL/min; the collected RNA- LNPs were dispersed in PBS after ultrafiltration. The products were diluted 1:10 in PBS, and the Z-average particle size and polydispersity index were determined by Zetasizer Nano ZS (Spectris) in triplicate. The products were diluted or concentrated to a concentration of 1 μg/µL and stored at 4°C for subsequent in vivo experiments.
In vivo mRNA expression
The animal experimentation protocols, compliant with the guidelines of the National Institute of Biological Sciences, were approved by the Animal Ethics Committee of the Beijing Institute of Biotechnology (No.IACUC-DWZX-2023-037).
Female KM mice (an outbred group of mice derived from Swiss mice) (weight = 18–20 g) (SPF (Beijing)Biotechnology Co., Ltd., Beijing, China) were randomly divided into three groups (n = 4), which were intravenously administered 100 μg of mRNA-B9-hFc-LNPs, blank LNPs (negative control), or B9-hFc (positive control). Mouse serum samples were collected at 2, 6, 12, 24, 48, 72, and 96 h after administration; the supernatants were centrifuged at 3,000 g and 4°C and then stored at − 80°C until further analysis. Serum antibody concentrations were determined through ELISA.
Challenge experiments
In total, 48 KM female mice (weight = 18–20 g) (SPF (Beijing)Biotechnology Co., Ltd., Beijing, China) were divided into four experimental, four positive control, and four negative control groups (n = 4); they were administered a single intravenous injection of mRNA-B9-hFc (100 µg per mouse), B9-hFc (40 µg per mouse), and standard saline, respectively. BoNT/B was diluted in dilution buffer (50 mM KH2PO4, 50 mM Na2HPO4, 1 M NaCl, 1% gelatin, pH 6.5). At 1, 3, 5, and 7 days after mRNA administration, the mice were intraperitoneally injected with 100 LD50 BoNT/B (500 µL)(the National Institute for Food and Drug Control, Beijing, China). Accordingly, positive control mice received i.p. injections of 100 LD50 of BoNT/B at intervals of 0, 2, 4, or 6 days post-administration. Over the 7 days, the mice were evaluated once daily.
To verify the correlation between BoNT/B intoxication prevention and B9-hFc concentration, the mouse serum samples were collected before the challenge and stored at − 80°C for further analysis. Serum B9-hFc concentrations were measured through ELISA as described above.
Higher doses of BoNT/B, respectively 1000 LD50, 5000 LD50, 10000 LD50, or 50,000 LD50, were intraperitoneally injected into mice either 1 day after intravenous administration of 100 µg of mRNA-B9-hFc or 2 hours after injection of 40 µg of B9-hFc. This was done to determine the dose of toxin at which B9-hFc and mRNA encoding B9-hFc no longer exhibited a protective effect. The mice were evaluated once daily for 7 days.
Result
Design and synthesis of mRNA-encoded antibodies
On the basis of the structure of heavy-chain antibody and mRNA, we designed and constructed a B9-hFc-encoding transcription template, containing the T7 promoter, 5′ untranslated region (UTR), antibody-coding region [open reading frame (ORF)], and 3′UTR (). The mRNA was capped at the 5′ end with a natural Cap1 structure, added using Vaccinia Capping Enzyme and Cap 2′-O-Methyltransferase. Moreover, a poly-A tail was added at the 3′ end by using Escherichia coli poly-A polymerase. The capillary electrophoresis results () demonstrated that mRNA-B9-hFc – obtained through in vitro transcription, nucleoside modification, 5′ capping, and 3′ tailing – demonstrated a normal band size, high sample purity, and a single and clear peak pattern, indicating a satisfactory quality control effect.
In vitro expression of mRNA-encoded antibodies
To confirm that mRNA-B9-hFc provided efficient protein translation, we transfected it into HEK-293F, COS-7, and CHO cells. Cell culture supernatants were obtained 48 h after transfection. The initial concentration of recombinant B9-hFc was 0.25 μg/ml, and the antibody-antigen binding curve () was plotted using gradient concentration antibody ELISA. The linear interval obtained (OD495nm/595 nm value ranging from 0.1397 to 1.0527) was considered as the standard curve for quantifying the antibody concentration in the supernatant. The results () demonstrated that mRNA-B9-hFc was well expressed in all three cell lines; the concentrations of B9-hFc were 1070.376, 414.268, and 1425.704 ng/mL in the supernatant of HEK-293F, COS-7, and CHO cells, respectively.
