ABSTRACT
Mucosal immunity plays a crucial role in combating and controlling the spread of highly mutated severe acute respiratory syndrome coronavirus 2 (SARS-CoV-2). Recombinant subunit vaccines have shown safety and efficacy in clinical trials, but further investigation is necessary to evaluate their feasibility as mucosal vaccines. This study developed a SARS-CoV-2 mucosal vaccine using spike (S) proteins from a prototype strain and the omicron variant, along with a cationic chitosan adjuvant, and systematically evaluated its immunogenicity after both primary and booster immunization in mice. Primary immunization through intraperitoneal and intranasal administration of the S protein elicited cross-reactive antibodies against prototype strains, as well as delta and omicron variants, with particularly strong effects observed after mucosal vaccination. In the context of booster immunization following primary immunization with inactivated vaccines, the omicron-based S protein mucosal vaccine resulted in a broader and more robust neutralizing antibody response in both serum and respiratory mucosa compared to the prototype vaccine, enhancing protection against different variants. These findings indicate that mucosal vaccination with the S protein has the potential to trigger a broader and stronger antibody response during primary and booster immunization, making it a promising strategy against respiratory pathogens.
Introduction
Severe acute respiratory syndrome coronavirus 2 (SARS-CoV-2) is a highly contagious virus that poses a serious threat to public health. The rapid development of traditional inactivated vaccines and universal immunization has significantly reduced the severity and mortality of SARS-CoV-2 infection.Citation1 However, over time post-vaccination and with the emergence of variants like the delta and omicron strains, there has been a observed decline in the levels of cross-reactive neutralizing antibodies generated by inactivated vaccines.Citation2,Citation3 In particular, the omicron variant has an unusually large number of mutations, which could potentially facilitate immune evasion in hosts and increased transmission rates.Citation4,Citation5 Therefore, there is a pressing demand for the development of more efficient vaccines and immunization strategies to control SARS-CoV-2 and prevent breakthrough infections caused by vaccine-resistant variants.Citation6
SARS-CoV-2 initially infects the host through the nasal cavity and pharyngeal mucosa.Citation7 The period before the infection progresses to the lower respiratory tract and leads to lung infection represents the ideal window to achieve optimal efficacy with preventive vaccines.Citation8 Nasal mucosal vaccination, which simulates natural viral infection, has the potential to trigger the production of immunoglobulin A (IgA) and immunoglobulin G (IgG) antibodies in the respiratory tract and stimulate cellular immune responses, thereby aiding in the establishment of immune protection as the primary line of defense against viral invasion.Citation9,Citation10 Additionally, mucosal vaccination enhances public compliance and streamlines large-scale vaccination campaigns.Citation11 Hence, developing mucosal immunity in the upper respiratory tract emerges as a promising approach for managing SARS-CoV-2.Citation12
Most current mucosal vaccines against SARS-CoV-2, both clinically approved and under development, are viral vector vaccines (e.g., vaccines with adenovirus or live attenuated influenza virus vectors).Citation13,Citation14 These vaccines have exhibited the potential to stimulate both systemic and mucosal immune responses,Citation15 effectively reducing viral replication and shedding in the respiratory tract and altering the immune microenvironment.Citation16,Citation17 Recently, two viral-vectored mucosal vaccines have been approved as booster doses in India (intranasal iNCOVACC developed by Bharat Biotech International Limited in Hyderabad, India)Citation18 and China (orally inhaled Convidecia Air developed by CanSino Biologics Inc. in Tianjin, China).Citation19 However, concerns remain about preexisting immunity, virulence reversion, and other potential risks.Citation20 An intranasal SARS-CoV-2 mRNA vaccine, with an additional cationic lipid, induced strong binding and neutralizing antibody responses while inhibiting viral replication in the respiratory tract, opening up new avenues for possibilities for using traditional vaccine development platforms for intranasal vaccination.Citation21 Recombinant subunit vaccines like NVX-CoV2373 and ZF2001 have been demonstrated to be safe and effective,Citation22,Citation23 potentially addressing the limitations of viral vector vaccines. Nonetheless, more research is needed to determine their effectiveness as mucosal vaccines.
Chitosan, a biodegradable natural polymer, has been reported to possess properties similar to cationic lipids, which can enhance the penetration and absorption of drugs through the nasal mucosa while also delaying mucociliary clearance.Citation24,Citation25 In this study, cationic chitosan was utilized as a mucosal adjuvant to enhance the local adhesion and retention of the full-length spike (S) protein antigen. The objective of this approach was to assess the immunogenicity and efficacy of a mucosal S protein vaccine in both primary and booster immunization in mice, as well as to investigate the feasibility of this combination as a vaccine booster following two doses of inactivated vaccines.
Materials and methods
Cells, viruses, proteins and animals
HEK-293T cells stably expressing human ACE2 (293T-ACE2) were graciously provided by the Division of HIV/AIDS and Sex-transmitted Virus Vaccines, Institute for Biological Product Control, National Institutes for Food and Drug Control (NIFDC) of China, and selected using 15 μg/mL blasticidin S. The 293T-ACE2 cells were cultured in Dulbecco’s modified Eagle’s medium (DMEM; Gibco, USA) supplemented with 10% fetal bovine serum (FBS; Gibco), 15 μg/mL blasticidin S, 20 mM HEPES, and 1% penicillin/streptomycin. This medium was designated cDMEM. SARS-CoV-2-luciferase pseudovirus (including prototype Wuhan-1 strain, delta B.1.617.2 variant, and omicron BA.1 variant) was procured from Beijing SanYao Science & Technology Co., Ltd. Additionally, SARS-CoV-2 S proteins and bulk inactivated virus strains (prototype, delta, and omicron) were graciously provided by Sinovac Biotech Ltd. (Beijing, China). Six- to eight-week-old female specific pathogen-free BALB/c mice (weighing 18–20 g) were provided and raised by the Experimental Animal Center of the NIFDC of China. All animal experiments were conducted following approval from the Animal Ethical and Welfare Committee of the NIFDC of China (approval number 2022B033).
