Abstract
Flexible energy storage systems have substantial inherent advantages in comparison with many currently employed systems due to improved versatility, performance and potentially lower cost. The research within this field is currently undergoing tremendous developments as new materials, composites and large-scale assembly strategies are being developed. In this review, we summarize recent progresses toward the development of flexible electrodes based on carbonaceous nanomaterials with particular emphasis on rational electrode design. Strategies to assemble flexible electrodes, both with and without inert mechanical supports, are reviewed and compared. Depending on their composition, the flexible electrodes can be used in important energy storage systems including supercapacitors and lithium ion batteries. The trend on future developments is also analyzed.
1. Introduction
The continued growth of global population and economy has placed increasing demand for energy and the worldwide energy consumption is predicted to double by the middle and triple by the end of the century.Citation1 Currently, the majority of energy consumed is derived from fossil fuels (∼70%).Citation2Citation3 However, the worldwide fossil fuel reserves are limited and their consumptions result in production of greenhouse gases that are usually considered as the primary source for global warming. Hence, there are strong interests to develop efficient, clean and renewable energies (such as wind and solar energy).Citation4 However, the electricity generated from these sources is generally intermittent and geographically limited and therefore they often require efficient electrical energy storage (EES) systems.Citation5 Additionally, efficient EES systems are also required to progress from today's hybrid vehicles to plug-in hybrids or even all-electric vehicles and for consumer electronics.Citation6 Electrochemical energy storage systems, such as batteries and supercapacitor, are among the leading EES technologies nowadays.Citation7 Their capabilities to store and release energy are usually compared on the basis of energy and power densities. Energy density is the amount of energy stored per unit volume or mass, whereas power density is the amount of power (time rate of energy transfer) that can be released per unit volume or mass. Due to different operation mechanisms, batteries have high energy densities but with low power densities, whereas supercapacitors have high power densities with low energy densities.Citation8 With the existing technologies, however, neither of them could fully meet the requirements for providing electricity efficiently for the various applications described above. Therefore, the performance of batteries and supercapacitors need to be substantially improved in order to enable the required properties of high specific power and energy densities, long cycle life, low cost and improved safety.Citation9Citation10
Conventionally, electrodes for batteries and supercapacitors are fabricated by casting a mixture of active materials, conducting additive and binder on a piece of metallic current collector.Citation11Citation12 Using this approach, the active materials only represent a small fraction (∼ 30 wt%) when considering the total weight of electrolyte, separator and other functional components.Citation13 As a consequence, the device-level performance metrics are substantially reduced since only the active materials can contribute to energy storage. Hence, in addition to improving the activity and efficiency of the active materials, another type of promising approaches for high-performance energy storage is to reduce the total weight of functional components. Among these approaches, the fabrication of flexible electrodes received particular interests. One reason is that heavy metallic current collectors, conducting additive and binder are usually not necessary for flexible electrodes, thus could reduce the total weight of the device.Citation14Citation15 The removal of metallic current collectors also has benefits including improved cycling stability and corrosion resistivity. Additionally, flexible electrodes offer better manufacturability since they can be folded, twisted or cut to fit any desired spaces. The continued developments in consumer electronics, including flexible displays, wearable electronics and portable devices, also desire advanced flexible energy storage systems.Citation16
Recent developments in carbon nanotubes (CNTs) and graphene bring substantial opportunities for developing advanced flexible electrodes given their strong mechanical strength, superior electrical conductivity, lightweight and compatibility with a variety of redox-active materials.Citation4Citation17 Over the past decades, many approaches have been developed to fabricate flexible electrodes in which the electrode materials have been either pure carbon Citation18–Citation20 or a combination of carbon and redox-active materials, such as TiO2, MnO2 and activated carbon (AC).Citation14Citation21 Depending on their composition, such electrodes can be used for applications including batteries and supercapacitors. In the subsequent sections, we review recent progresses toward the fabrication of flexible electrodes for energy storage. Strategies to assemble electrodes, both with and without inert mechanical supports, are reviewed and compared. Since device data reported in the literature have been acquired using different types of configurations (two or three electrodes) and calculation of specific capacitances are often based on the weight of electroactive material only, it is generally difficult to obtain direct and clear comparisons of the flexible electrodes fabricated in different studies. Additionally, different electrode parameters (size, thickness, active material loading, etc.) often lead to very different results even using the same electrode testing system. In the present review, we therefore particularly focus on the rational design of flexible architectures and, when possible, highlight the method of measurements and calculations used to obtain these results.
2. Flexible Electrodes Based on Pure Carbon Materials
This section summarizes recent developments on the fabrication of flexible electrodes using pure carbon nanomaterials, where CNTs and graphene are mostly applied due to their remarkable properties including high mechanical strength, high surface area and superior electrical conductivity that are required for energy storage.Citation22Citation23 Such electrodes are mainly used in supercapacitors owing to the physiochemical properties of carbon and store electricity through the electrical double-layer capacitance mechanism in either aqueous or nonaqueous electrolytes. There are also some studies on applying these electrodes as anodes for lithium ion batteries as discussed in the following sections.
2.1. Flexible Electrodes Without Inert Mechanical Support
2.1.1. CNT Buckypaper
When assembled, the strong interactions between interconnected nanocarbon pieces enable the formation of freestanding films with varied degrees of mechanical strength depending on the structure of carbon materials and their engineering process.Citation24 Several early studies have demonstrated that upon simple vacuum filtration, CNTs readily form freestanding films that are known as CNT mat or buckypaper ().Citation27–Citation29 Buckypapers have remarkable mechanical strength and could withstand large forces before plastic deformation. For example, single-walled carbon nanotube (SWNT) buckypapers typically have tensile strength of 80–100 MPa and Young's modulus of 5–10 GPa. Buckypapers can be directly used as electrodes for storing electricity without any current collectors or binders Citation30 owing to their remarkable mechanical strength, inherent high surface area (up to ∼ 1,000 m2/g) and excellent electrical conductivity (∼ 5×105 S/m) of CNTs.Citation31 Depending on the type of nanotubes, buckypapers exhibit specific capacitance as high as ∼100 F/g in aqueous electrolyte and ∼80 F/g in nonaqueous electrolyte ().Citation32Citation33Citation40 The primary difference between aqueous and nonaqueous electrolytes is different operation voltages. Supercapacitors have higher operation voltages with nonaqueous electrolyte (e.g. ∼2.7 V with acetonitrile-based electrolyte) and thus higher energy densities (E=CV2/2) as opposed to aqueous-based electrolytes that exhibited more limited potential windows (∼1 V).Citation41 However, the use of aqueous electrolytes could support high-power operations because of their high ionic conductivity and high concentration of ions, as well as cost and safety advantages. Additionally, with aqueous electrolyte the need for extensive electrolyte purification and handling under a controlled atmosphere is minimized and the fabrication and packaging process are greatly simplified.Citation42
Figure 1. (a) Photograph of a freestanding SWNT buckypaper prepared using the vacuum filtration method. (b) scanning electron microscopy (SEM) image of the buckypaper. (c) Image of SWNT paper strips that are bent around a curved surface. Adapted from Landi et al.Citation25 (d) Cyclic voltammogram of buckypaper measured at 50 mV/s. (e) Galvanostatic charge–discharge curves measured at 5 mA. Adapted from Ci et al.Citation26
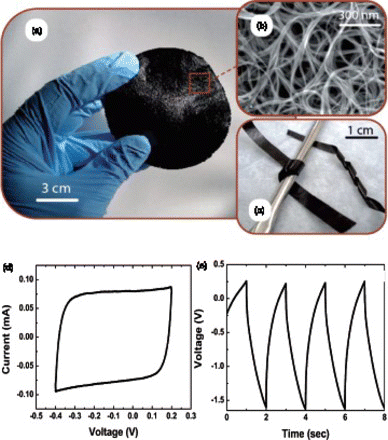
Table 1 Typical results obtained from flexible electrodes assembled using pure carbon nanomaterial for supercapacitors or lithium ion batteries..
