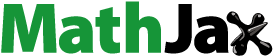
ABSTRACT
Prediction of tissue damage under thermal loads plays important role for thermal ablation planning. A new methodology is presented in this paper by combing non-Fourier bio-heat transfer, constitutive elastic mechanics as well as non-rigid motion of dynamics to predict and analyze thermal distribution, thermal-induced mechanical deformation and thermal-mechanical damage of soft tissues under thermal loads. Simulations and comparison analysis demonstrate that the proposed methodology based on the non-Fourier bio-heat transfer can account for the thermal-induced mechanical behaviors of soft tissues and predict tissue thermal damage more accurately than classical Fourier bio-heat transfer based model.
Introduction
Thermal ablation is a minimally invasive thermal treatment for curing unrespectable tumors located inside human organs such as the liver and stomach. Currently, the thermal ablation process is controlled using temperature elevation to form tissue coagulation zones.Citation1 This method is inaccurate, as an optimized temperature field does not necessarily imply optimized tissue damage. It also ignores the effect of thermal-mechanical deformation during the thermal therapy. In fact, even a small variation in thermal-induced mechanical deformation can lead to various effects such as protein denaturation, altered production of hormones, and suppressed immune response.Citation2 Therefore, it is an absolute necessity to study tissue response under thermal and mechanical loads to provide an effective guidance for precise control of thermal ablation tasks.
Several authors have studied thermal-mechanical behaviors of soft tissues under thermal therapy. Xu et al.Citation3 treated human skin as a kind of composite material, laminate, where heat-induced mechanical response (thermal stress) in different layers of skin was calculated using traditional composite material mechanics. However, this method is only limited to layered soft tissues and how thermal-mechanical response is incorporated into existed damage model to qualify thermal-mechanical damage was not discussed. In order to identify the influence of thermally induced mechanical deformation on thermal damage prediction, Shen et al.Citation4,5 developed a tissue damage model using Fourier bio-heat transfer equation. It shows that thermally induced mechanical deformation decreases the activation energy for protein denaturation, making soft tissues more easily to be damaged. Recently, the author's group developed a model to predict thermally induced mechanical deformation and thermal damage of soft tissues by combining the Fourier bio-heat transfer equation with the theory of linear thermo-elasticity.Citation6,7 In general, most of the existing methods are mainly dominated by the classical Fourier bio-heat transfer equation,Citation8 which assumes infinite speed of heat propagation in soft tissues. In fact, a change temperature in a local area does not instantaneously cause a perturbation on the temperature distribution in the rest area of the medium. The heat transfer in a tissue like non-homogenous medium needs a characteristic time to propagate to the nearest point. This non-Fourier thermal behavior has been observed in biological tissues via physical experiments.Citation9-11
This paper gives a new methodology for modeling of thermal-mechanical behaviors and associated damage of soft tissues during thermal ablation, where the modeling process combines non-Fourier bio-heat transfer, continuum mechanics, as well as non-rigid motion of dynamics to predict and analyze temperature distribution and thermal-induced mechanical deformations of soft tissues. An improved tissue damage model is developed by accounting for thermal-induced mechanical damage. The finite difference scheme and numerical algorithms are utilized to construct the proposed thermal-mechanical tissue damage model. Simulations and comparison analysis with the classical Fourier bio-heat transfer have been performed to evaluate the performance of the proposed methodology.
Material model and numerical modeling
Non-Fourier bio-heat transfer equation
The classical Fourier based bio-heat transfer equation considering coupled thermo-elastic effect can be described as,Citation12(2)
(2) where ρ is the density soft tissues, C the specific heat, β the coefficient of thermal expansion, u the displacement vector, k the thermal conductivity, T the temperature at time t,
the blood perfusion,
the density of blood, Cb the specific heat of blood, Tb the temperature of blood vessel, T0 the initial temperature,
the external heat source, and Qm the generated metabolic heat.
Applying the concept of finite heat propagation velocity,Citation3 a modified unsteady heat transfer equation can be obtained(2)
(2) where
is the characteristic time of biological soft tissues.