Figure 2. In vitro expression of mRNA-B9-hFc. (a) BHc binding activity of the antibodies by ELISA. The concentration of the antigen was 2 μg/mL, while the initial concentration of recombinant B9-hFc protein was set at 0.25 μg/mL. (b) B9-hFc concentrations in mRNA-transfected cell lines. Determine the antibody concentration in the cell supernatant by using the standard curve and the OD450nm/595 nm value of the sample well.

In vivo expression of mRNA-encoded antibodies
Next, we explored whether mRNA-B9-hFc efficiently and persistently expressed the target proteins in mice. In vitro synthesized mRNA-B9-hFc, was encapsulated with lipid nanoparticles (LNPs) for delivery and expression in mice. This is currently the most commonly used technique for mRNA delivery to humans.Citation20 Because LNPs are highly effective in delivering nucleic acid drugs in vivo, two mRNA-loaded LNP (mRNA-LNP) vaccines for neocoronavirus infections have been approved for use in humans.Citation21,Citation22 In addition to the negatively charged mRNA, mRNA-LNPs typically contain four components: ionizable lipids, neutral auxiliary phospholipids, cholesterol, and polyethylene glycol-modified lipids (i.e., PEGylated lipids). The mRNAs in aqueous solution are encapsulated using ionizable cationic lipids, which form nanoparticles with other lipid components (mPEG-DMG-2K and cholesterol), with an encapsulation efficiency of approximately 89% and an effective concentration of 825 μg/mL ().
Figure 3. In vivo expression of mRNA-B9-hFc. (a) mRNA-B9-hFc-LNPs particle size analysis results. The schematic in the top left corner of the figure represents its complex structure. (b) Curve of time versus plasma concentrations of mRNA-encoded antibody. The blue line represents the expression level of the mRNA-encoded B9-hFc, while the beige line represents the level of the B9-hFc.

KM mice were injected intravenously with 100 μg of mRNA-B9-hFc-LNPs or B9-hFc; the postadministration dose – response relationship for B9-hFc was determined through the analysis of mouse sera with BHc-specific ELISA. The ELISA results () demonstrated that at 24 h after administration, the concentration of mRNA-expressed B9-hFc protein in serum peaked, at 21.476 μg/mL; this concentration decreased gradually over the next few days. In general, our mRNA-B9-hFc-LNPs enabled rapid, efficient neutralizing antibody expression in vivo.
Prophylaxis with mRNA-encoded B9-hFc in mice
To evaluate the in vivo efficacy of mRNA-B9-hFc, experimental mice were given prophylactic treatment with 100 μg mRNA-B9-hFc-LNPs or 40 μg recombinant B9-hFc (positive control, 24 hour post mRNA administration). Here the dose was determined according to , in which the total serum mass of B9-hFc in mRNA-B9-hFc group was approxi-mately 40 μg (to multiply 20 μg/ml by 2 ml). The four groups of mRNA-B9-hFc-LNPs treated mice were intraperitoneally challenged with 100 LD50 of BoNT/B at 1, 3, 5, or 7 days post-administration separately, and the parallel positive control mice were challenged at 0, 2, 4, or 6 days post-administration accordingly. As the result showed ( and Table S1), all mice received mRNA-B9-hFc-LNPs or recombinant B9-hFc survived through seven days, whereas untreated mice died within 2–3 h of toxin administration (the recorded time is 1 day). The results demonstrate that mRNA-B9-hFc can be effectively expressed in vivo, offering protection against low levels of BoNT exposure. Additionally, we measured prechallenge serum antibody concentrations. As illustrated in , a single intravenous injection of mRNA-B9-hFc-LNPs, which demonstrated continuous and stable B9-hFc expression in mice, enabled maintenance of the serum B9-hFc concentration of >20 µg/mL until postadministration day 7, with a relatively slow degradation rate. Compared with the result of , the serum antibody concentrations displayed higher levels after mRNA-B9-hFc administration. This may be a result of the batch differences caused by the mRNA non-industrial preparation system.