In addition to the most widely used vacuum filtration approach, freestanding CNT films can also be obtained by directly peeling off the vertically aligned CNT forests that were pre-synthesized using the chemical vapor deposition (CVD) method.Citation30Citation43Citation44 The flexible electrodes fabricated using this method usually have similar specific capacitance as the buckypaper (), but they have the additional advantage of controlled alignment.Citation26Citation30 Freestanding electrodes could be readily obtained with the methods described above. However, the fabrication of flexible electrodes with arbitrary larger sizes is challenging because the sizes of filtration membrane and CVD systems are both limited. Recently, Xie and co-workers.Citation45 developed a scalable process to end-by-end assemble individual buckypapers with a tensile strength of 250 MPa (). Supercapacitor electrodes assembled using this method showed a specific capacitance of 35 F/g, high energy and power densities (43.7 Wh/kg, 197.3 kW/kg) in an organic electrolyte using the two-electrode testing system.Citation33 This approach is also potentially applicable to assemble large electrodes for other flexible systems. The inherent advantage of buckypaper-based electrodes for supercapacitors is ultrahigh power densities (>100 kW/kg) compared with other materials such as graphene and AC because of their highly conductive and porous characteristics.Citation46 However, their energy densities are relatively low because of limited surface area. Various strategies have been developed to improve their energy densities.Citation46–Citation48 For example, the specific capacitance of buckypapers was increased from 75 to 290 F/g after plasma treatment.Citation49
Figure 2. Schematic diagram of assembling compact-designed supercapacitor using freestanding flexible SWNT films. Reproduced with permission from Niu et al.Citation33
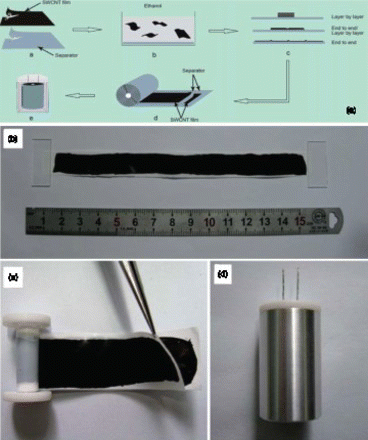
Besides working as supercapacitor electrodes, CNT papers were also studied as flexible anodes for lithium ion batteries. They can deliver reversible capacities of 250–500 mAh/g depending on the type of nanotubes and testing conditions with substantially improved rate capabilities compared with traditional graphite-based electrodes.Citation25,Citation50Citation51Citation52
2.1.2. Flexible Graphene Paper
Graphene is under intense research in recent years because of their unique properties including much higher surface area than nanotubes and outstanding electric conductivity.Citation53–Citation55 Similar to buckypapers, freestanding graphene papers can also be prepared through either vacuum or pressure filtration where stable dispersions are filtered through a porous inert membrane.Citation56 Graphene papers usually have remarkable strength due to strong inter-sheet van der Waals attractions between adjacent graphene sheets.Citation17Citation57 However, they cannot be directly used as electrodes for double-layer capacitors because of their low surface area caused by restacking of graphene sheets.Citation58 A possible approach to minimize restacking is to use a ‘spacer’ to separate adjacent graphene sheets during the preparation of graphene papers.Citation59 The spacers can be dissolved afterwards to obtain highly porous graphene paper. For example, porous graphene papers with specific capacitance up to 202 F/g was obtained at 1 A/g with polystyrene particles as the spacer, whereas pristine graphene papers only have 97 F/g.Citation36 Noticeably, some spacers could also contribute to energy storage and work together with graphene to enable improved performance toward storing electricity (). For example, flexible electrodes made with graphene and carbon black nanoparticles were able to deliver 138 and 83 F/g in aqueous and nonaqueous electrolytes, respectively, which are both higher than pure graphene papers without carbon black.Citation60
Alternatively, restacking of graphene can also be effectively minimized by controlling the preparation process of graphene papers.Citation20Citation34Citation35 In one study, wet graphene papers that were prepared just after vacuum filtration without drying were directly used as supercapacitor electrodes and showed better performance than dried papers. The ‘wet’ graphene papers, with water serving as the spacer, were able to deliver specific capacitance as high as 215 F/g, which is much higher than dried graphene films ().Citation20 In another study, porous graphene papers were prepared from dried graphene oxide papers using an autoclaved leavening and steaming strategy. In this case, the controlled release of gases leads to the formation of highly porous graphene papers. Flexible electrodes prepared using this method were able to deliver ∼110 F/g using the two electrode measurement method.Citation35 Recently, Xu et al. fabricated flexible supercapacitors using 185 μm graphene hydrogel thin films and demonstrated remarkable areal specific capacitance of up to 402 mF/cm2 as well as excellent cycling stability and mechanical flexibility. These results show that graphene hydrogels can also be used for high performance flexible energy storage devices.Citation61
Figure 3. Characterization of self-stacked, solvated graphene (SSG) film. (a,b) photographs of the as-formed flexible SSG film, (c) schematic of the cross-section of the SSG film, (d) CV curves obtained at 10 mV/s and (e) gravimetric capacitances measured at various charge–discharge currents. Reproduced from Yang et al.Citation20
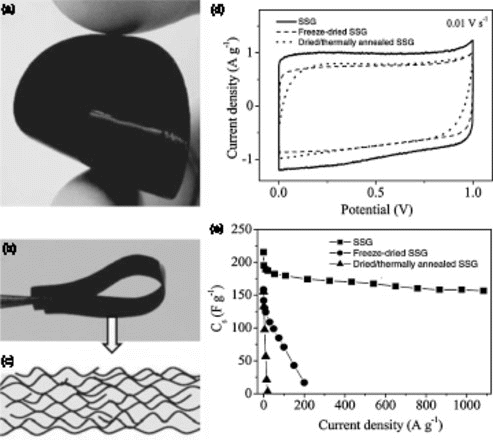
2.1.3. Flexible Electrodes Based on Composite Carbon Nanomaterials
Composite flexible electrodes consisting of more than one type of carbon nanomaterials were actively pursued to take the advantages of different materials.Citation62 CNTs and graphene composites, in particular, have high surface area and porous structure that are both beneficial for electronic and ionic transport. Furthermore, previous studies have established that graphene oxide could work as an efficient surfactant to disperse CNTs and therefore uniform mixture of CNTs and graphene could be prepared.Citation63 When prepared as flexible electrodes, such composites generally have better performance than either of CNTs or graphene alone, and a remarkable specific capacitance of 265 F/g was achieved for electrodes with 16 wt% of multiwalled carbon nanotubes ().Citation37 In another similar study, 326 F/g was obtained for composite electrodes with 10% CNTs, which is also substantially higher than pure CNTs and graphene electrodes.Citation64
Figure 4. (a) digital and (b) SEM image of CNT and graphene composite electrode (16% CNTs), (c) specific capacitances of nanotube/graphene composites with different nanotube percentages measured within−1.0 to 0 V vs. saturated calomel electrode at 0.1 A/g in 6 M KOH. Adapted from Lu et al.Citation37
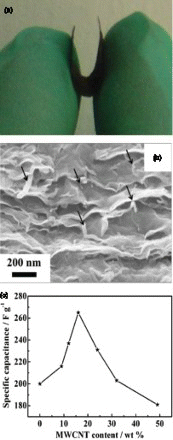
Composite flexible electrodes other than graphene/ CNTs were also actively studied. In a recent study, flexible electrodes were fabricated using SWNTs and nanohorns. The combined contributions from nanotubes and nanohorns enable flexible electrodes with high meso–macro pore volume (2.6 ml/g) and facilitated ion transport. High power density of 990 kW/kg in 1 M Et4NBF4/propylene carbonate electrolyte with outstanding stability (6.5% decline in 100,000 cycles) was successfully demonstrated.Citation18 AC is currently widely used in the production of supercapacitors because of their high specific surface area and high specific capacitance in both aqueous and nonaqueous electrolytes.Citation65 The fabrication of flexible electrodes based on AC is particularly attractive due to their existing wide applications. This can be achieved by vacuum filtration of the stable suspension containing both CNTs and AC particles.Citation15Citation38Citation66 Experimental results also demonstrated that the flexible composite electrodes have much better performance compared with other electrodes made with traditional approaches. Flexible and binder-free CNTs/AC electrodes containing 95 wt% of AC were able to reach specific capacitance of 268 F/g at 200 mV/s in 6 M KOH. The rate performance was also improved and the capacitance retention ratio was increased from 21.8% for the AC/carbon black electrode (traditional approach) to 59.3% for the AC/CNT flexible electrodes when the scan rates were increased from 10 to 200 mV/s. Energy density of 22.5 Wh/kg and power density of 7.3 kW/kg, along with remarkable stability of 97.5% retention for 5,000 cycles were also demonstrated.Citation38
2.2. Flexible Electrodes with Electrochemically Inert Mechanical Supports
Another type of attractive approach for fabricating flexible electrodes is using flexible and porous substrates as mechanical supports. The introduction of mechanical supports brings improved stability and integrity to electrodes during operations. The most widely applied supports are based on flexible, porous and lightweight materials. An excellent example of such supports is paper (printer paper or newspaper), which was developed by Cui et al.Citation39,Citation67–Citation69Citation70 In this case, a piece of paper conformably coated with conductive CNT ink or graphene ink was studied as the flexible electrodes for supercapacitors (). A high specific capacitance of 200 F/g was obtained in aqueous electrolyte. Specific energy of 30–47 Wh/kg and specific power of up to 200 kW/kg on the basis of active nanotubes were obtained when operated at 3 V in an organic electrolyte (). When using the total weight of the complete device, a specific energy of 7.5 Wh/kg was determined. The device exhibited good cycling stability, with ∼ 3% capacitance loss in aqueous electrolyte and ∼ 0.6% in organic electrolyte after 40,000 charge–discharge cycles.Citation39 Furthermore, they can also be used as flexible current collectors as discussed in detail in the following sections.