Animal immunization
For primary immunization, mice were immunized intranasally with either 30 µg of S protein (prototype S protein [i.n.]) or inactivated vaccine (prototype bulk [i.n.]) twice at 2-week intervals, and the immunization dose was 30 μg/30 μL. Chitosan (Invitrogen, USA) was used as a mucosal adjuvant at a concentration of 1% (w/v). Additionally, intraperitoneal immunization groups received aluminum hydroxide adjuvant at a dose of 30 μg/100 μL. Serum samples were collected on day 14 (before the second immunization) and day 28 to measure specific IgG antibodies. Nasal lavage fluid (NLF) and bronchoalveolar lavage fluid (BALF) were collected on day 28 to determine specific IgA antibody levels.
For booster immunization, mice were preinoculated with two doses of the prototype inactivated vaccine, followed by intranasal booster immunization with the S protein or intraperitoneal immunization with the inactivated vaccine three months later. Mice injected with PBS for booster immunization were designated as the control group. Serum and lavage fluid samples were collected on day 14 after booster immunization.
Enzyme-linked immunosorbent assay (ELISA)
Specific antibody titers were measured using indirect ELISA. Briefly, ELISA plates were coated with SARS-CoV-2 S protein at a concentration of 1 μg mL−1 in PBS and incubated at 4°C overnight. After five washes with PBST (0.05% Tween-20 in PBS), the plates were blocked with 200 μL of blocking solution (1% bovine serum albumin in PBS) and then incubated at 37°C for 2 h. The standard serum utilized in this study was laboratory-prepared mouse serum with established antibody titers (as determined via ELISA and quantification by Karber`s method). The diluted serum and lavage fluid samples were incubated with primary antibodies for 1 h at 37°C, followed by incubation with either an HRP-conjugated goat anti-mouse IgG antibody (Cytiva, China) or an HRP-conjugated goat anti-mouse IgA antibody (BETHYL, USA) as the secondary antibody for 1 h at 37°C. After five washes with PBST, the chromogenic TMB substrate solution (Seracare, USA) was added and incubated at room temperature in the dark for 15 min. The reaction was then stopped by the addition of H2SO4 (1 N). Optical density was measured at 450/630 nm using a SpectraMax ABS Plus microplate reader (Molecular Devices, USA). The OD values and antibody titers of the standards were fitted to a four-parameter curve using SoftMax software to calculate the antibody titers of the samples.
Pseudovirus-based neutralization assay
The titers of neutralizing antibodies against SARS-CoV-2 were evaluated through a pseudovirus-based neutralization assay. The pseudovirus was diluted in cDMEM to a concentration of 1000 × TCID50/50 μL. The samples were twofold serially diluted in cDMEM in a 96-well plate to a final volume of 50 μL per well and further preincubated with 50 μL of pseudovirus at 37°C for 1 h. The detection limits for neutralizing antibody assays were set at a serum dilution of 1:8 and a lavage fluid dilution of 1:4. 293T-ACE2 cells (5 × 104 cells per well/100 μL) were then added to the sample/virus mixture and incubated at 37°C for 18–24 h. The cell control group containing only cells and the virus control group containing virus and a tenfold dilution of negative control serum were set up at the same time. Subsequently, the supernatant was removed, and 100 μL of luciferase assay reagent (Promega, USA) was added to each well. The plate was gently shaken at room temperature in the dark for 2 min, and the relative light unit (RLU) value was measured using a GloMax Navigator luminometer (Promega). The pseudovirus inhibition rate was calculated as follows: (RLU sample well − RLU cell control well)/(RLU virus control well − RLU cell control well) × 100%. The neutralizing antibody titer was defined as the maximum sample dilution corresponding to a virus inhibition rate of less than 50%.
Statistical analysis
Statistical analysis and graphical representations were performed using GraphPad Prism 8 software (GraphPad Software Inc., USA). The values in all the graphs are presented as the geometric mean (GMT) and geometric standard deviation (SD). The statistical significance of differences between groups was evaluated by one-way ANOVA with Tukey’s post hoc test or the Kruskal‒Wallis rank-sum test with post-hoc Dunn’s pairwise comparison test (ns, not significant; *p < .05, **p < .01, ***p < .001).
Results
Intranasal immunization with a prototype SARS-CoV-2 S protein vaccine induced high levels of IgG and IgA antibody responses in mice
To assess the mucosal immunogenicity of the S protein vaccine in vivo, mice were immunized intranasally (i.n.) or intraperitoneally (i.p.) with 30 µg of prototype S protein (prototype S protein [i.n.] or prototype S protein [i.p.]) or inactivated prototype bulk vaccine (prototype bulk [i.n.] or prototype bulk [i.p.]) twice at 2-week intervals. Serum samples were collected on day 14 (before the second immunization) and day 28 to measure S protein-specific IgG antibodies. Additionally, S protein-specific IgA antibodies were detected in the lavage fluid samples obtained on day 28. Both the S protein and the inactivated viral antigen elicited strong S protein-specific serum IgG antibody responses after the first and second immunizations, regardless of the route of administration (), indicating activation of antigen-specific humoral immunity. Notably, intranasal immunization with the S protein elicited high levels of specific IgG responses, demonstrating the potential for nasal administration of the protein. At the mucosal level, intranasal vaccination with the S protein and inactivated viral antigen effectively induced the production of S protein-specific IgA in both NLF and BALF, with the IgA antibody titer induced in NLF being more than 10 times higher than that induced by intraperitoneal immunization (). Interestingly, intraperitoneal immunization with inactivated viral antigen also resulted in high levels of IgA antibodies in the lungs (GMT = 104), showing no significant differences compared to the levels induced by intranasal immunization with the S protein vaccine.