Figure 4. Prophylactic protection provided by the mRNA-encoded B9-hFc against toxin challenges. (a) Protective effects of 100 μg of mRNA-B9-hFc-LNPs against 100 LD50 of BoNT/B in mice (n = 4). The x-axis represents the observation time after exposure of mice to BoNT/B. Mice per group were monitored for survival over 7d. (b) Concentrations of B9-hFc at various timepoints after injection with a 100-μg dose of mRNA-B9-hFc-LNPs or 40-μg of B9-hFc in treated mouse serum. The left graph shows the concentration of mRNA-encoded B9-hFc. The right graph shows the concentration of the recombinant B9-hFc protein.
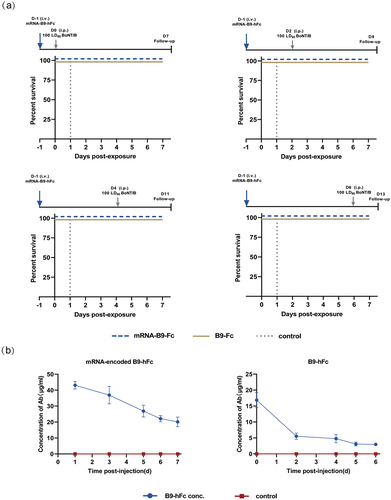
Furthermore, prophylactic treated mice were also challenged with 1,000 LD50, 5,000 LD50, 10,000 LD50, or 50,000 LD50 of BoNT/B at 1 day (mRNA-B9-hFc-LNPs) or 2 hours (B9-hFc) post administration. As the result showed ( and Table S2), mice that received mRNA-B9-hFc-LNPs experienced one fatality when the toxin dose reached 10000 LD50, and half of the mice succumbed at a dose of 50,000 LD50. In contrast, all mice received recombinant B9-hFc had perished by the time the toxin dose reached 5000 LD50. All control mice receiving standard saline died.
Discussion
In the current study, we obtained candidate mRNA molecules that effectively encoded B9-hFc (a previously obtained heavy-chain antibody fused to camel-derived VHH and Fc region of human antibody) and noted that it protected mice from high-dose BoNT/B challenge. The mRNA molecules, termed mRNA-B9-hFc-LNPs, somewhat prolonged in vivo antibody duration, potentially broadening the applicability of neutralizing antibodies in botulism prevention.
Generally, VHH displays a short half-life which limits its application in human as therapeutic agents.Citation9,Citation16 In our study, we fused VHH (nanobody) to Fc region of human antibody to construct a chimeric heavy chain antibody. Accord-ing to our result (), the fusion with Fc region, whether B9-hFc or mRNA-B9-hFc, apparently prolonged the dura-tion of VHH in mice serum, which resulted in a long period protection against BoNT/B challenge (). It was evident that the concentration of mRNA-encoded antibody was more persistent and stable in mice compared to recombinant protein, which showed significant attenuation within days 1–2 (). However, it was worth noting that the inter-batch consistency of mRNA prepared at the laboratory level wasn’t sufficiently high. The in vivo expression of the samples used for the mouse protective experimental batches exceeded predictions (). Meanwhile, it can be observed from the results () that the expression efficiency of mRNA-B9-hFc in mice is not high enough, suggesting that the optimization in regulatory elements and codons of mRNA is possible. In vivo expression of mRNA-B9-hFc () showed that mRNA-encoded antibodies reach a serum concentration of approximately 5 μg/ml within 2–6 hours post-injection, achieving 100% protection against 100 LD50 BoNT/B at this time (). This also suggests that the application of mRNA in post-exposure treatment could be more promising when the expression of the mRNA encoding antibodies is optimized in vivo to peak within a very short period of time.
The strategy of constructing broad-spectrum neutralizing antibodies by linking six VHHs against three botulinum neurotoxin serotypes has been reported, and it has demonstrated excellent functional activity.Citation16 Currently, our laboratory is also conducting research on novel nanobodies targeting other serotypes of BoNT. In the future, we plan to utilize antibody engineering techniques to fuse VHHs capable of neutralizing different serotypes of BoNTs at the amino and carboxyl ends of the Fc, constructing broad-spectrum neutralizing antibodies, and exploring more efficient strategies for BoNT prevention and treatment.