Figure 5. (a) schematic illustration of a paper-based CNTs supercapacitor device, (b) charge–discharge curves in aqueous (lower curve) and nonaqueous (upper curve) electrolytes, (c) CNT weight normalized capacitance as a function of the charge–discharge current, (d) Ragone plot based on active mass, (e) device specific Ragone plot and (f) cycle life of the supercapacitor device. Adapted from Hu and Cui.Citation69
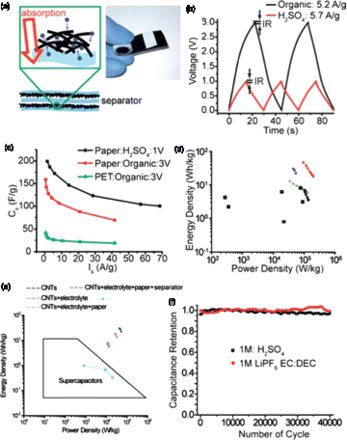
Besides paper, stretchable and porous cotton textiles and sponge were also been studied as substrates for flexible energy storage.Citation71Citation72 Active materials including nanotubes and graphene can be coated with their inks using a ‘dipping and drying’ process, resulting in highly conductive textiles (up to 125 S/cm) which could be utilized directly as flexible electrodes for supercapacitors or as current collectors (). Compared with paper, CNT-coated cotton textiles (fibers) have outstanding elasticity and full-textile supercapacitors are highly stretchable, showing excellent capacitance retention after being stretched to 120% strain for 100 cycles.Citation72 Specific capacitance of up to 140 F/g with 3 V voltage was achieved in 1 M LiPF6 electrolyte. Compared with papers, porous and highly absorbable cotton and sponge could absorb much more CNTs or graphene and reach about ∼ 30 wt% of the electrode total weight. This means that they have significant improvements on the device-level performances since more active material can be loaded. Similarl to cotton textile, cellulose fiber-based paper was also been used to fabricate flexible and stretchable supercapacitors using a vacuum filtration method with graphene as the active material.Citation74 Specific capacitances of 120 F/g and 81 mF/cm2 were obtained with excellent mechanical integrity (6% loss after 1,000 bending cycles). To further increase the active material coating, a ‘screen printing’ method was applied in another study using AC (YP17) as the active material and woven cotton/ polyester fabrics as the flexible substrate. In that study, active material coatings up to ∼ 4.9 mg/cm2 was achieved. The composite electrodes were able to deliver specific capacitance of 85–95 F/g in aqueous electrolyte with areal specific capacitance of ∼ 0.43 F/cm2 and 92% retention for 10,000 cycles, indicating that such a screen printing method is very attractive for large-scale applications.Citation16
Figure 6. Textile-based electrodes for flexible energy storage: (a) Dipping a piece of textile into aqueous CNT ink. (b) SEM image of CNT-coated textile. (c) SEM image of MnO2–CNT–textile. (d) Specific capacitances at different scan rates for MnO2–CNT–textile electrodes with different areal mass loadings of MnO2. (e) SEM image of graphene-coated textile with uniform MnO2 coating (scale bar 200 μ m). (f) SEM image of a typical microfiber with conformal coating of MnO2 nanostructures (scale bar 5 μ m). Adapted from Yu et al.Citation73
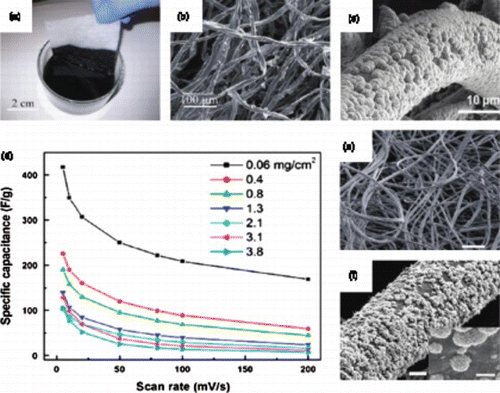
Planar flexible substrates made with plastic also showed substantial promise as supports despite they usually load much less active materials compared with porous paper and textiles. By spraying CNTs onto a polyethylene-therephthalate (PET) substrate, flexible electrodes were successfully made and demonstrated with specific capacitance of 90–120 F/g using solid electrolytes.Citation75 Similarly, functionalized reduced graphene oxide thin films that were prepared by vacuum filtration were combined with Au-coated PET films. Flexible all solid-state supercapacitors fabricated using such electrodes were able to deliver specific capacitance of 118 F/g at 1 A/g and remarkable rate capability (90% retention at 30 A/g).Citation76
3. Flexible Electrodes Based on Composites with Carbon Nanomaterials
The amount of energy (energy density) that can be stored in flexible electrodes consisting only carbon materials (CNTs and/or graphene) is ultimately limited by the specific surface area of active materials.Citation77 To further increase their energy densities, flexible electrodes consisting of both carbon and redox-active materials such as metal oxides and/or conducting polymers have been actively pursed. The introduction of redox-active materials enables electricity to be stored via redox reactions that have a much higher capacity than the double-layer capacitance as described previously.Citation78 In the subsequent sections, we will review the design and fabrication of hybrid flexible electrodes for supercapacitors, with particular emphasis on inorganic electroactive materials. Readers may refer to several previously published reviews for conducting polymer-based flexible electrodes.Citation79Citation80
3.1. Manganese Oxides (MnO2) Based Flexible Supercapacitors
MnO2 have been widely studied for pseudocapacitive supercapacitors during the last decade owing to their attractive features, including natural abundance, environmental benign and high theoretical capacitance (∼ 1,380 F/g).Citation81Citation82 Hence, the fabrication of flexible MnO2-based supercapacitors is particularly attractive. Electrochemical deposition of MnO2 on conductive and flexible substrates is one of the most widely used approaches to fabricate flexible MnO2 electrodes.Citation83 The mass loading densities and structure of deposited MnO2 can be readily controlled by tuning the deposition voltage, current and electrolyte and properties of the flexible substrates have significant influences over their energy storage performance.Citation84Citation85 Kang et al. deposited MnO2 onto CNTs coated printer paper and found that the specific capacitance of such flexible electrode was able to reach 540 F/g at 2 mV/s. However, the active MnO2 loading was only 0.38 mg/cm2 and therefore the electrode-level performance metrics, when considering its total weight, were actually quite poor.Citation86 Therefore, highly conductive and porous substrates are desired for depositing MnO2 with higher mass densities. In this case, cotton textiles and polyester fabrics coated with CNTs or graphene that was discussed in the previous section are excellent choices ().Citation71,Citation87–Citation89 The macroporous nature of these substrates making them capable of loading much more MnO2 and densities up to ∼8 mg/cm2 was successfully demonstrated using CNTs coated textiles.Citation87 Using these substrates, a high capacitance of 410 F/g was obtained when the MnO2 loading was 0.06 mg/cm2. Even though the gravimetric capacitance of MnO2 was decreased along with the increase in MnO2 loading (<100 F/g when MnO2 loadings were >1 mg/cm2), the areal capacitance was increased and reached 2.8 F/cm2 when 8.3 mg/cm2 MnO2 was used.Citation87 Using a similar approach, CNTs coated sponges were examined as the flexible substrate for depositing MnO2. Experimental results demonstrated that the lightweight and highly absorbing sponges were able to load MnO2 as high as 12.8 mg/cm2. High specific capacitances of ∼ 1,000 F/g was obtained when MnO2 loading was less than 0.1 mg/cm2. As the density of MnO2 was increased, its specific capacitance was also decreased to ∼ 200 F/g.Citation71 The MnO2–CNT–sponge supercapacitors show only 4% degradation after 1,000 cycles at charge–discharge specific current of 5 A/g and their specific power and energy are high with values of 64 kW/kg and 31 Wh/kg, respectively. Following these studies, a three-dimensional conductive wrapping strategy using CNTs ink or conducting polymers was developed to further increase the performance of MnO2/graphene/textile electrodes. Specific capacitances of the flexible electrodes were substantially increased by ∼20% and ∼45% after wrapping with CNTs and conducting polymers, respectively, with values as high as ∼ 380 F/g being achieved. Moreover, the wrapped electrodes also exhibited remarkable cyclic stability with >95% retention over 3,000 cycles.Citation70 These results demonstrated that the conductive coating approach is a promising approach for enhancing the device performance of metal oxide-based electrochemical capacitors and can be generalized for designing next-generation high-performance flexible energy storage devices.
