ABSTRACT
The brain is one of the most vulnerable organs inside the human body. Head accidents often appear in daily life and are easy to cause different level of brain damage inside the skull. Once the brain suffered intense locomotive impact, external injuries, falls, or other accidents, it will result in different degrees of concussion. This study employs finite element analysis to compare the dynamic characteristics between the geometric models of an assumed simple brain tissue and a brain tissue with complex cerebral sulci. It is aimed to understand the free vibration of the internal brain tissue and then to protect the brain from injury caused by external influences. Reverse engineering method is used for a Classic 5-Part Brain (C18) model produced by 3B Scientific Corporation. 3D optical scanner is employed to scan the human brain structure model with complex cerebral sulci and imported into 3D graphics software to construct a solid brain model to simulate the real complex brain tissue. Obtaining the normal mode analysis by inputting the material properties of the true human brain into finite element analysis software, and then to compare the simplified and the complex of brain models.
Introduction
Traffic accidental fatalities have increased each year and around 58% of these deaths are due to head injuries. The research papers regarding the dynamics analysis of human head slowly began to develop in recent years. Therefore, it is worthwhile to explore the characteristic of human brain as well. Zhu.Citation1 and Lin.Citation2 both used the finite element (FE) analysis to investigate the mechanism of the formation of brain contusion. They explained 2 main theories of occurrence of brain contusion, one was high negative pressure generated vortex erosion inducing brain damage; another was unsmooth between the skull and brain limiting the relative motion and producing high shear strain and damage. Analysis results illustrated that shear strain theory seemed better able to explain mechanism of brain contusion caused by the impact of brain or hindbrain. Huang et al.Citation3 used 3-dimensional (3D) finite element analysis to study the cerebral contusion. The model was first validated against a set of experimental results from the literature. The result showed that shear strain theory appeared to better account for the clinical findings that most cerebral contusions in the absence of skull fracture occurred at the frontal and temporal lobes. Kleiven and HardyCitation4 modeled the brain material properties by using hyperelastic and viscoelastic constitutive law. They showed that a range of shear stiffness properties for the brain could be used to model the pressure experiments, while relative motion was a more complex measure that was highly sensitive to the brain tissue properties. The results suggested that significantly lower values of the shear properties of the human brain than currently used in most 3D FE models were needed to predict the localized brain response of an impact to the human head. Sayed et al.Citation5 used FE model to explore traumatic brain injury and soft tissue damage by frontal and oblique head impacts with external objects. The dynamic behavior of the brain and cerebrospinal fluid were studied according to external injuries. Ho and KleivenCitation6 constructed a detailed 3D FE model and a smoothed brain surface model based on a Magnetic Resonance imaging (MRI) scan of a human head. The validated FE models were subjected to several acceleration impulses and the maximum principle strain and strain rate in the brain were analyzed. The results suggested that the inclusion of sulci indeed alters the strain and strain distribution in an FE model. WeiCitation7 adopted Pro/ENGINEER software to combine 3 individual geometry models of the skull (validated with experiments), simplified brain and simplified Cerebrospinal Fluid (CSF). Both free vibrations and impact force analyses were studied to obtain the frequency spectrum, modal and displacement responses at different impact time duration and in different locations. The displacements on different locations of brain model with CSF were lower than that of the brain model without CSF after external impacts. Rashid et al.Citation8 obtained the material parameters via unconfined compression tests and stress relaxation tests. It would help to develop biofidelic human brain finite element models, which could subsequently be used to predict brain injuries under impact conditions. Post et al.Citation9 used the University College Dublin Brain Trauma Model (UCDBTM) to analyze 3 distinct loading curve shapes meant to represent different helmet loading scenarios. The loading curves were applied independently in each axis of linear and angular acceleration and their effect on currently used predictors of Traumatic Brain Injury (TBI) and Mild Traumatic Brain Injury (mTBI) was examined.
In order to find the dynamic behavior of real human brain, this study focused on the modal characteristics of internal entity of the head, i.e., brain tissue. The analysis of complex brain model (a brain tissue with complex cerebral sulci) is compared with the simple brain tissue. In order to understand the variation of each mode, this study is to analyze the natural vibration modes of human brain tissue. Once the frequency spectrum of the brain tissue is obtained, it is easy to design the operational speed of a motorcycle or to develop a motorcycle helmet to avoid those natural frequencies, and to protect human brain better. The analysis results can be referenced for automobile industrial and other future research study.