In addition, several recent studies have focused on the application of antibody-encoding RNA molecules, such as including circular and self-amplifying RNAs.Citation23,Citation24 Prepared through the cyclization reaction in vitro, circular RNAs are less prone to degradation and more durable target protein expression. Self-amplifying RNAs mimic RNA viruses by allowing in vitro synthesis of RNAs that replicate within cells, achieving more sustained protein expression. These RNA technology platforms may enable the development of mRNA drugs encoding neutralizing antibodies for botulism prevention and treatment and improve their clinical effectiveness.
Author contributions
Conceptualization, P.D., J.L and G.Q.; methodology, G.Q., Y.J. and G.L.; software, G.Q.; formal analysis, G.Q., P.D. and J.L.; investigation, G.Q. and Y.J.; resources, G.Q. and G.L.; data curation, G.Q.; writ-ing – original draft preparation, G.Q., Y.J. and J.L.; writing – review and editing, Y.C., P.D., G.Q., J.L., Z.Y., F.L. and Y.Y.; supervision, P.D., Y.C., J.L., Z.Y.and F.L.; project administration, Y.C., P.D., J.L. and G.Q. All authors have read and agreed to the published version of the manuscript.
Supplemental Material
Download PDF (151.9 KB)Supplemental Material
Download PDF (161.1 KB)Acknowledgments
We thank Dr. Xiaofan Zhao (Beijing Institute of Biotechnology) for support of the LNPs coating technique.
Disclosure statement
No potential conflict of interest was reported by the author(s).
Data availability statement
The datasets generated during and/or analyzed during the current study are available from the corresponding author on reasonable request.
Supplementary material
Supplemental data for this article can be accessed on the publisher’s website at https://doi.org/10.1080/21645515.2024.2358570
Additional information
Funding
References
- Popoff MR, Bouvet P. Genetic characteristics of toxigenic Clostridia and toxin gene evolution. Toxicon 2013;75:63–8. doi:10.1016/j.toxicon.2013.05.003.
- Hill KK, Smith TJ. Genetic diversity within Clostridium botulinum serotypes, botulinum neurotoxin gene clusters and toxin subtypes. Curr Top Microbiol Immunol. 2013;364:1–20. doi:10.1007/978-3-642-33570-9_1.
- Pirazzini M, Rossetto O, Eleopra R, Montecucco C. Botulinum neurotoxins: biology, pharmacology, and toxicology. Pharmacol Rev. 2017;69(2):200–35. doi:10.1124/pr.116.012658.
- Rossetto O, Montecucco C. Tables of toxicity of botulinum and tetanus neurotoxins. Toxins 2019;11(12):686. doi:10.3390/toxins11120686.
- Dutta SR, Passi D, Singh M, Singh P, Sharma S, Sharma A. Botulinum toxin the poison that heals: a brief review. Natl J Maxillofac Surg. 2016;7(1):10–16. doi:10.4103/0975-5950.196133.
- Johnson EA, Montecucco C:. Botulism. Handb Clin Neurol. 2008;91:333–68. doi:10.1016/s0072-9752(07)01511-4.
- Cherington M. Clinical spectrum of botulism. Muscle Nerve. 1998;21(6):701–10. doi:10.1002/(sici)1097-4598(199806)21:6<701:aid-mus1>3.0.co;2-b.
- Godakova SA, Noskov AN, Vinogradova ID, Ugriumova GA, Solovyev AI, Esmagambetov IB, Tukhvatulin AI, Logunov DY, Naroditsky BS, Shcheblyakov DV. et al. Camelid VHHs fused to human Fc fragments provide long term protection against botulinum neurotoxin a in mice. Toxins 2019;11(8):464. doi:10.3390/toxins11080464.
- Thran M, Mukherjee J, Pönisch M, Fiedler K, Thess A, Mui BL, Hope MJ, Tam YK, Horscroft N, Heidenreich R. et al. mRNA mediates passive vaccination against infectious agents, toxins, and tumors. EMBO Mol Med. 2017;9(10):1434–47. doi:10.15252/emmm.201707678.
- Dezfulian M, Hatheway CL, Yolken RH, Bartlett JG. Enzyme-linked immunosorbent assay for detection of Clostridium botulinum type a and type B toxins in stool samples of infants with botulism. J Clin Microbiol. 1984;20(3):379–83. doi:10.1128/jcm.20.3.379-383.1984.