Also using the electrodeposition method, many other flexible substrates were also used to fabricate MnO2-based flexible electrodes. Some typical examples are summarized as follows (as well as in ): carbon cloth (MnO2 specific capacitance up to 425 F/g with good stability for over 3,000 cycles under bending test) Citation90; carbon fabric with carbon nanoparticles (MnO2 capacitance up to 800 F/g, areal capacity up to 109 mF/cm2) Citation91; Zn2SnO4-coated carbon microfiber (621.6 F/g at 2 mV/s) Citation92; ZnO-coated carbon cloth (138.7 mF/cm2, 1,260.9 F/g and 87.5% retention after 10,000 cycles) Citation99; CNTs buckypaper (516 F/g at 77 mA/g)Citation94 and graphene 3-D network that was obtained by direct growth using 3-D Ni foam as the sacrificial substrate (465 F/g with 0.1 mg/cm2 of MnO2, 1.42 F/cm2 with 9.8 mg/cm2 MnO2).Citation95
Table 2. Examples of flexible electrodes enabled by carbon nanomaterials for supercapacitors reported in the literature.
Most of the approaches described above for fabricating flexible electrodes involved an ‘electrochemically inert’ component in their electrode structures (such as cotton textile and printer paper). These inert components, however, did not contribute to energy storage and therefore will reduce device-level energy storage metrics. Hence, there are rising interests on the fabrication of flexible electrodes without using inert supports. Li et al. fabricated flexible electrodes using graphene/MnO2 composites simply by vacuum filtration of their aqueous dispersion. Their study indicates that flexible electrodes with 24% of MnO2 were able to deliver 256 F/g at 0.5 A/g but the MnO2 loading is very low (∼0.1 mg).Citation93 Electrodes with low MnO2 loadings generally have high electrochemical activity, but practical applications require high MnO2 loadings.Citation13 In general, however, fabrication of flexible electrodes with both high MnO2 loading and high specific capacitance is very challenging as any increases in MnO2 loading will lead to decreases in performance. In a recent study, Cheng et al. developed a strategy to fabricate flexible electrodes with high specific capacitance at thick MnO2 coatings by enabling the synergistic effects from graphene and CNTs.Citation14Citation15 In that work, functionalized CNTs were used to link the pre-synthesized graphene/MnO2 pieces (). The introduction of highly conductive CNTs brings about remarkable conductivity (∼5Ω/□) and mechanical strength (Young's modulus of 2.3 GPa and tensile strength of 48 MPa) to the freestanding electrodes. Moreover, the energy storage activity was substantially improved with the interconnected CNTs working as the nanoscale current collector. Specific capacitances as high as ∼ 200 F/g at the areal density of 8.8 mg/cm2, together with remarkable rate performance, was achieved. Additionally, such graphene and CNTs enabled flexible electrodes exhibited excellent cycling performance with >95% retention over 1,000 cycles. Furthermore, the significant thickness-dependence activity of MnO2 electrodes was effectively minimized using this architecture. Such rationally designed flexible architecture presents a general direction for fabricating flexible electrodes with improved performances.
Figure 7. (a) Schematic illustration of the structure of the graphene/MnO2/CNT electrode; (b) SEM image and digital image (inserted) of the fabricated flexible electrode; (c) typical stress–strain curve for the flexible electrodes; (d) CV curves for a 30 μ m flexible electrode (2.02 mg/cm2) acquired at different scan rates and (e) comparison of the specific capacitance of the graphene/MnO2/CNTs and the graphene/MnO2 electrodes at different areal densities tested at 50 mV/s. Adapted from Cheng et al.Citation14
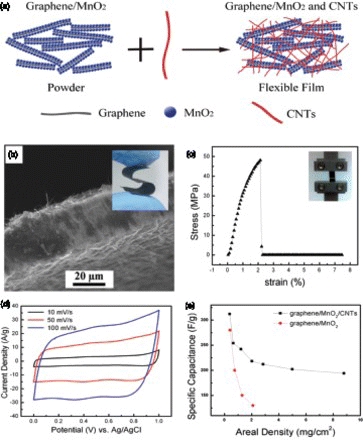
3.2. Flexible Supercapacitors Using Other Metal Oxides/Hydroxides
Several metal oxides/hydroxides, in addition to MnO2 as described in the previous section, also have outstanding pseudocapacitive activities and therefore there are many studies on using these redox-active materials for flexible electrodes. Yuan et al. developed a process to fabricate flexible and porous cobalt hydroxide electrodes using conductive cotton textiles.Citation96 The conductive cotton textile was prepared by uniformly coating CNTs onto textiles also using the dip-coating process. Afterwards the textile was subjected to hydrothermal reactions to coat with the active Co(OH) 2. Electrochemical results show that such composite flexible electrodes were able to deliver high areal specific capacitance of 11.22 F/cm2 at 15 mA/cm2 and even 7.71 F/cm2 at 60 mA/cm2 with good stability of 4% capacity loss after 2,000 cycles at high rates. In another study, flexible electrodes were prepared by electrodeposition of porous Co(OH) 2 nanoflake films on a stainless steel mesh. Such electrodes were able to deliver high specific capacitance of 609.4 F/g, good rate capability (less than 15% as current was increased by 10 times) and remarkable cyclic stability for 3,000 cycles.Citation100
Vanadium oxide is another widely studied material for flexible pseudocapacitors. Boukhalfa et al. used an atomic layer deposition method to deposit ultrathin vanadium oxides on the surface of CNTs that was assembled as buckypaper. Flexible electrodes fabricated using this method were able to deliver remarkable capacitance of up to 1,550 F/g per active mass of the V2O5 and 600 F/g per mass of the composite electrode at 1 A/g in 8 M LiCl.Citation97 In another study, Perera et al. prepared flexible electrodes consisting of V2O5 nanowires and CNTs using the vacuum filtration method. Their study demonstrated that supercapacitors fabricated using such flexible electrodes as the anode and high surface area fiber electrode as the cathode exhibited a power density of 5.26 kW/kg and an energy density of 46.3 Wh/kg in an organic electrolyte (LiTFSI in acetonitrile, 3 V voltage). They further prepared Li+-doped V2O5 nanowires and showed that such nanowire-based flexible electrodes have improved electroactivity, reaching power density of 8.32 kW/kg and energy density of 65.9 Wh/kg. Such electrodes also have remarkable rate performance as they delivered nearly stable capacitance over a wide range of current densities from 0.5 A/g to 10 A/g.Citation101
Several other metal oxides are also being used to fabricate flexible electrodes. Zhang et al. synthesized porous CuO nanobelts with high surface area and small crystal grains and integrated them into flexible electrodes by depositing the mixture of CuO nanobelts and SWNTs as network films onto pure SWNT films without any binders. The flexible electrodes showed specific capacitance of 75.7 F/g when considering the total weight of the electrode in 1 M LiPF6/EC:DEC at 5 A/g with excellent cycling performances.Citation98 Chen et al. prepared flexible In2O3 electrode by filtering its dispersion through a pre-prepared SWNT film. The as-prepared flexible electrodes showed a layered structure and were able to deliver specific capacitance of 201 F/g.Citation102 They further used this electrode to fabricate flexible asymmetric supercapacitors (ASC) as discussed below.
3.3. Flexible ASC
Flexible single electrode can be readily fabricated using the strategies described above. Any working electrochemical energy storage devices, however, require two electrodes with one working as the positive electrode and the other one as the negative electrode. Therefore, the fabrication of supercapacitor devices also requires optimization of the positive and negative electrodes. ASC that use different active materials for the two electrodes have extended voltage window and hence could have higher specific energy density (E ∝ V2) compared with symmetric supercapacitors (identical material for the two electrodes) and received intense research focus over the past few years.Citation77Citation103 In this section we will introduce some of the typical results obtained on the fabrication of flexible ASC where in most cases a flexible metal oxide-based electrode is used as the positive electrode and a flexible carbon-based electrode is used as the negative electrode. In principle, any pure carbon electrodes described in Section 2 and any metal-oxide-based electrodes described in Sections 3.1 and 3.2 can be used to fabricate flexible ASC as long as they can operate in the same electrolyte.