Finite element analysis
This study refers to New Practical Anatomy and PhysiologyCitation10 to understand internal structure of the brain tissue (). Through reverse engineering method, the scale of one to one Classic 5-Part Brain (C18) models (includes brain, cerebellum, brain stem, complex cerebral sulci) (), manufactured by 3B Scientific Corporation, are scanned by 3D optical scanner. Geomagic Studio and 3D SolidWorks are employed to repair and smooth the defect areas, irregular surface, and then mirror of the graphs generated by the scanner. This process is extremely tedious and time consuming. Any existing tine hole and discontinuity will cause mesh error in ANSYS software. The improved and assembled solid models of both simplified and complex brain tissue are established. The assembled 3D graph is then imported into ANSYS software to analyze the dynamic behavior of the brain tissue.
Figure 1. Brain structure diagram.Citation10
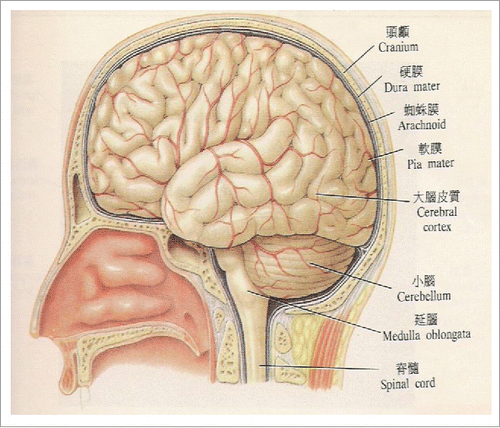
In order to obtain the accurate data of the human brain, 2 different set of natural frequencies for the simple and complex model of the brain tissue are analyzed and compared. Element Solid187 is chosen for both brain models. This type of element is suitable for irregular grids and can reduce the poor accuracy problems caused by free mesh. ANSYS intelligent meshing technology has strengthen element cutting capability and generates smooth meshes for both simple and complex types of brain tissues ( and ), respectively.
Real human material coefficients of Young's modulus, Poisson's ratio and densityCitation1,2,3,7 are selected for analysis and shown in . The boundary conditions for both simple and complex models are set completely fixed in X, Y, Z directions at the cross section of the junction of the brain and brain stem, respectively. Element size, shape and coarseness may affect the accuracy and correctness of analytical results. The convergence tests are proceeded to validate the number of elements for each model separately before normal analysis. Finite element analysis and convergence validation process are shown in . The results include natural vibration frequency, amplitude, intensity and displacement of the brain tissue models.
Table 1. Material properties of the human brain.Citation1,2,3,7
Results and discussion
The present study is to investigate a simple brain tissue and a more realistic complex model with cerebral sulci. 3D optical scanner, Geomagic Studio, 3D SolidWorks, ANSYS software, and material parameters of real human brain are used to build the geometric models and to run the analysis. The dynamic analysis is divided into 2 parts in order to discuss and to compare a few fundamental natural vibration frequencies and corresponding mode shapes. “Convergence test” in the finite element analysis is necessary to study. The finite element results will be changed by the element number meshed of the system in this finite element analysis. If the element number meshed is enough or more, the results will keep an almost constant value. It is so-called “Convergence test.” In the analysis, only this convergence result can be employed to accept and study.Citation11
Modal analysis of a simple brain tissue
The meshed model of the simple brain is imported by material parameters of real human brain. As mentioned above, after series of convergence tests, the converged first natural frequency is 1.21 Hz when element number in finite element analysis is equal to and greater than 43,000. Therefore, 43,000 elements are used for structural analysis of the simple brain model. In nature, the any physical system definitely has the infinite numbers of natural frequency, which lead to this system damage when the external exciton frequency close or equal to these natural frequency. So, to avoid the brain damage, the natural frequencies of this brain must be studied. However, in real applications, only the lower natural frequencies of human brain are frequently excited by the external excitations, such as an impact or shock. Therefore, only the lower 6 natural frequencies and corresponding modes are considered to study in this work. In order to understand the vibration mode detail of natural frequency, both 2 different direction views are employed to display in the same natural frequency.