Using first-order Taylor expansion, the above equation becomes(3)
(3)
Substituting (Equation3(3)
(3) ) into (Equation1
(2)
(2) ), the non-Fourier bio-heat transfer equation can be obtained,
(4)
(4)
Linear elastodynamics
Biologically, soft tissues are complex in terms of material compositions and structural formations. While soft tissue structure shows time-dependent, nonlinear and anisotropic behaviors, in terms of small deformation caused by thermal load, soft tissues can be investigated by linear thermo-elastic models to a high precision.
From the constitutive elastic material law under thermal loads, the stress tensor is related to the strain tensor and temperature change θ, which equals (5)
(5) where
is the displacement components, and
is the Kronecker's symbol defined as
Rewriting the governing equation of non-rigid mechanics of motion as(6)
(6)
Rearranging the motion equation as,(7)
(7) where λ and μ are the Lame constants, F is the exerted external force and,
Constructing the proposed model on a regular cubic mesh straightforwardly using a finite difference scheme, gives the following global characteristic equation for all mesh points:(8)
(8)
To solve Eq. (Equation8(8)
(8) ), it is necessary to specify the boundary conditions under concerning. Here, the boundary condition for solving Eq. (Equation8
(8)
(8) ) is realized by Dirichlet boundary condition, in which specified temperature and displacement are enforced to the related points on the solution domain boundaries at all times.
Thermal-mechanical damage prediction model
To predict thermal damage with consideration of thermal induced mechanical deformation effect, the relevant governing equation is expressed in Eq. (Equation9(9)
(9) ). Basically, it assumes that soft tissues subject to thermal stress cause tissue deformation. Thus, tissues are more easily being damaged under such a condition, because tissue protein denaturation may occur at relatively small activation energy.
(9)
(9) where
is the strain energy per mole, which is represented by Eq. (10).
stands for molar volume of target tissue.
(10)
(10)
Results and discussion
Several simulations were conducted to test the performance of proposed thermal-mechanical model based on non-Fourier bio-heat transfer. Consider a cubic volumetric tissue model, which contains 1000 elements with 1331 nodes, with a needle inserted inside (see ). The needle has 5 evenly spaced point sources at the front to generate heat energy and they are positioned in the Y direction at the center of the middle horizontal plane inside the cubic tissue. The needle generates thermal energy at the 5 points at to heat up the tissue model. shows the thermal and mechanical parameters used for the cubic tissue under thermal loads.Citation13-18 The boundary of the cubic model is fixed and its temperature is set to 310 K. The cubic model has the initial temperature of 310 K at the rest state.
Table 1. Tissue parameters and constants.
Temperature distributions
To study the thermal behavior of the cubic tissue, 3 observation points P1 (0.005, 0.005, 0.005), P2 (0.005, 0.005, 0.006) and P3 (0.005, 0.005, 0.007) (blue points in ), were chosen to observe the temperature variation inside the cubic tumor during the heating process. illustrates the temperature distributions at these 3 points within a heating period of 120 s for both models. As we can see, at the initial stage of heating (within about 40 s), the non-Fourier model involves oscillations in the temperature rise. After the initial stage of heating, with the increase of the heating time, the temperature rise for the non-Fourier model is larger than Fourier model, but the difference between both models gradually becomes smaller and finally the temperature curves of both models are converged to each other. This demonstrates that although the Fourier and non-Fourier models have similar accuracy for temperature prediction after a sufficient time of heating, the non-Fourier bio-heat model accounts for the transition state of bio-heat transfer, thus more suitable for the prediction of tissue's instantaneous thermal-mechanical behavior.
To further study the thermal behavior of the cubic tumor, the plane at Y = 0.005 m, which is perpendicular to the heating line, was selected to observe the temperature variation during the heating process. shows the temperature distributions on this plane at the initial time of 10 s for both Fourier and non-Fourier models. It can be seen that the temperature distributions of both models have a similar behavior with the highest temperature at the center of the selected plane, which is also the center of the heating sources. However, the non-Fourier model has smaller temperature rise than the Fourier model. This is because the non-Fourier model involves a process of time relaxation, leading to the smaller temperature rise at the initial heating stage.