The combination of positive electrodes based on MnO2 and negative electrodes based on carbon is probably the most widely studied system for ASC.Citation77 In principle, the combination of MnO2/carbon should be able to afford ASC with voltages of ∼ 2.0 V in aqueous electrolytes. Yu et al. assembled ASC using graphene/MnO2-textile as the positive electrode and CNT-textile as the negative electrode. The fabrication of such textile electrodes is identical as discussed in the previous sections. The devices can be operated with 1.5 V and were able to deliver energy density of 12.5 Wh/kg and power density of 110 kW/kg that are both higher then symmetric supercapacitors. The devices also have excellent cyclic performance, with ∼ 95% capacitance retention over 5,000 cycles at 2.2 A/g.Citation88 In a recent study, Cheng et al. fabricated flexible ASC that have even a higher voltage of 2 V in an aqueous electrolyte.Citation15 These devices were fabricated using a flexible graphene/MnO2/CNTs film as the positive electrode and AC/CNTs film as the negative electrode without using any current collectors through the roll-up approach (). As has been discussed above, these electrodes have superior flexibility and outstanding mechanical strength owing to the interconnected CNTs networks. The roll-up approach, compared with the stack-up approach as being widely used in conventional devices, has advantages of using large-area electrodes and achieving large capacitance without making the devices too heavy or bulky. Therefore, the roll-up design could have substantial promises in the fabrication of compact devices and being able to be used for this design is another unique advantage inherent to flexible electrodes. Electrochemical testing revealed that the rolled-up ASC devices have superior high rate performance owing to the interconnected and highly conductive nanotubes, with 78% of the original capacitance (46 F/g) retained when the scan rate was increased from 2 to 500 mV/s. Therefore, these devices were able to deliver much higher energy density under high-power conditions compared with other systems, reaching 24 Wh/kg at 7.8 kW/kg.Citation15 In a similar study, Shao et al. used porous graphene/MnO2 nanorod and graphene/Ag thin films that were both prepared using the vacuum filtration method as positive and negative electrode, respectively, in their fabrication of flexible ASC. This study demonstrated that Ag nanoparticles is very important in improving the performance of the graphene negative electrode because they can repair defects in the graphene surface and enhance their electronic conductivities. Additionally, they can also work as a spacer to eliminate the restacking between adjacent graphene sheets. These devices exhibited a maximum energy density of 50.8 Wh/kg and still retain 7.53 Wh/kg at a high power density of 90.3 kW/kg. Their bending tests revealed that such devices are very promising as flexible ASC, with only 2.8% decrease in specific capacitance under bending conditions.Citation104
Figure 8. (a) Schematic illustration of the assembly of a flexible asymmetric supercapacitor using the roll-up approach. The positive electrode is a flexible graphene/MnO2/CNTs film and the negative electrode is AC/CNTs flexible film. (b) CV curves of a asymmetric supercapacitor acquired at increasing scan rates from 2 to 500 mV/s in 1 M Na2SO4, (c) galvanostatic charge–discharge curves obtained under different current densities and (d) comparison of the Ragone plots for supercapacitor devices assembled using different materials and/or different electrode structures. Adapted from Cheng et al.Citation15
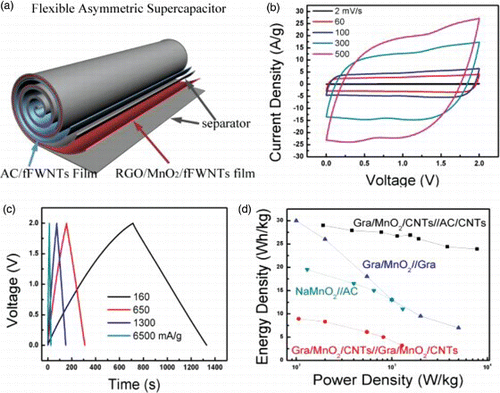
Flexible hybrid electrodes that could effectively utilize the full potential of all the desired functions of each component are particularly promising for developing high-performance electrodes. Lu et al. developed a procedure to fabricate flexible solid-state ASC based on one-dimensional core-shell nanowire hybrids. In their approach, electroactive MnO2 (positive electrode) and carbon shell (negative electrode) were uniformly coated onto hydrogen-treated TiO2 that was pre-synthesized on carbon cloths. Devices fabricated using these electrodes were able to operate in 1.8 V and deliver a high specific capacitance of 139.6 F/g with maximum volumetric energy density of 0.3 mWh/cm3 (59 Wh/kg) and volumetric power density of 0.23 W/cm3 (45 kW/kg). Moreover, the device has excellent cycling performance (8.8% capacitance loss for 5,000 cycles) and good flexibility.Citation105
Other combinations of materials are also being studied besides the MnO2//carbon system as discussed above to prepare flexible ASC with high power and energy densities. A remarkable example is the flexible ASC based on MnO2 and In2O3 and CNT films. The optimized devices were able to operate in 2 V window and deliver specific capacitance of 184 F/g, energy density of 25.5 Wh/kg and power density of 50.3 kW/kg.Citation102 RuO2 is one of the most widely studied materials for supercapacitors due to its remarkable performances. In a recent study, Choi et al fabricated flexible ASC using an ionic liquid functionalized-chemically modified graphene (IL-CMG) film as the negative electrode and a RuO2-IL-CMG composite film as the positive electrode. A solid-state electrolyte made with polyvinyl alcohol–H2SO4 was used to fabricate all-solid-state devices. Such ASC with optimized structure were able to operate with cell voltage up to 1.8 V and deliver a high energy density of 19.7 Wh/kg and power density of 6.8 kW/kg. More remarkably, such devices can be operated with high rate of 10 A/g with 79.4% retention of specific capacitance.Citation106
4. Conclusion and Future Directions
The significant reliance on electrical energy for the progression of society has attracted tremendous research efforts on developing advanced energy storage systems. Making electrodes flexible for storing electricity offers unique advantages including much higher energy and power densities, increased cycle life, lower cost and better manufacturability. Furthermore, many of the flexible electrodes being developed are free from current collector and binder and thus could dramatically reduce the total weight of the electrodes. Flexible energy storage systems are currently undergoing exciting developments, as new materials, composites and large-scale assembly strategies are being developed. Depending on the active materials being used, the flexible electrodes can be used in either supercapacitors or lithium-ion batteries, where improved energy storage metrics have been successfully demonstrated.
Flexible substrates (such as paper, textiles and plastics) were widely used in the fabrication of flexible electrodes and active materials were coated mostly through a dip-coating or electrodeposition route. Meanwhile, flexible electrodes can also be fabricated without using any mechanical support by taking the advantage of freestanding networks formed by interconnected CNTs and/or graphene. Electrodes made using the latter approach could have higher electrode-level energy and energy densities as the weight percentage of active materials were dramatically increased and therefore it is likely that such type of approaches will attract more emphasis for future developments, in which case further understandings regarding the rational assembly of porous, lightweight and highly conductive carbon matric are critical. It is also evident that most of the previous works were focused on single electrode design and hence additional work is also needed to fabricate and evaluate complete cells with both positive and negative electrodes integrated. Furthermore, very little efforts were devoted to study the self-discharge behavior associated with flexible electrodes even though self-discharge is one of the most critical performance metric and several pervious works have shown that supercapacitors generally have much higher self-discharge rate than batteries. It is thus evident that more fundamental work is needed to fully understand the reasons for their self-discharge behavior and to design electrodes with minimal self-discharge rate.
As has been discussed in this review, many strategies have been developed and shown promise toward the fabrication of flexible energy storage devices. In these studies, however, electrodes were usually fabricated with different configurations (such as architecture, active material loadings, thickness, etc.) and tested with different setups (two vs. three electrodes, different voltage ranges and electrolytes). As a consequence, it is generally difficult or even impossible to directly compare results obtained from different studies. This is further complicated by the fact that the reported energy density and power density were usually calculated using different approaches (for example, based on the active material only or whole electrodes). Hence, it is necessary to develop clear rules to report and evaluate the performance of flexible electrodes in a consistent manner, by doing which results published by different studies could be more comparable. For example, reporting two sets of energy and power densities, one set based on the total weight of active materials and the other set based on the weight of whole electrodes, both calculated using results from the two-electrode measurement method. Additionally, protocols to study the flexibility of the electrodes are also required, as mechanical strength is an important factor in determining the performance of flexible devices. Nevertheless, flexible devices hold great promise for a number of new applications, which are incompatible with conventional contemporary battery and supercapacitor technologies.
Acknowledgements
Financial support from Army Research Office (ARO) under contract W911NF-04-D-0001, National Science Foundation (NSF) and the Environmental Protection Agency (EPA) under NSF Cooperative Agreement EF-0830093, Center for the Environmental Implications of NanoTechnology (CEINT). Any opinions, findings, conclusions or recommendations expressed in this material are those of the author(s) and do not necessarily reflect the views of the ARO, NSF or the EPA. This work has not been subjected to EPA review and no official endorsement should be inferred.