The first natural frequency of the simple brain model is 1.21 Hz and the corresponding mode shape is a swing type of vibration in left and right direction, as shown in . Referenced maximum displacement, 60.3 mm, occurred at the front end slightly above the brain tissue. The second natural frequency of the simple brain model is 1.27 Hz and the corresponding mode shape is also a swing type of vibration in front and rear direction, as shown in . Referenced maximum displacement, 58.1 mm, occurred at the front end of the brain tissue. The third natural frequency of the simple brain model is 2.0 Hz and the corresponding mode shape is a spinning back and forth around the stem axis. Referenced maximum displacement, 62.0 mm, occurred at back of the brain tissue. The forth natural frequency of the simple brain model is 10.34 Hz and the corresponding mode shape is a combination of expansion up and down parallel to brain stem and a swing type of vibration in front and rear direction. Referenced maximum displacement, 64.0 mm, occurred at front end of the brain tissue. The fifth natural frequency of the simple brain model is 13.76 Hz and the corresponding mode shape is a swing type of vibration in left and right direction with nearly zero displacement at the top of the brain and bottom of the brain stem. Referenced maximum displacement, 62.1 mm, occurred at outer sides, bottom of the cerebellum position. The sixth natural frequency of the simple brain model is 16.45 Hz and the corresponding mode shape is similar to forth mode but the referenced maximum displacement occurred at top rear of the brain tissue. The natural frequencies can be found in the first column of comparison table as shown in . The total deformation of the modes range from 0 to around 60 mm is represented by color gradient from blue to red, accordingly.
Figure 6. First mode shape of a simple brain model: a swing type of vibration in left and right direction.
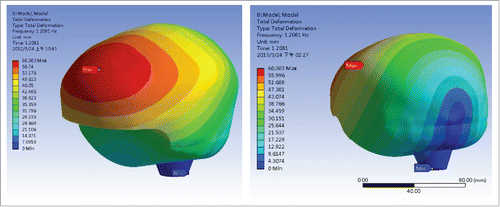
Figure 7. Second mode shape of a simple brain model: a swing type of vibration in front and rear direction.
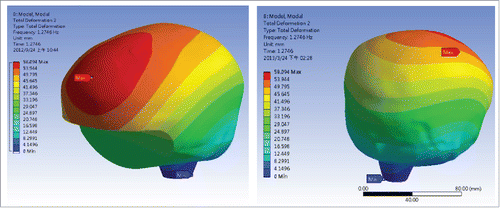
Table 2. Comparison of natural frequencies between simple and complex brain models.
Modal analysis of a brain tissue with complex cerebral sulci
The meshed model of a brain tissue with complex cerebral sulci is also imported by material parameters of real human brain. Again, after series of convergence tests, the converged first natural frequency is 1.87 Hz when element number is equal to and greater than 85,000. Hence, 85,000 elements are used for structural analysis of the complex brain model. The first natural frequency of the complex brain model is 1.87 Hz and the corresponding mode shape is a swing type of vibration in front and rear direction (similar to the second mode shape of simple brain model), as shown in . Referenced maximum displacement, 68.4 mm, occurred at the front end of the brain tissue. The second natural frequency of the simple brain model is 2.74 Hz and the corresponding mode shape is a swing type of vibration in left and right direction (similar to the 1st mode shape of simple brain model), as shown in . Referenced maximum displacement, 65.1 mm, occurred at the front end slightly above the brain tissue. The third natural frequency of the simple brain model is 3.23 Hz and the corresponding mode shape is a spinning back and forth around the stem axis. Referenced maximum displacement, 67.9 mm, occurred at front of the brain tissue. The forth natural frequency of the simple brain model is 11.99 Hz and the corresponding mode shape is a combination of expansion up and down parallel to brain stem and a swing type of vibration in front and rear direction. Referenced maximum displacement, 58.5 mm, occurred at top end of the brain tissue. The fifth natural frequency of the simple brain model is 13.51 Hz and the corresponding mode shape is a swing type of vibration in left and right direction with nearly zero displacement at the top of the brain and bottom of the brain stem. Referenced maximum displacement, 67.5 mm, occurred at outer sides, bottom of the cerebellum position. The sixth natural frequency of the simple brain model is 14.57 Hz and the corresponding mode shape is similar to forth mode but the referenced maximum displacement occurred at front end of the brain tissue. The natural frequencies can be found in the second column of the comparison table as shown in . The total deformation of the modes range from 0 to around 60 mm is represented by color gradient from blue to red, accordingly.
Figure 8. First mode shape of a complex brain model: a swing type of vibration in front and rear direction.
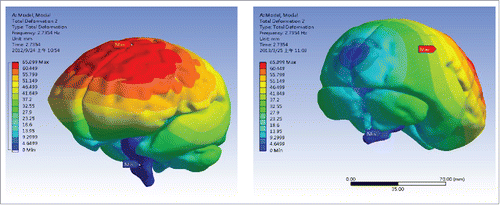
Figure 9. The second mode shape of a complex brain model: a swing type of vibration in right and left direction.