Figure 1. Antibody responses induced by the prototype SARS-CoV-2 vaccine in mice. a. S protein-specific IgG titers in serum samples collected after the first dose and the second dose (n = 10). b–c. S protein-specific IgA titers in NLF (b) and BALF (c) collected after the second dose (n = 10). The endpoint IgA titer of naïve animals was approximately 50.
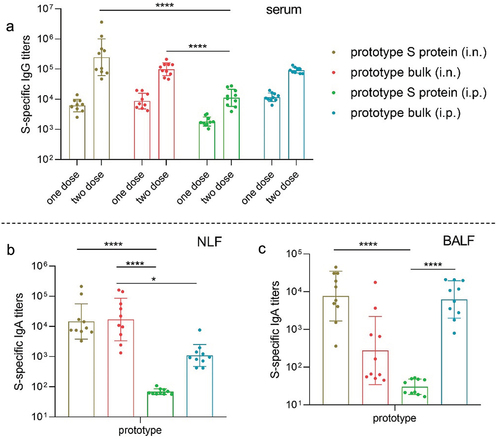
We subsequently evaluated cross-reactive antibody responses to SARS-CoV-2 variants in both serum and lavage fluid. Intranasal administration of the prototype S protein and inactivated viral antigen induced similarly high levels of cross-reactive IgG antibodies to the S protein of the delta and omicron variants in the serum (). Compared to intraperitoneal immunization, intranasal immunization with the S protein elicited stronger IgG antibody responses to the delta and omicron variants, consistent with previous observations for the prototype strain. The antibody response to both the prototype strain and the delta and omicron variants indicates the potential superiority of the S protein in mucosal immunization. Moreover, cross-reactive IgA antibody responses to the delta and omicron variants were observed in NLF and BALF, especially in NLF (). The data in this section demonstrated that intranasal immunization with the prototype S protein induces high levels of cross-reactive IgA antibodies in the respiratory tract and stimulates systemic immune responses by inducing the generation of serum antibodies.
Figure 2. The prototype SARS-CoV-2 vaccine induced cross-reactive antibody responses in mice. a. Delta and omicron S protein-specific IgG titers in serum samples collected after the first dose (n = 10). b. Delta and omicron S protein-specific IgG titers in serum samples collected after the second dose (n = 10). c–d. Delta and omicron S protein-specific IgA titers in NLF (c) and BALF (d) collected after the second dose (n = 10).
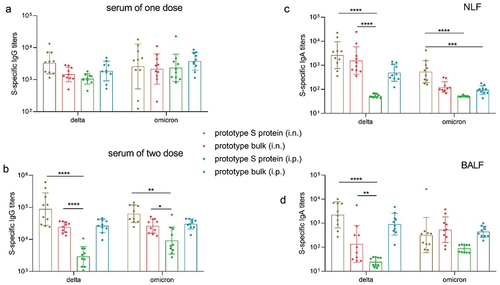
The use of prototype S protein-based mucosal vaccines as boosters after priming vaccination with inactivated vaccines elicited robust humoral and mucosal immunity
The intranasal S protein vaccine induced cross-reactive antibody responses during primary immunization, but its immunogenicity after inactivated vaccine administration still needed further investigation. From the perspectives of humoral immunity, we systematically compared the differences in immune responses induced by a third booster immunization with intraperitoneal inactivated vaccine or intranasal S protein vaccine after basic immunization with the inactivated vaccine. Mice received a booster immunization with either intranasal S protein or intraperitoneal inactivated vaccine three months after the initial two doses of the inactivated vaccine, at which point, antibody levels started to naturally decline. Serum and lavage fluid samples were collected on the 14th day following booster immunization.
The serum S protein-specific IgG antibody titer increased by 6.3-fold after booster intranasal immunization, while that of the nonbooster control decreased 0.7-fold. The IgA antibody titer in the respiratory lavage fluid also significantly increased, with a striking 189-fold increase in NLF. Although booster intraperitoneal immunization increased S protein-specific antibody titers in the serum and respiratory lavage fluid, the total antibody levels were significantly lower than those resulting from booster intranasal immunization (). These findings suggest that mucosal vaccines act as vaccine boosters in the context of inactivated vaccines, providing benefits for eliciting systemic IgG antibodies in the serum and mucosal IgA antibodies in the respiratory tract.
Figure 3. Using the prototype SARS-CoV-2 vaccine as a booster induced S protein-specific IgG and IgA antibody responses. a. S protein-specific IgG titers in serum samples collected before and after booster immunization (n = 10). b. S protein-specific IgA titers in NLF and BALF collected after booster immunization (n = 10). The pre-boost endpoint titer of S-specific IgA response was approximately 50.