References
- Yang Z G Zhang J L Kintner-Meyer M CW Lu X C Choi D W Lemmon J P Liu JElectrochemical energy storage for green grid. Chem Rev. 2011;111(5):3577–3613 (doi:10.1021/cr100290v)
- Holditch S A Chianelli R RFactors that will influence oil and gas supply and demand in the 21st century. Mrs Bull. 2008;33(4):317–323 (doi:10.1557/mrs2008.65)
- Facing the hard truths about energy–a comprehensive view to 2030 of global oil and natural gas. Washington, DC: National Petrolenum Council; 2007.
- Dillon A CCarbon nanotubes for photoconversion and electrical energy storage. Chem Rev. 2010;110(11):6856–6872 (doi:10.1021/cr9003314)
- Lund H Kempton WIntegration of renewable energy into the transport and electricity sectors through V2G. Energ Policy. 2008;36(9):3578–3587 (doi:10.1016/j.enpol.2008.06.007)
- Vazquez S Lukic S M Galvan E Franquelo L G Carrasco J MEnergy storage systems for transport and grid applications. Ieee T Ind Electron. 2010;3881–3895(12
- Hall P J Bain E JEnergy-storage technologies and electricity generation. Energ Policy. 2008;36(12):4352–4355 (doi:10.1016/j.enpol.2008.09.037)
- Khaligh A Li Z HBattery, ultracapacitor, fuel cell, and hybrid energy storage systems for electric, hybrid electric, fuel cell, and plug-in hybrid electric vehicles: state of the art. Ieee T Veh Technol. 2010;59(6):2806–2814 (doi:10.1109/TVT.2010.2047877)
- Baker JNew technology and possible advances in energy storage. Energ Policy. 2008;36(12):4368–4373
- Wang H Dai HStrongly coupled inorganic-nano-carbon hybrid materials for energy storage. Chem Soc Rev. 2013;42(7):3088–3113
- Yan J Fan Z J Wei T Qian W Z Zhang M L Wei FFast and reversible surface redox reaction of graphene-MnO2 composites as supercapacitor electrodes. Carbon. 2010;48(13):3825–3833 (doi:10.1016/j.carbon.2010.06.047)
- Athouel L Moser F Dugas R Crosnier O Belanger D Brousse TVariation of the MnO2 birnessite structure upon charge/discharge in an electrochemical supercapacitor electrode in aqueous Na2SO4 electrolyte. J Phys Chem C. 2008;112(18):7270–7277 (doi:10.1021/jp0773029)
- Gogotsi Y Simon PTrue performance metrics in electrochemical energy storage. Science. 2011;334(6058):917–918 (doi:10.1126/science.1213003)
- Cheng Y W Lu S T Zhang H B Varanasi C V Liu JSynergistic effects from graphene and carbon nanotubes enable flexible and robust electrodes for high-performance supercapacitors. Nano Lett. 2012;12(8):4206–4211 (doi:10.1021/nl301804c)
- Cheng Y W Zhang H B Lu S T Varanasiad C V Liu JFlexible asymmetric supercapacitors with high energy and high power density in aqueous electrolytes. Nanoscale. 2013;5(3):1067–1073 (doi:10.1039/c2nr33136e)
- Jost K Perez C R McDonough J K Presser V Heon M Dion G Gogotsi YCarbon coated textiles for flexible energy storage. Energ Environ Sci. 2011;4(12):5060–5067 (doi:10.1039/c1ee02421c)
- Dikin D A Stankovich S Zimney E J Piner R D Dommett G HB Evmenenko G Nguyen S T Ruoff R SPreparation and characterization of graphene oxide paper. Nature. 2007;448(7152):457–460 (doi:10.1038/nature06016)
- Izadi-Najafabadi A Yamada T Futaba D N Yudasaka M Takagi H Hatori H Iijima S Hata KHigh-power supercapacitor electrodes from single-walled carbon nanohorn/nanotube composite. Acs Nano. 2011;5(2):811–819 (doi:10.1021/nn1017457)
- Zhao X Hayner C M Kung M C Kung H HFlexible holey graphene paper electrodes with enhanced rate capability for energy storage applications. Acs Nano. 2011;5(11):8739–8749 (doi:10.1021/nn202710s)
- Yang X W Zhu J W Qiu L Li DBioinspired effective prevention of restacking in multilayered graphene films: towards the next generation of high-performance supercapacitors. Adv Mater. 2011;23(25):2833–2838 (doi:10.1002/adma.201100261)
- Wang H L Liang Y Y Mirfakhrai T Chen Z Casalongue H S Dai H JAdvanced asymmetrical supercapacitors based on graphene hybrid materials Nano Res2011;4(8):729–736
- Frackowiak E Beguin FElectrochemical storage of energy in carbon nanotubes and nanostructured carbons. Carbon. 2002;40(10):1775–1787
- Pumera MGraphene-based nanomaterials for energy storage. Energ Environ Sci. 2011;4(3):668–674 (doi:10.1039/c0ee00295j)
- Whitten P G Spinks G M Wallace G GMechanical properties of carbon nanotube paper in ionic liquid and aqueous electrolytes. Carbon. 2005;43(9):1891–1896 (doi:10.1016/j.carbon.2005.02.038)
- Landi B J Ganter M J Cress C D DiLeo R A Raffaelle R PCarbon nanotubes for lithium ion batteries. Energ Environ Sci. 2009;2(6):638–654 (doi:10.1039/b904116h)
- Ci L J Manikoth S M Li X S Vajtai R Ajayan P MUltrathick freestanding aligned carbon nanotube films. Adv Mater. 2007;19(20):3300–3303 (doi:10.1002/adma.200602974)
- Rinzler A G Liu J Dai H Nikolaev P Huffman C B Rodriguez-Macias F J Boul P J Lu A H Heymann D Colbert D T Lee R S Fischer J E Rao A M Eklund P C Smalley R ELarge-scale purification of single-wall carbon nanotubes: process, product, and characterization. Appl Phys a-Mater. 1998;67(1):29–37 (doi:10.1007/s003390050734)
- Endo M Muramatsu H Hayashi T Kim Y A Terrones M Dresselhaus N S‘Buckypaper’ from coaxial nanotubes. Nature. 2005;433(7025):476–476 (doi:10.1038/433476a)
- Whitby R LD Fukuda T Maekawa T James S L Mikhalovsky S VGeometric control and tuneable pore size distribution of buckypaper and buckydiscs. Carbon. 2008;46(6):949–956 (doi:10.1016/j.carbon.2008.02.028)
- Wang D Song P C Liu C H Wu W Fan S SHighly oriented carbon nanotube papers made of aligned carbon nanotubes. Nanotechnology. 2008;19(7):075609-1–6
- Peigney A Laurent C Flahaut E Bacsa R R Rousset ASpecific surface area of carbon nanotubes and bundles of carbon nanotubes. Carbon. 2001;39(4):507–514 (doi:10.1016/S0008-6223(00)00155-X)
- Niu C M Sichel E K Hoch R Moy D Tennent HHigh power electrochemical capacitors based on carbon nanotube electrodes. Appl Phys Lett. 1997;70(11):1480–1482 (doi:10.1063/1.118568)
- Niu Z Q Zhou W Y Chen J Feng G X Li H Ma W J Li J Z Dong H B Ren Y Zhao D A Xie S SCompact-designed supercapacitors using free-standing single-walled carbon nanotube films. Energ Environ Sci. 2011;4(4):1440–1446 (doi:10.1039/c0ee00261e)
- Lee S H Kim H W Hwang J O Lee W J Kwon J Bielawski C W Ruoff R S Kim S OThree-dimensional self-assembly of graphene oxide platelets into mechanically flexible macroporous carbon films. Angew Chem Int Edit. 2010;49(52):10084–10088 (doi:10.1002/anie.201006240)
- Niu Z Q Chen J Hng H H Ma J Chen X DA leavening strategy to prepare reduced graphene oxide foams. Adv Mater. 2012;24(30):4144–4150 (doi:10.1002/adma.201200197)
- Choi B G Yang M Hong W H Choi J W Huh Y S3D Macroporous graphene frameworks for supercapacitors with high energy and power densities. Acs Nano. 2012;6(5):4020–4028 (doi:10.1021/nn3003345)
- Lu X J Dou H Gao B Yuan C Z Yang S D Hao L Shen L F Zhang X GA flexible graphene/multiwalled carbon nanotube film as a high performance electrode material for supercapacitors. Electrochim Acta. 2011;56(14):5115–5121 (doi:10.1016/j.electacta.2011.03.066)