- Tacket CO, Shandera WX, Mann JM, Hargrett NT. Blake PA: equine antitoxin use and other factors that predict outcome in type a foodborne botulism. Am J Med. 1984;76(5):794–8. doi:10.1016/0002-9343(84)90988-4.
- Wolff JA, Malone RW, Williams P, Chong W, Acsadi G, Jani A, Felgner PL. Direct gene transfer into mouse muscle in vivo. Science 1990;247(4949 Pt 1):1465–8. doi:10.1126/science.1690918.
- Fiolet T, Kherabi Y, MacDonald CJ, Ghosn J, Peiffer-Smadja N. Comparing COVID-19 vaccines for their characteristics, efficacy and effectiveness against SARS-CoV-2 and variants of concern: a narrative review. Clin Microbiol Infect. 2022;28(2):202–21. doi:10.1016/j.cmi.2021.10.005.
- Schlake T, Thran M, Fiedler K, Heidenreich R, Petsch B, Fotin-Mleczek M. mRNA: a novel avenue to antibody therapy? Mol Ther. 2019;27(4):773–84. doi:10.1016/j.ymthe.2019.03.002.
- Karikó K. In vitro-transcribed mRNA therapeutics: out of the shadows and into the spotlight. Mol Ther. 2019;27(4):691–2. doi:10.1016/j.ymthe.2019.03.009.
- Mukherjee J, Ondeck CA, Tremblay JM, Archer J, Debatis M, Foss A, Awata J, Erasmus JH, McNutt PM, Shoemaker CB. Intramuscular delivery of formulated RNA encoding six linked nanobodies is highly protective for exposures to three botulinum neurotoxin serotypes. Sci Rep. 2022;12(1):11664. doi:10.1038/s41598-022-15876-2.
- Jiang Y, Wang R, Guo J, Cheng K, Chen L, Wang X, Li Y, Du P, Gao C, Lu J. Isolation and characterization of Hc-targeting chimeric heavy chain antibodies neutralizing botulinum neurotoxin type B. Front Immunol. 2024;15:1380694. doi:10.3389/fimmu.2024.1380694.
- Sample PJ, Wang B, Reid DW, Presnyak V, McFadyen IJ, Morris DR, Seelig G. Human 5′ UTR design and variant effect prediction from a massively parallel translation assay. Nat Biotechnol. 2019;37(7):803–9. doi:10.1038/s41587-019-0164-5.
- Jackson LA, Anderson EJ, Rouphael NG, Roberts PC, Makhene M, Coler RN, McCullough MP, Chappell JD, Denison MR, Stevens LJ. et al. An mRNA vaccine against SARS-CoV-2 — preliminary report. N Engl J Med. 2020;383(20):1920–31. doi:10.1056/NEJMoa2022483.
- Paunovska K, Loughrey D, Dahlman JE. Drug delivery systems for RNA therapeutics. Nat Rev Genet. 2022;23(5):265–80. doi:10.1038/s41576-021-00439-4.
- Chaudhary N, Weissman D, Whitehead KA. mRNA vaccines for infectious diseases: principles, delivery and clinical translation. Nat Rev Drug Discov. 2021;20(11):817–38. doi:10.1038/s41573-021-00283-5.
- Alfagih IM, Aldosari B, AlQuadeib B, Almurshedi A, Alfagih MM. Nanoparticles as adjuvants and nanodelivery systems for mRNA-based vaccines. Pharmaceutics 2020;13(1):45. doi:10.3390/pharmaceutics13010045.
- Wesselhoeft RA, Kowalski PS, Anderson DG. Engineering circular RNA for potent and stable translation in eukaryotic cells. Nat Commun. 2018;9(1):2629. doi:10.1038/s41467-018-05096-6.
- Erasmus JH, Archer J, Fuerte-Stone J, Khandhar AP, Voigt E, Granger B, Bombardi RG, Govero J, Tan Q, Durnell LA. et al. Intramuscular delivery of replicon RNA encoding ZIKV-117 human monoclonal antibody protects against Zika virus infection. Mol Ther Methods Clin Dev. 2020;18:402–14. doi:10.1016/j.omtm.2020.06.011.