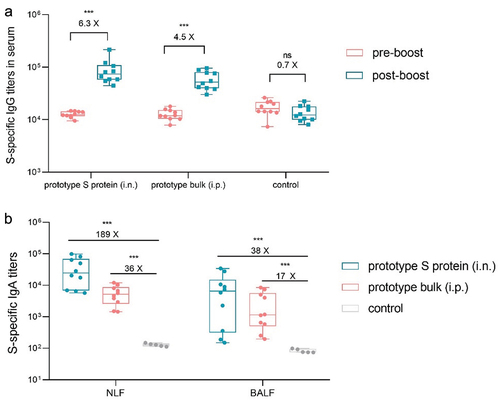
Subsequently, we tested cross-reactive antibody responses after booster vaccination. As shown in , the serum titers of IgG antibodies against the delta and omicron variants after intranasal immunization increased by 3.3-fold and 2.2-fold, respectively, while these titers after intraperitoneal immunization increased by 2.6-fold and 1.6-fold, respectively. Additionally, intranasal immunization resulted in increased IgA levels in both NLF and BALF (). We also observed that the extent of the increase in the titers of IgG and IgA antibodies against the delta and omicron variants induced by booster immunization was limited compared to that of the prototype strain. This may be associated with the utilization of the prototype S protein as an antigen for booster immunization.
Figure 4. Using the prototype SARS-CoV-2 vaccine as a booster induced cross-reactive antibody responses in mice. a–b. Delta (a) and omicron (b) S protein-specific IgG titers in serum samples collected before and after booster immunization (n = 10). c–d. Delta and omicron S protein-specific IgA titers in NLF (c) and BALF (d) collected after booster immunization (n = 10).
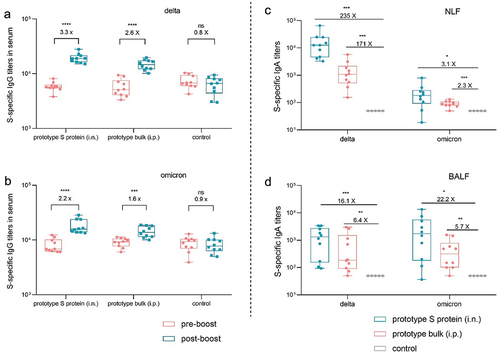
The presence of neutralizing antibodies is a crucial indicator of vaccine efficacy. To further evaluate the effect of mucosal immunity on booster vaccination, we next evaluated the levels of neutralizing antibodies against the prototype, delta and omicron variant pseudoviruses in the serum and lavage fluid. As shown in , booster immunization with intranasal S protein and intraperitoneal inactivated virus markedly increased the levels of neutralizing antibodies against the three pseudoviruses in the serum, with a notable increase in neutralizing antibody activity against the omicron variant. Similarly, booster immunization substantially increased neutralizing antibody activity against the prototype and delta pseudoviruses in both NLF and BALF, especially intranasal immunization. However, in terms of neutralizing antibody activity against the omicron variant, the two immunization routes did not show a significant difference, and both resulted in relatively weak activity. These data demonstrated that booster immunization with the prototype S protein or inactivated virus produced a robust strain-specific antibody response with potent neutralizing activity against the delta variant but limited cross-reactive immunity to the omicron variant. These findings underscore the challenges posed by the immune escape ability of the omicron variant and emphasize the critical need for more effective vaccination strategies.
Figure 5. Using the prototype SARS-CoV-2 vaccine as a booster induced cross-reactive neutralizing antibodies in mice. a–c. Neutralizing antibodies against the prototype (a), delta (b), and omicron (c) strains in serum samples collected before and after booster immunization (n = 10). d–f. Neutralizing antibodies against the prototype (d), delta (e), and omicron (f) strains in NLF and BALF collected after booster immunization (n = 10). The dotted curve represents the limit of detection.
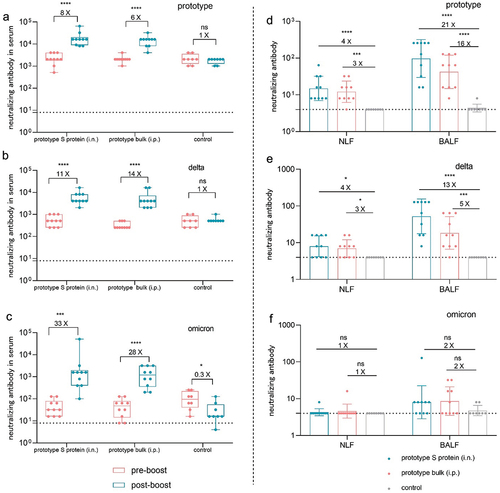
Using an omicron S protein-based mucosal vaccine as a booster after priming vaccination with an inactivated prototype SARS-CoV-2 vaccine elicited broad-spectrum humoral and mucosal immunity
The intranasal prototype S protein vaccine efficiently triggered both humoral and mucosal immune responses, but only limited production of cross-reactive antibodies against the omicron variant. To address this, we investigated the effectiveness of an intranasal omicron S protein vaccine in stimulating robust antibody production against the omicron variant. After receiving two doses of the prototype inactivated vaccine, the mice were immunized intranasally with 30 µg of omicron S protein or intraperitoneally with 30 µg of inactivated omicron bulk vaccine. Serum and lavage fluid samples were collected on the 14th day after booster immunization.
In contrast to the antibody levels in the control group, which continuously decreased after booster immunization, the levels of IgG antibodies against the prototype, delta and omicron variants in mouse serum significantly increased following booster immunization with mucosal omicron S protein vaccine and omicron inactivated vaccine, with mucosal omicron S protein vaccine exerting particularly strong effects. Similarly, booster immunization with mucosal omicron S protein vaccine significantly increased IgA antibody titers against these variants in both NLF and BALF compared with those in the control group, with a substantial increase of greater than tenfold and peak titers higher than those in the group administered intraperitoneal inactivated vaccine. While the mucosal omicron S protein vaccine induced a superior mucosal immune response, the specific IgA antibody response to the omicron variant in NLF was only 4.2-fold stronger than that in the control group (). Nonetheless, this response was still stronger than that observed with the mucosal prototype S protein vaccine, highlighting the potential of the omicron S protein in inducing cross-reactive antibody responses.