- Xu G H Zheng C Zhang Q Huang J Q Zhao M Q Nie J Q Wang X H Wei FBinder-free activated carbon/carbon nanotube paper electrodes for use in supercapacitors. Nano Res. 2011;4(9):870–881 (doi:10.1007/s12274-011-0143-8)
- Hu L B Choi J W Yang Y Jeong S La Mantia F Cui L F Cui YHighly conductive paper for energy-storage devices. P Natl Acad Sci USA. 2009;106(51):21490–21494 (doi:10.1073/pnas.0908858106)
- Futaba D N Hata K Yamada T Hiraoka T Hayamizu Y Kakudate Y Tanaike O Hatori H Yumura M Iijima SShape-engineerable and highly densely packed single-walled carbon nanotubes and their application as super-capacitor electrodes. Nat Mater. 2006;5(12):987–994 (doi:10.1038/nmat1782)
- Frackowiak E Beguin FCarbon materials for the electrochemical storage of energy in capacitors. Carbon. 2001;39(6):937–950 (doi:10.1016/S0008-6223(00)00183-4)
- Zhang L L Zhao X SCarbon-based materials as supercapacitor electrodes. Chem Soc Rev. 2009;38(9):2520–2531 (doi:10.1039/b813846j)
- Chen H Roy A Baek J B Zhu L Qu J Dai L MControlled growth and modification of vertically-aligned carbon nanotubes for multifunctional applications. Mat Sci Eng R. 2010;70(3–6):63–91 (doi:10.1016/j.mser.2010.06.003)
- Zhang M Fang S L Zakhidov A A Lee S B Aliev A E Williams C D Atkinson K R Baughman R H.Strong transparent, multifunctional, carbon nanotube sheets. Science. 2005;309(5738):1215–1219 (doi:10.1126/science.1115311)
- Ma W J Song L Yang R Zhang T H Zhao Y C Sun L F Ren Y Liu D F Liu L F Shen J Zhang Z X Xiang Y J Zhou W Y Xie S SDirectly synthesized strong, highly conducting, transparent single-walled carbon nanotube films. Nano Lett. 2007;7(8):2307–2311 (doi:10.1021/nl070915c)
- An K H Kim W S Park Y S Moon J M Bae D J Lim S C Lee Y S Lee Y HElectrochemical properties of high-power supercapacitors using single-walled carbon nanotube electrodes. Adv Funct Mater. 2001;11(5):387–392 (doi:10.1002/1616-3028(200110)11:5<387::AID-ADFM387>3.0.CO;2-G)
- Pan H Li J Y Feng Y PCarbon nanotubes for supercapacitor. Nanoscale Res Lett. 2010;5(3):654–668 (doi:10.1007/s11671-009-9508-2)
- Kang Y J Chun S J Lee S S Kim B Y Kim J H Chung H Lee S Y Kim WAll-solid-state flexible supercapacitors fabricated with bacterial nanocellulose papers, carbon nanotubes, and triblock-copolymer ion gels. Acs Nano. 2012;6(7):6400–6406 (doi:10.1021/nn301971r)
- Roy S Bajpai R Soin N Bajpai P Hazra K S Kulshrestha N Roy S S McLaughlin J A Misra D SEnhanced field emission and improved supercapacitor obtained from plasma-modified bucky paper. Small. 2011;7(5):688–693 (doi:10.1002/smll.201002330)
- Ng S H Wang J Guo Z P Wang G X Liu H KSingle wall carbon nanotube paper as anode for lithium-ion battery. Electrochim Acta. 2005;51(1):23–28 (doi:10.1016/j.electacta.2005.04.045)
- Landi B J Ganter M J Schauerman C M Cress C D Raffaelle R PLithium ion capacity of single wall carbon nanotube paper electrodes. J Phys Chem C. 2008;112(19):7509–7515 (doi:10.1021/jp710921k)
- Chew S Y Ng S H Wang J Z Novak P Krumeich F Chou S L Chen J Liu H KFlexible free-standing carbon nanotube films for model lithium-ion batteries. arbon. 2009;47(13):2976–2983
- Geim A K Novoselov K SThe rise of graphene. Nat Mater. 2007;6(3):183–191 (doi:10.1038/nmat1849)
- Stankovich S Dikin D A Piner R D Kohlhaas K A Kleinhammes A Jia Y Wu Y Nguyen S T Ruoff R SSynthesis of graphene-based nanosheets via chemical reduction of exfoliated graphite oxide. Carbon. 2007;45(7):1558–1565 (doi:10.1016/j.carbon.2007.02.034)
- Dreyer D R Park S Bielawski C W Ruoff R SThe chemistry of graphene oxide. Chem Soc Rev. 2010;39(1):228–240 (doi:10.1039/b917103g)
- Yu A P Roes I Davies A Chen Z WUltrathin, transparent, and flexible graphene films for supercapacitor application. Appl Phys Lett. 2010;96(25):253105-1–3 (doi:10.1063/1.3455879)
- Park S Lee K S Bozoklu G Cai W Nguyen S T Ruoff’ R SGraphene oxide papers modified by divalent ions – enhancing mechanical properties via chemical cross-linking. Acs Nano. 2008;2(3):572–578 (doi:10.1021/nn700349a)
- Moon I K Lee J Ruoff R S Lee HReduced graphene oxide by chemical graphitization. Nat Commun. 2010;1(73):1–6
- Si Y C Samulski E TExfoliated graphene separated by platinum nanoparticles. Chem Mater. 2008;20(21):6792–6797 (doi:10.1021/cm801356a)
- Wang G K Sun X Lu F Y Sun H T Yu M P Jiang W L Liu C S Lian JFlexible pillared graphene-paper electrodes for high-performance electrochemical supercapacitors. Small. 2012;8(3):452–459 (doi:10.1002/smll.201101719)
- Xu Y Lin Z Huang X Liu Y Huang Y Duan XFlexible solid-state supercapacitors based on three-dimensional graphene hydrogel films. Acs Nano. 2013;7(5):4042–4049 (doi:10.1021/nn4000836)
- Su Q Liang Y Y Feng X L Mullen KTowards free-standing graphene/carbon nanotube composite films via acetylene-assisted thermolysis of organocobalt functionalized graphene sheets. Chem Commun. 2010;46(43):8279–8281 (doi:10.1039/c0cc02659j)
- Zhang C Ren L L Wang X Y Liu T XGraphene oxide-assisted dispersion of pristine multiwalled carbon nanotubes in aqueous media. J Phys Chem C. 2010;114(26):11435–11440 (doi:10.1021/jp103745g)
- Yang S Y Chang K H Tien H W Lee Y F Li S M Wang Y S Wang J Y Ma C CM Hu C CDesign and tailoring of a hierarchical graphene-carbon nanotube architecture for supercapacitors. J Mater Chem. 2011;21(7):2374–2380 (doi:10.1039/c0jm03199b)
- Gamby J Taberna P L Simon P Fauvarque J F Chesneau MStudies and characterisations of various activated carbons used for carbon/carbon supercapacitors. J Power Sources. 2001;101(1):109–116 (doi:10.1016/S0378-7753(01)00707-8)
- Smithyman J Moench A Liang R Zheng J P Wang B Zhang CBinder-free composite electrodes using carbon nanotube networks as a host matrix for activated carbon microparticles. Appl Phys a-Mater. 2012;107(3):723–731 (doi:10.1007/s00339-012-6790-0)
- Hu L B Wu H Cui YPrinted energy storage devices by integration of electrodes and separators into single sheets of paper. Appl Phys Lett. 2010;96(18):183502-1–3 (doi:10.1063/1.3425767)
- Zheng G Y Hu L B Wu H Xie X Cui YPaper supercapacitors by a solvent-free drawing method. Energ Environ Sci. 2011;4(9):3368–3373 (doi:10.1039/c1ee01853a)
- Hu L B Cui YEnergy and environmental nanotechnology in conductive paper and textiles. Energ Environ Sci. 2012;5(4):6423–6435 (doi:10.1039/c2ee02414d)
- Yu G H Hu L B Liu N A Wang H L Vosgueritchian M Yang Y Cui Y Bao Z AEnhancing the supercapacitor performance of graphene/MnO2 nanostructured electrodes by conductive wrapping. Nano Lett. 2011;11(10):4438–4442 (doi:10.1021/nl2026635)
- Chen W Rakhi R B Hu L B Xie X Cui Y Alshareef H NHigh-performance nanostructured supercapacitors on a sponge. Nano Lett. 2011;11(12):5165–5172 (doi:10.1021/nl2023433)
- Hu L B Pasta M La Mantia F Cui L F Jeong S Deshazer H D Choi J W Han S M Cui YStretchable, porous, and conductive energy textiles. Nano Lett. 2010;10(2):708–714 (doi:10.1021/nl903949m)
- Yu G H Xie X Pan L J Bao Z N Cui YHybrid nanostructured materials for high-performance electrochemical capacitors. Nano Energy. 2012;2(2):213–234 (doi:10.1016/j.nanoen.2012.10.006)