Thermal-induced mechanical deformations
Trials were also conducted to study the thermal-induced deformation behaviors of the cubic tumor. shows the strain distributions at the above plane (Y = 0.005 m) at the heating time of 10 s for both Fourier and non-Fourier based methods. It can be seen that following the temperature distributions shown in , the strain in the Y direction of the non-Fourier based method is also smaller than that of the Fourier based method. It should be noted that as the selected observation plane is perpendicular to the Y axis, the strains in the X and Z directions are of a similar behavior, but are different from that of the strain in the Y direction. The above similar phenomenon can also be observed for the stresses in the 3 axis directions shown in . shows the strain energy distributions for both models. It can be seen that the non-Fourier model has smaller strain energy than the Fourier model at the initial heating time of 10 s. This proves that the strain energy distribution also follows the temperature distributions shown in . From the above, it is evident that the temperature distribution has a significant effect on thermal-induced strain and stress as well as strain energy.
Figure 4. Comparison of strain distributions between the Fourier (top) and non-Fourier (bottom) based methods.
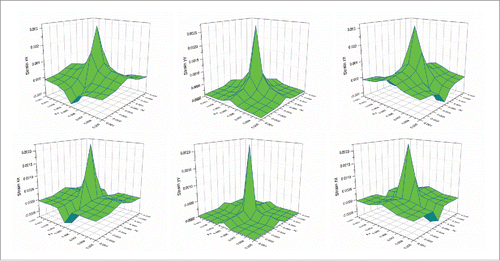
Damage distribution
Simulations were also conducted to test the performance of the proposed thermal-mechanical tissue damage model. shows the damage comparison between the Fourier and non-Fourier models at the 3 selected points P1-P3. As the non-Fourier based method involves the transition state at the initial heating stage, at the points (e.g. P1 and P2) nearer to the center of the heat sources (i.e. the center of the selected observation plane), it needs more time to reach the threshold of irreversible tissue thermal damage (Ω = 1)Citation3 than the Fourier based method. This phenomenon basically follows the above analysis at initial heating time of 10 s for points P1 and P2, where both temperature and strain energy prediction values were larger for the Fourier model. However, at the points (e.g., P3) further away from the center of the heat sources, it needs less time to reach the tissue damage threshold than the Fourier based method. This can be attributed to larger temperature prediction values toward the time point where the damage threshold was reached (around 60 s) for the non-Fourier model at point P3, as shown in . Moreover, shows the damage distributions at the above plane (Y = 0.005 m) at the end heating time of 120 s. It can be seen that the final tissue damage zone for the non-Fourier based method (in green) is clearly larger for that of the Fourier based method (in Bangladesh green).
Conclusion
This paper presents a new methodology to predict thermal-induced mechanical deformation and associated tissue damage of soft tissues under thermal load. This methodology establishes a thermal-mechanical model and tissue damage model based on non-Fourier bio-heat transfer and continuum mechanics. Simulations and comparison analysis demonstrate that the proposed methodology based on non-Fourier bio-heat transfer accounts for thermal-induced mechanical deformation of soft tissues more accurately, leading to more accurate prediction of tissue thermal damage. Future work will focus on the construction of the proposed methodology on irregular human organ models using finite element method.
Disclosure of potential conflicts of interest
No potential conflicts of interest were disclosed.
References
- Zhang B, Moser M, Zhang E, Zhang W. Radiofrequency ablation technique in the treatment of liver tumours: review and future issues. J Med Eng Technol 2013;37:150-9; PMID:23360198; http://dx.doi.org/10.3109/03091902.2012.754510
- Dai W. Skin Burn Injury Prediction. Encyclopedia of Thermal Stresses, R. B. Hetnarski (Ed.), Dordrecht (Netherlands): Springer, 2014:4421-7; http://dx.doi.org/10.1007/978-94-007-2739-7, ISBN:978-94-007-2738-0.