Figure 6. Using the omicron SARS-CoV-2 vaccine as a booster induced cross-reactive antibody responses in mice. a. Prototype, delta, and omicron S protein-specific IgG titers in serum samples collected before and after booster immunization (n = 10). b–c. Prototype, delta, and omicron S protein-specific IgA titers in NLF (b) and BALF (c) collected after booster immunization (n = 10).
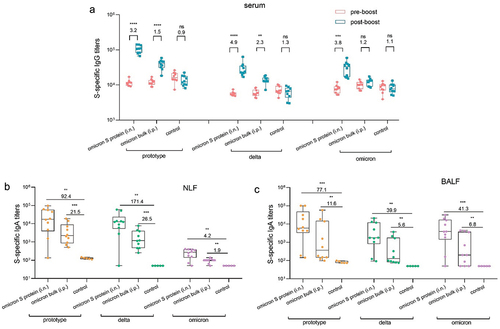
Subsequently, neutralizing antibodies against the prototype, delta, and omicron variant pseudoviruses were evaluated in serum and lavage fluid. As shown in , compared with two doses of the inactivated vaccine, booster immunization with mucosal omicron S protein vaccine or the omicron inactivated vaccine significantly increased the titers of neutralizing antibodies against the prototype, delta, and omicron pseudoviruses in mouse serum. In particular, following the administration of a booster immunization with mucosal omicron S protein vaccine, the titer of neutralizing antibodies increased by 81-fold compared to the prebooster immunization level. Moreover, both types of booster immunizations significantly increased the levels of neutralizing antibodies against the prototype and delta pseudoviruses in NLF and BALF (), consistent with nasal immunization with the prototype S protein. Additionally, the mucosal omicron S protein vaccine slightly enhanced the levels of neutralizing antibodies against the omicron variant, with effects superior to those of nasal immunization with the prototype S protein. As such, optimizing the antigen, delivery vector, and adjuvant may be essential for improving neutralization efficacy against the omicron variant and providing sufficient protection against severe illness caused by the omicron variant. The data presented in this section demonstrated that use of the mucosal omicron S protein-based vaccine as a booster after priming vaccination with inactivated prototype SARS-CoV-2 vaccine elicited broad-spectrum humoral and mucosal immune responses against prototype, delta, and omicron variants in both serum and lavage fluid.
Figure 7. Using omicron SARS-CoV-2 vaccine as a booster induced neutralizing antibodies in mice. (a) Neutralizing antibodies against prototype, delta, and omicron strains in serum samples collected before and after booster immunization (n = 10). b–c. Neutralizing antibodies against prototype, delta, and omicron strains in NLF (b) and BALF (c) collected after booster immunization (n = 10). The dotted curve represents the limit of detection.
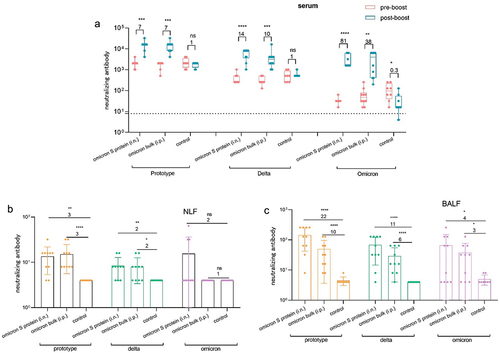
Discussion
Mucosal immunity plays a crucial role in combating and controlling the spread of SARS-CoV-2, especially in the development and application of mucosal vaccines.Citation26 This study utilized prototype and omicron S proteins as antigens, along with cationic chitosan as a mucosal adjuvant, to investigate the systemic and mucosal immunogenicity induced by different vaccine candidates and vaccination regimens. This approach sets the groundwork for utilizing mucosal vaccines in both primary and booster immunization against SARS-CoV-2.