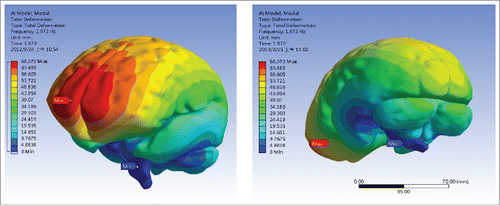
This study compares 2 sets of 6 fundament natural frequencies for simple and complex models of brain tissue, respectively, as shown in . From the table, 6 pairs of the natural frequencies are closed to each other. The actual value of the maximum error does not exceed more than 2Hz. Although the percentage errors in the list of mode 1 to mode 3 are between 35% and 53%, the other 3 higher modes, mode 4 to mode 6, confine their errors to less than 15%. These results indicate that the pairs of natural frequencies of 2 different geometric structures are closed to each other due to same human brain material coefficients and boundary conditions adopted. Furthermore, the appearance of the surface of the brain tissue with or without complex cerebral sulci does not affect the natural frequency and deformation much. In other words, by using finite element analysis, the researchers can have a quick solution to save a lot of effort to draw a simple model instead of a complex one.
However, both simple and complex models will be used for future impact analysis to obtain the dynamic characteristics of the brain tissue.
Summary
In this study, 2 different modeling skills are used to represent the brain structures, a simple brain tissue and a brain tissue with brain, cerebellum, brain stem, and complex cerebral sulci. Finite element analysis simulations are proceeded to identify the dynamic characteristics and relationship simple and complex brain tissues. Modal analyses are performed with same human brain material constants and boundary conditions. Pairs of natural frequencies from mode 1 to mode 6 of the 2 models are compared. Several interesting point can be pointed out from the above analysis as follows:
Six pairs of lower natural frequencies of 2 different geometric structures, simple and complex brain tissues, are closed to each other, due to same human brain material coefficients and boundary conditions adopted.
From normal mode analysis, the appearance of the surface of the brain tissue with or without complex cerebral sulci does not affect the natural frequency and deformation much.
By using finite element analysis, the researchers can have a quick solution to save a lot of effort to draw a simple model instead of a complex one.
Disclosure of potential conflicts of interest
No potential conflicts of interest were disclosed.
Funding
This research was financially supported by the National Science Foundation, Taiwan, R.O.C. NSC99-2632-E-230-001-MY3.
References
- Zhu XS. Finite element analysis of brain contusion [master's thesis]. [Taipei, Taiwan]: National Yang-Ming University; 1990; 128 p.
- Lin M-X. Finite element analysis of brain contusion (II) [master's thesis]. [Taipei, Taiwan, R.O.C.]: National Yang-Ming University; 1991; 110 p.
- Huang HW, Lee MC, Lee SY, Chiu WT, Pan LC, Chiu CT. Finite element analysis of brain contusion: an indirect impact study. Med Biol Eng Comput 2000; 38(3):253-59; PMID:10912340; http://dx.doi.org/10.1007/BF02347044
- Kleiven S, Hardy WN. Correlation of an FE model of the human head with local brain motion consequences for injury prediction. Stapp Car Crash J 2002; 46:123-44; PMID:17096222
- Sayed TE, Mota A, Fraternali F, Ortiz M. Biomechanics of traumatic brain injury. Comput Method Appl M 2008; 197(51–52):4692-701; http://dx.doi.org/10.1016/j.cma.2008.06.006
- Ho J, Kleiven S. Can sulci protect the brain from traumatic injury?. J Biomech 2009; 42:2074-80; PMID:19679308; http://dx.doi.org/10.1016/j.jbiomech.2009.06.051
- Wei C-W. An investigation on the dynamic properties of a human brain with impulse, [master's thesis]. Kaohsiung, Taiwan, R.O.C.: Cheng Shiu University, 2011; 93 p.
- Rashid B, Destrade M, Gilchrist MD. Mechanical characterization of brain tissue in compression at dynamic strain rates. J Mech Behav Biomed 2012; 10:23-38; http://dx.doi.org/10.1016/j.jmbbm.2012.01.022
- Post A, Hoshizaki B, Gilchrist MD. Finite element analysis of the effect of loading curve shape on brain injury predictors. J Biomech 2012; 45(4):679-83; PMID:22239921; http://dx.doi.org/10.1016/j.jbiomech.2011.12.005
- Xu S. New Practical Anatomy and Physiology. Taipei, Taiwan: Yeong Dah Press; 1991; 1113 p.
- Huang B-W, Kung H-K, Chang K-Y, Hsu P-K, Tseng J-G. Human cranium dynamic analysis. Life Sci J 2009; 6(4):15-22.