- Xu F, Lu T. Introduction to skin biothermomechanics and thermal pain. Berlin (Heidelberg):Springer, 2011; http://10.1007/978-3-642-13202-5; ISBN:978-3-642-13202-5.
- Shen WS, Zhang J. Modeling and numerical simulation of bioheat transfer and biomechanics in soft tissue. Math Comp Model 2005;41:1251-65; http://dx.doi.org/10.1016/j.mcm.2004.09.006
- Shen WS, Zhang J, Yang FQ. Skin thermal injury prediction with strain energy. Int J Nonlinear Sci Numer Sim 2005;6:317-28; http://dx.doi.org/10.1515/IJNSNS.2005.6.3.317
- Li X, Zhong Y, Jazar R, Subic A. Thermal-mechanical deformation modelling of soft tissues for thermal ablation. Bio-med Mater Eng 2014;24:2299-310; PMID:25226930
- Li X, Zhong Y, Subic A, Jazar R, Smith J, Gu C. Prediction of tissue thermal damage. Technol Health Care 2016;s2:5625-5629; PMID:27163325; http://dx.doi.org/10.3233/THC-161189.
- Pennes HH. Analysis of tissue and arterial blood temperatures in the resting human forearm (Reprinted from Journal of Applied Physiology, vol 1, pg 93-122, 1948). J Appl Physiol 1998;85:5-34; PMID:9714612
- Mitra K, Kumar S, Vedevarz A, Moallemi M. Experimental evidence of hyperbolic heat conduction in processed meat. J Heat Transfer 1995;117:568-73; http://dx.doi.org/10.1115/1.2822615
- Xu F, Seffen K, Lu T. Non-Fourier analysis of skin biothermomechanics. Int J Heat Mass Transfer 2008;51:2237-59; http://dx.doi.org/10.1016/j.ijheatmasstransfer.2007.10.024
- Banerjee A, Ogale AA, Das C, Mitra K, Subramanian C. Temperature distribution in different materials due to short pulse laser irradiation. Heat Transfer Eng 2005;26:41-9; http://dx.doi.org/10.1080/01457630591003754
- Jimenez-Lozano J, Vacas-Jacques P, Franco W. Thermo-elastic response of cutaneous and subcutaneous tissues to noninvasive radiofrequency heating. Proceedings of the 2012 COMSOL Conference in Boston, 2012 October 3-5; Boston, Massachusetts; ISBN:978-0-9839688-9-4.
- Evans DW, Moran EC, Baptista PM, Soker S, Sparks JL. Scale-dependent mechanical properties of native and decellularized liver tissue. Biomech Model Mechanobiol 2013;12:569-80; PMID:22890366; http://dx.doi.org/10.1007/s10237-012-0426-3
- Davalos RV, Mir L, Rubinsky B. Tissue ablation with irreversible electroporation. Ann Biomed Eng 2005;33:223-31; PMID:15771276; http://dx.doi.org/10.1007/s10439-005-8981-8
- Keangin P, Wessapan T, Rattanadecho P. Analysis of heat transfer in deformed liver cancer modeling treated using a microwave coaxial antenna. Appl Thermal Eng 2011;31:3243-54; http://dx.doi.org/10.1016/j.applthermaleng.2011.06.005
- López-Molina JA, Rivera MJ, Trujillo M, Burdío F, Lequerica JL, Hornero F, Berjano EJ. Assessment of hyperbolic heat transfer equation in theoretical modeling for radiofrequency heating techniques. Open Biomed Eng J 2008;2:22; http://dx.doi.org/10.2174/1874120700802010022
- Schutt DJ, Haemmerich D. Effects of variation in perfusion rates and of perfusion models in computational models of radio frequency tumor ablation. Med Phys 2008;35:3462-70; PMID:18777906; http://dx.doi.org/10.1118/1.2948388
- Roan E. The effect of Glisson's capsule on the superficial elasticity measurements of the liver. J Biomech Eng 2010;132:104504; PMID:20887022; http://dx.doi.org/10.1115/1.4002369