The results of this study indicated that during the primary immunization phase, compared to injection of a vaccine containing only S protein components, injection of the bulk inactivated prototype or omicron virus containing the complete viral proteome resulted in stimulation of a stronger IgG antibody response in the serum. This enhanced response may be attributed to the synergistic ability of nucleoproteins or other conserved proteins in whole virus particles to boost the effect of the S protein antigen, as supported by previous research findings.Citation27 In the context of booster immunization following primary immunization with inactivated vaccines, both mucosal and injectable vaccines significantly increased the neutralizing antibody activity against the prototype, delta, and omicron strains in the serum. This enhanced activity which may be linked to the immunological memory established by primary immunization with the prototype strain vaccine. Notably, nasal immunization with the S protein elicited a broader immune response against different variants in NLF and BALF. This may be attributed to the fact that intranasal vaccination mimics pathogen entry, directly engaging the mucosal immune system in the respiratory tract where IgA is the primary antibody isotype. By targeting the mucosal-associated lymphoid tissue, this administration route provides essential protection at the entry point of pathogens and facilitates the development of robust and enduring immune memory.Citation28–30
The omicron variant, characterized by significant amino acid sequence variation, compromises the protective efficacy of antibodies elicited by some prototype vaccines.Citation31,Citation32 This study found that using an inactivated vaccine or a mucosal omicron S protein vaccine as a booster immunization resulted in lower levels of neutralizing antibodies against the omicron variant in both the respiratory tract and serum of mice compared to using a prototype strain or the delta variant. This finding suggests the potential impact of mutated epitopes on the immunogenicity of the S protein of the omicron variant. Nevertheless, in the current context of primary immunization with prototype SARS-CoV-2 vaccines,Citation33 booster immunization with a mucosal omicron-based S protein vaccine and an inactivated vaccine increased neutralizing antibody activity against omicron strains when compared to the prototype vaccine, especially the intranasal S protein vaccine. Therefore, booster immunization with a mucosal omicron-based vaccine is likely to enhance the immune response, broaden and extend the protective effects of the vaccine, and enhance its effectiveness. Importantly, effective mucosal immunization requires the use of potent antigens, adjuvants, delivery systems, and other strategies to elicit adequate protective immunity.Citation34
The activation of humoral and mucosal immunity via intranasal delivery of the S protein could offer a unique advantage in the development of SARS-CoV-2 vaccines.Citation35 By targeting the mucosal immune system in the respiratory tract, a primary entry point for this virus, intranasal vaccines can stimulate a localized immune response at the infection site.Citation36,Citation37 Humoral immunity, which involves antibody production, is essential for neutralizing the virus and preventing its spread within the body.Citation38 Additionally, the potential activation of cellular immunity by intranasal vaccines could be beneficial in the development of SARS-CoV-2 vaccines, although this aspect was not explored in this study and is absent in inactivated vaccines.Citation39
Conclusions
When administered in the context of primary and booster immunization, intranasal SARS-CoV-2 S protein vaccines, particularly the omicron S protein vaccine, have the potential to induce broader and more robust immune responses in both the serum and respiratory mucosa. This reveals a promising strategy for combatting respiratory tract infections caused by highly mutated SARS-CoV-2. We aim to enhance the design and components of mucosal vaccines to simultaneously achieve robust local mucosal immunity and protective systemic cellular and humoral immunity, thereby broadening the scope and efficacy of SARS-CoV-2 vaccines in the future.
Authors contributions
H.Y., C.L., and Y.Q. designed the study, supervised the whole project and revised the manuscript. Y.X. performed the experiments and statistical analysis. J.N. wrote the manuscript. S.L., C.B. and J.W. participated in the experimental performance and revised the manuscript. All authors read and approved the final manuscript.
Author information
Huijie Yang and Ying Xie are co-first authors.
Availability of data and materials
The data and materials that support the findings of this study are available from the corresponding author upon reasonable request.
Ethics approval and consent to participate
All procedures for animal experiments were reviewed and approved by the Animal Ethical and Welfare Committee of the NIFDC of China (approval number 2022B033).
Disclosure statement
No potential conflict of interest was reported by the author(s).
Additional information
Funding
References
- Huang Z, Xu S, Liu J, Wu L, Qiu J, Wang N, Ren J, Li Z, Guo X, Tao F. et al. Effectiveness of inactivated and Ad5-nCoV COVID-19 vaccines against SARS-CoV-2 Omicron BA. 2 variant infection, severe illness, and death. BMC Med. 2022;20(1):400. doi:10.1186/s12916-022-02606-8.
- Yu X, Qi X, Cao Y, Li P, Lu L, Wang P, Feng Y, Yang J, Wei H, Guo L. et al. Three doses of an inactivation-based COVID-19 vaccine induces cross-neutralizing immunity against the SARS CoV-2 Omicron variant. Emerg Microbes Infect. 2022;11(1):749–10. doi:10.1080/22221751.2022.2044271.
- Schultz BM, Melo-Gonzalez F, Duarte LF, Galvez NMS, Pacheco GA, Soto JA, Berrios-Rojas RV, Gonzalez LA, Moreno-Tapia D, Rivera-Perez D. et al. A booster dose of coronavac increases neutralizing antibodies and T cells that recognize delta and omicron variants of concern. mBio. 2022;13(4):e0142322. doi:10.1128/mbio.01423-22.
- Hoffmann M, Kruger N, Schulz S, Cossmann A, Rocha C, Kempf A, Nehlmeier I, Graichen L, Moldenhauer AS, Winkler MS. et al. The Omicron variant is highly resistant against antibody-mediated neutralization: implications for control of the COVID-19 pandemic. Cell. 2022;185(3):447–56 e411. doi:10.1016/j.cell.2021.12.032.
- Servellita V, Syed AM, Morris MK, Brazer N, Saldhi P, Garcia-Knight M, Sreekumar B, Khalid MM, Ciling A, Chen PY. et al. Neutralizing immunity in vaccine breakthrough infections from the SARS-CoV-2 Omicron and Delta variants. Cell. 2022;185(9):1539–48.e5. doi:10.1016/j.cell.2022.03.019.
- Zhao MM, Zhu Y, Zhang L, Zhong G, Tai L, Liu S, Yin G, Lu J, He Q, Li MJ. et al. Novel cleavage sites identified in SARS-CoV-2 spike protein reveal mechanism for cathepsin L-facilitated viral infection and treatment strategies. Cell Discov. 2022;8(1):53. doi:10.1038/s41421-022-00419-w.
- Gilbert C, Lefeuvre C, Preisser L, Pivert A, Soleti R, Blanchard S, Delneste Y, Ducancelle A, Couez D, Jeannin P. Age-related expression of IFN-λ1 versus IFN-I and beta-defensins in the nasopharynx of SARS-CoV-2-infected individuals. Front Immunol. 2021;12:750279. doi:10.3389/fimmu.2021.750279.
- Zarkoob H, Allue-Guardia A, Chen YC, Garcia-Vilanova A, Jung O, Coon S, Song MJ, Park JG, Oladunni F, Miller J. et al. Modeling SARS-CoV-2 and influenza infections and antiviral treatments in human lung epithelial tissue equivalents. Commun Biol. 2022;5(1):810. doi:10.1038/s42003-022-03753-7.