- Weng Z Su Y Wang D W Li F Du J H Cheng H MGraphene-cellulose paper flexible supercapacitors. Adv Energy Mater. 2011;1(5):917–922 (doi:10.1002/aenm.201100312)
- Kaempgen M Chan C K Ma J Cui Y Gruner GPrintable thin film supercapacitors using single-walled carbon nanotubes. Nano Lett. 2009;9(5):1872–1876 (doi:10.1021/nl8038579)
- Choi B G Hong J Hong W H Hammond P T Park HFacilitated ion transport in all-solid-state flexible supercapacitors. Acs Nano. 2011;5(9):7205–7213 (doi:10.1021/nn202020w)
- Long J W Belanger D Brousse T Sugimoto W Sassin M B Crosnier OAsymmetric electrochemical capacitors-stretching the limits of aqueous electrolytes. Mrs Bull. 2011;36(7):513–522 (doi:10.1557/mrs.2011.137)
- Hou Y Cheng Y W Hobson T Liu JDesign and synthesis of hierarchical MnO2 nanospheres/carbon nanotubes/conducting polymer ternary composite for high performance electrochemical electrodes. Nano Lett. 2010;10(7):2727–2733 (doi:10.1021/nl101723g)
- Nyholm L Nystrom G Mihranyan A Stromme MToward flexible polymer and paper-based energy storage devices. Adv Mater. 2011;23(33):3751–3769
- Snook G A Kao P Best A SConducting-polymer-based supercapacitor devices and electrodes. J Power Sources. 2011;196(1):1–12 (doi:10.1016/j.jpowsour.2010.06.084)
- Wang G P Zhang L Zhang J JA review of electrode materials for electrochemical supercapacitors. Chem Soc Rev. 2012;41(2):797–828 (doi:10.1039/c1cs15060j)
- Xu C J Kang F Y Li B H Du H DRecent progress on manganese dioxide based supercapacitors. J Mater Res. 2010;25(8):1421–1432 (doi:10.1557/JMR.2010.0211)
- Lu X Zhai T Zhang X Shen Y Yuan L Hu B Gong L Chen J Gao Y Zhou J Tong Y Wang Z LWO3-x@Au@MnO2 core-shell nanowires on carbon fabric for high-performance flexible supercapacitors. Adv Mater. 2012;24(7):938–944 (doi:10.1002/adma.201104113)
- Broughton J N Brett M JVariations in MnO2 electrodeposition for electrochemical capacitors. Electrochim Acta. 2005;50(24):4814–4819 (doi:10.1016/j.electacta.2005.03.006)
- Wei W F Cui X W Chen W X Ivey D GPhase-controlled synthesis of MnO2 nanocrystals by anodic electrodeposition: implications for high-rate capability electrochemical supercapacitors. J Phys Chem C. 2008;112(38):15075–15083 (doi:10.1021/jp804044s)
- Kang Y J Kim B Chung H Kim WFabrication and characterization of flexible and high capacitance supercapacitors based on MnO2/CNT/papers. Synthetic Met. 2010;160(23-24):2510–2514
- Hu L B Chen W Xie X Liu N A Yang Y Wu H Yao YPasta M, Alshareef HN, Cui Y. Symmetrical MnO2-carbon nanotube-textile nanostructures for wearable pseudocapacitors with high mass loading. Acs Nano. 2011;5(11):8904–8913 (doi:10.1021/nn203085j)
- Yu G H Hu L B Vosgueritchian M Wang H L Xie X McDonough J R Cui X Cui Y Bao Z NSolution-processed graphene/MnO2 nanostructured textiles for high-performance electrochemical capacitors. Nano Lett. 2011;11(7):2905–2911 (doi:10.1021/nl2013828)
- Bao L H Li X DTowards textile energy storage from cotton T-shirts. Adv Mater. 2012;24(24):3246–3252 (doi:10.1002/adma.201200246)
- Chen Y C Hsu Y K Lin Y G Lin Y K Horng Y Y Chen L C Chen K HHighly flexible supercapacitors with manganese oxide nanosheet/carbon cloth electrode. Electrochim Acta. 2011;56(20):7124–7130 (doi:10.1016/j.electacta.2011.05.090)
- Yuan L Y Lu X H Xiao X Zhai T Dai J J Zhang F C Hu B Wang X Gong L Chen J Hu C G Tong Y X Zhou J Wang Z LFlexible solid-state supercapacitors based on carbon nanoparticles/MnO2 nanorods hybrid structure. Acs Nano. 2012;6(1):656–661 (doi:10.1021/nn2041279)
- Bao L H Zang J F Li X DFlexible Zn2SnO4/MnO2 core/shell nanocable-carbon microfiber hybrid composites for high-performance supercapacitor electrodes. Nano Lett. 2011;11(3):1215–1220 (doi:10.1021/nl104205s)
- Li Z P Mi Y J Liu X H Liu S Yang S R Wang J QFlexible graphene/MnO2 composite papers for supercapacitor electrodes. J Mater Chem. 2011;21(38):14706–14711 (doi:10.1039/c1jm11941a)
- Chou S L Wang J Z Chew S Y Liu H K Dou S XElectrodeposition of MnO2 nanowires on carbon nanotube paper as free-standing, flexible electrode for supercapacitors. Electrochem Commun. 2008;10(11):1724–1727 (doi:10.1016/j.elecom.2008.08.051)
- He Y Chen W Li X Zhang Z Fu J Zhao C Xie EFreestanding three-dimensional graphene/MnO2 composite networks as ultra light and flexible supercapacitor electrodes. Acs Nano. 2013;7(1):174–182 (doi:10.1021/nn304833s)
- Yuan C Z Hou L R Li D K Shen L F Zhang F Zhang X GSynthesis of flexible and porous cobalt hydroxide/conductive cotton textile sheet and its application in electrochemical capacitors. Electrochim Acta. 2011;56(19):6683–6687 (doi:10.1016/j.electacta.2011.05.050)
- Boukhalfa S Evanoff K Yushin GAtomic layer deposition of vanadium oxide on carbon nanotubes for high-power supercapacitor electrodes. Energ Environ Sci. 2012;5(5):6872–6879 (doi:10.1039/c2ee21110f)
- Zhang X J Shi W H Zhu J X Kharistal D J Zhao W Y Lalia B S Hng H H Yan Q YHigh-power and high-energy-density flexible pseudocapacitor electrodes made from porous CuO nanobelts and single-walled carbon nanotubes. Acs Nano. 2011;5(3):2013–2019 (doi:10.1021/nn1030719)
- Yang P Xiao X Li Y Ding Y Qiang P Tan X Mai W Lin Z Wu W Li T Jin H Liu P Zhou J Wong C P Wang Z LHydrogenated ZnO Core-shell nanocables for flexible supercapacitors and self-powered systems. Acs Nano. 2013;7(3):2617–2626 (doi:10.1021/nn306044d)
- Chou S L Wang J Z Liu H K Dou S XElectrochemical deposition of porous Co(OH)(2) nanoflake films on stainless steel mesh for flexible supercapacitors. J Electrochem Soc. 2008;155(12):A926–A929 (doi:10.1149/1.2988739)
- Perera S D Patel B Nijem N Roodenko K Seitz O Ferraris J P Chabal Y J Balkus K JVanadium oxide nanowire-carbon nanotube binder-free flexible electrodes for supercapacitors. Adv Energy Mater. 2011;1(5):936–945 (doi:10.1002/aenm.201100221)
- Chen P C Shen G Z Shi Y Chen H T Zhou C WPreparation and characterization of flexible asymmetric supercapacitors based on transition-metal-oxide nanowire/single-walled carbon nanotube hybrid thin-film electrodes. Acs Nano. 2010;4(8):4403–4411 (doi:10.1021/nn100856y)
- Wu Z S Ren W C Wang D W Li F Liu B L Cheng H MHigh-energy MnO2 nanowire/graphene and graphene asymmetric electrochemical capacitors. Acs Nano. 2010;4(10):5835–5842 (doi:10.1021/nn101754k)
- Shao Y L Wang H Z Zhang Q H Li Y GHigh-performance flexible asymmetric supercapacitors based on 3D porous graphene/MnO2 nanorod and graphene/Ag hybrid thin-film electrodes. J Mater Chem C. 2013;1(6):1245–1251 (doi:10.1039/c2tc00235c)
- Lu X H Yu M H Wang G M Zhai T Xie S L Ling Y C Tong Y X Li YH-TiO2@MnO2//H-TiO2@C core-shell nanowires for high performance and flexible asymmetric supercapacitors. Adv Mater. 2013;25(2):267–272 (doi:10.1002/adma.201203410)
- Choi B G Chang S J Kang H W Park C P Kim H J Hong W H Lee S Huh Y SHigh performance of a solid-state flexible asymmetric supercapacitor based on graphene films. Nanoscale. 2012;4(16):4983–4988 (doi:10.1039/c2nr30991b)