- Nie J, Zhou Y, Ding F, Liu X, Yao X, Xu L, Chang Y, Li Z, Wang Q, Zhan L. et al. Self-adjuvant multiepitope nanovaccine based on ferritin induced long-lasting and effective mucosal immunity against H3N2 and H1N1 viruses in mice. Int J Biol Macromol. 2024;259(Pt 1):129259. doi:10.1016/j.ijbiomac.2024.129259.
- Liu S, Stauft CB, Selvaraj P, Chandrasekaran P, D’Agnillo F, Chou CK, Wu WW, Lien CZ, Meseda CA, Pedro CL. et al. Intranasal delivery of a rationally attenuated SARS-CoV-2 is immunogenic and protective in Syrian hamsters. Nat Commun. 2022;13(1):6792. doi:10.1038/s41467-022-34571-4.
- Nantachit N, Sunintaboon P, Ubol S. Responses of primary human nasal epithelial cells to EDIII-DENV stimulation: the first step to intranasal dengue vaccination. Virol J. 2016;13(1):142. doi:10.1186/s12985-016-0598-z.
- Hassan AO, Kafai NM, Dmitriev IP, Fox JM, Smith BK, Harvey IB, Chen RE, Winkler ES, Wessel AW, Case JB. et al. A single-dose intranasal ChAd vaccine protects upper and lower respiratory tracts against SARS-CoV-2. Cell. 2020;183(1):169–84.e13. doi:10.1016/j.cell.2020.08.026.
- Wang Q, Yang C, Yin L, Sun J, Wang W, Li H, Zhang Z, Chen S, Liu B, Liu Z. et al. Intranasal booster using an Omicron vaccine confers broad mucosal and systemic immunity against SARS-CoV-2 variants. Signal Transduct Target Ther. 2023;8(1):167. doi:10.1038/s41392-023-01423-6.
- Deng S, Liu Y, Tam RC, Chen P, Zhang AJ, Mok BW, Long T, Kukic A, Zhou R, Xu H. et al. An intranasal influenza virus-vectored vaccine prevents SARS-CoV-2 replication in respiratory tissues of mice and hamsters. Nat Commun. 2023;14(1):2081. doi:10.1038/s41467-023-37697-1.
- Li JX, Wu SP, Guo XL, Tang R, Huang BY, Chen XQ, Chen Y, Hou LH, Liu JX, Zhong J. et al. Safety and immunogenicity of heterologous boost immunisation with an orally administered aerosolised Ad5-nCoV after two-dose priming with an inactivated SARS-CoV-2 vaccine in Chinese adults: a randomised, open-label, single-centre trial. Lancet Respir Med. 2022;10(8):739–48. doi:10.1016/S2213-2600(22)00087-X.
- van Doremalen N, Purushotham JN, Schulz JE, Holbrook MG, Bushmaker T, Carmody A, Port JR, Yinda CK, Okumura A, Saturday G. et al. Intranasal ChAdOx1 nCoV-19/AZD1222 vaccination reduces viral shedding after SARS-CoV-2 D614G challenge in preclinical models. Sci Transl Med. 2021;13(607):eabh0755. doi:10.1126/scitranslmed.abh0755.
- Zhang L, Jiang Y, He J, Chen J, Qi R, Yuan L, Shao T, Zhao H, Chen C, Chen Y. et al. Intranasal influenza-vectored COVID-19 vaccine restrains the SARS-CoV-2 inflammatory response in hamsters. Nat Commun. 2023;14(1):4117. doi:10.1038/s41467-023-39560-9.
- Bharat Biotech International Limited: BBV154 – a novel adenovirus vectored, intranasal vaccine for COVID-19. 2022. https://www.bharatbiotech.com/intranasal-vaccine.html.
- CanSino Biologics Inc.: CanSinoBIO’s convidecia Air™ receives approval in China. 2022. www.cansinotech.com/html/1/179/180/1100.html.
- Li JQ, Zhang ZR, Zhang HQ, Zhang YN, Zeng XY, Zhang QY, Deng CL, Li XD, Zhang B, Ye HQ. Intranasal delivery of replicating mRNA encoding neutralizing antibody against SARS-CoV-2 infection in mice. Signal Transduct Target Ther. 2021;6(1):369. doi:10.1038/s41392-021-00783-1.
- Baldeon Vaca G, Meyer M, Cadete A, Hsiao CJ, Golding A, Jeon A, Jacquinet E, Azcue E, Guan CM, Sanchez-Felix X. et al. Intranasal mRNA-LNP vaccination protects hamsters from SARS-CoV-2 infection. Sci Adv. 2023;9(38):eadh1655. doi:10.1126/sciadv.adh1655.
- Heath PT, Galiza EP, Baxter DN, Boffito M, Browne D, Burns F, Chadwick DR, Clark R, Cosgrove C, Galloway J. et al. Safety and efficacy of NVX-CoV2373 Covid-19 vaccine. N Engl J Med. 2021;385(13):1172–83. doi:10.1056/NEJMoa2107659.
- Dai L, Gao L, Tao L, Hadinegoro SR, Erkin M, Ying Z, He P, Girsang RT, Vergara H, Akram J. et al. Efficacy and safety of the RBD-Dimer-Based COVID-19 vaccine ZF2001 in adults. N Engl J Med. 2022;386(22):2097–111. doi:10.1056/NEJMoa2202261.
- Guo H, Li F, Xu W, Chen J, Hou Y, Wang C, Ding J, Chen X. Mucoadhesive cationic polypeptide nanogel with enhanced penetration for efficient intravesical chemotherapy of bladder cancer. Adv Sci (Weinh). 2018;5(6):1800004. doi:10.1002/advs.201800004.
- Li Y, Wang C, Sun Z, Xiao J, Yan X, Chen Y, Yu J, Wu Y. Simultaneous intramuscular and intranasal administration of chitosan nanoparticles-adjuvanted Chlamydia vaccine elicits elevated protective responses in the lung. Int J Nanomed. 2019;14:8179–93. doi:10.2147/IJN.S218456.
- Wu S, Zhong G, Zhang J, Shuai L, Zhang Z, Wen Z, Wang B, Zhao Z, Song X, Chen Y. et al. A single dose of an adenovirus-vectored vaccine provides protection against SARS-CoV-2 challenge. Nat Commun. 2020;11(1):4081. doi:10.1038/s41467-020-17972-1.
- Yang H, Xie Y, Lu S, Sun Y, Wang K, Li S, Wang J, Liao G, Li C. Independent protection and influence of the spike-specific antibody response of SARS-CoV-2 nucleocapsid protein (N) in Whole-Virion vaccines. Vaccines (Basel). 2023;11(11):1681. doi:10.3390/vaccines11111681.
- Sterlin D, Mathian A, Miyara M, Mohr A, Anna F, Claer L, Quentric P, Fadlallah J, Devilliers H, Ghillani P. et al. IgA dominates the early neutralizing antibody response to SARS-CoV-2. Sci Transl Med. 2021;13(577):eabd2223. doi:10.1126/scitranslmed.abd2223.
- Larson CL, Baker RE, Baker MB, Smith DM. Delayed hypersensitivity induced in guinea pigs with tuberculoprotein from M. bovis (BCG). Proc Soc Exp Biol Med. 1969;131(1):100–4. doi:10.3181/00379727-131-33813.
- Kuzin AM, Vagabova ME. Synergism of the effect of gamma radiation and radiotoxins. 1. Effect of radiotoxins on the growth of native and irradiated corn rootlets. Radiobiologiia. 1981;21(1):117–19.
- Lu L, Mok BWY, Chen LL, Chan JMC, Tsang OTY, Lam BHS, Chuang VWM, Chu AWH, Chan WM, Ip JD. et al. Neutralization of severe acute respiratory syndrome coronavirus 2 omicron variant by Sera from BNT162b2 or CoronaVac vaccine recipients. Clin Infect Dis. 2022;75(1):822–6. doi:10.1093/cid/ciab1041.
- Bartsch YC, Tong X, Kang J, Avendano MJ, Serrano EF, Garcia-Salum T, Pardo-Roa C, Riquelme A, Cai Y, Renzi I. et al. Omicron variant Spike-specific antibody binding and Fc activity are preserved in recipients of mRNA or inactivated COVID-19 vaccines. Sci Transl Med. 2022;14(642):eabn9243. doi:10.1126/scitranslmed.abn9243.
- Hu L, Xu Y, Wu L, Feng J, Zhang L, Tang Y, Zhao X, Mai R, Chen L, Mei L. et al. The E484K substitution in a SARS-CoV-2 Spike protein subunit vaccine resulted in limited cross-reactive neutralizing antibody responses in mice. Viruses. 2022;14(5):854. doi:10.3390/v14050854.
- Sen Chaudhuri A, Yeh YW, Zewdie O, Li NS, Sun JB, Jin T, Wei B, Holmgren J, Xiang Z. S100A4 exerts robust mucosal adjuvant activity for co-administered antigens in mice. Mucosal Immunol. 2022;15(5):1028–39. doi:10.1038/s41385-022-00535-6.
- Cao H, Mai J, Zhou Z, Li Z, Duan R, Watt J, Chen Z, Bandara RA, Li M, Ahn SK. et al. Intranasal HD-Ad vaccine protects the upper and lower respiratory tracts of hACE2 mice against SARS-CoV-2. Cell Biosci. 2021;11(1):202. doi:10.1186/s13578-021-00723-0.
- Tioni MF, Jordan R, Pena AS, Garg A, Wu D, Phan SI, Weiss CM, Cheng X, Greenhouse J, Orekov T. et al. Mucosal administration of a live attenuated recombinant COVID-19 vaccine protects nonhuman primates from SARS-CoV-2. NPJ Vaccines. 2022;7(1):85. doi:10.1038/s41541-022-00509-6.
- Lara-Puente JH, Carreno JM, Sun W, Suarez-Martinez A, Ramirez-Martinez L, Quezada-Monroy F, Paz-De la Rosa G, Vigueras-Moreno R, Singh G, Rojas-Martinez O. et al. Safety and immunogenicity of a newcastle disease virus vector-based SARS-CoV-2 vaccine candidate, AVX/COVID-12-HEXAPRO (Patria), in Pigs. mBio. 2021;12(5):e0190821. doi:10.1128/mBio.01908-21.
- Ikegame S, Siddiquey MNA, Hung CT, Haas G, Brambilla L, Oguntuyo KY, Kowdle S, Chiu HP, Stevens CS, Vilardo AE. et al. Neutralizing activity of Sputnik V vaccine sera against SARS-CoV-2 variants. Nat Commun. 2021;12(1):4598. doi:10.1038/s41467-021-24909-9.
- Luan N, Wang Y, Cao H, Lin K, Liu C. Comparison of immune responses induced by two or three doses of an alum-adjuvanted inactivated SARS-CoV-2 vaccine in mice. J Med Virol. 2022;94(5):2250–8. doi:10.1002/jmv.27637.