ABSTRACT
Nd–Fe–B sintered magnets with a giant intrinsic coercivity of 47.1 kOe and a high maximum energy product of 38.5 MGOe have been prepared via a combination of conventional sintering techniques and novel grain boundary diffusion process. Microstructural observations show that the Nd2Fe14B main phase grains in the magnets exhibit a core/shell type rare-earth elements distribution, where Tb enriched (Nd,Tb)2Fe14B phases are formed in the surface regions of the grains. Magnetic domain observations indicate that these modified surface regions strongly restrain the nucleation process of reversed magnetic domains, leading to a remarkable enhancement of the coercivity and overall magnetic performance of the magnets.
Impact statement
Nd–Fe–B sintered magnets with an unprecedented overall magnetic property have been fabricated with a novel approach, and the origin of magnetic property enhancement was elaborated via magnetic domain observation.
GRAPHICAL ABSTRACT
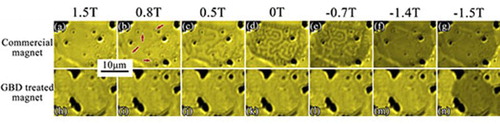
1. Introduction
In recent years, the rapid development of some green-energy technologies, such as electric transportation and wind turbines, has led to the growing demand for Nd–Fe–B sintered magnets bearing both high maximum energy product [(BH)max] and high intrinsic coercivity (Hci) [Citation1–3]. The traditional method for enhancing the coercivity of Nd–Fe–B sintered magnets is to partially substitute Nd with heavy rare earth, such as Dy or Tb, to increase the anisotropy field of the magnets [Citation4–7]. However, the increase in Hci occurs at the expense of the remarkable decrease of (BH)max due to the ferrimagnetic characteristic of (Dy/Tb)2Fe14B compounds [Citation7]. In 2000, Park et al. proposed a promising way of grain boundary diffusion (GBD) to enhance the coercivity of thin Nd–Fe–B sintered magnets [Citation8]. Lately, several methods focusing on grain boundary modification (GBM), i.e. concentrating Dy or Tb in the surface regions of the Nd2Fe14B main phase grains, have been developed [Citation9–18]. By annealing Nd–Fe–B sintered magnets, coated with micron-size TbF3 particles, Nakamura et al. [Citation9] achieved a coercivity enhancement from 0.9 to 1.7 MA/m in the magnets without sacrificing their remanence. Furthermore, their observations indicate that Tb diffuses into the magnets along the grain boundary phase and segregates near the surface regions of the grains. Watanabe et al. [Citation14] sputtered a thin Tb layer on the surface of Nd–Fe–B sintered magnets, and using a similar GBD technique, they improved the coercivity of the magnets from 0.95 to 1.98 MA/m with a slight decrease, of 0.01 T, in the remanence. Apart from GBD, Yue et al. [Citation18] introduced a new approach to achieve GBM. They doped Tb nanoparticles with Nd–Fe–B powders to prepare Nd–Fe–B sintered magnets, with Tb-enriched surface regions of Nd2Fe14B grains after sintering. As a result, the magnets exhibit remarkable improvement in coercivity without any obvious decreases in remanence and maximum energy product.
Based upon above approaches, one can obtain new Nd–Fe–B sintered magnets with both high Hci and high (BH)max, which could have a wider application field [Citation19–21]. Moreover, the new magnets, containing less Dy or Tb, will have low fabrication costs compared with the present Nd–Fe–B sintered magnets. Up to now, however, the specific magnetic hardening mechanism of magnets with GBM is still unclear. More investigations to the micromagnetic structure of the magnets are necessary to understand the nature of the coercivity enhancement [Citation22,Citation23]. In this paper, we describe a novel approach to develop Nd–Fe–B sintered magnets with (BH)max of 38.5 MGOe and Hci of 47.1 kOe, leading to a record high overall magnetic performance (OMP) [Citation19] of (BH)max (MGOe) + Hci (kOe) = 85.6. These superior magnetic properties, in particular the giant coercivity, enable Nd–Fe–B sintered magnets to compete for wider applications at an elevated temperature. The microstructure and magnetic domain of the magnets were also investigated to understand the origin of this high performance.
2. Experiments
Nd–Fe–B sintered magnets with a commercial grade of 40TH (the magnetic properties were shown in ), prepared by conventional sintering techniques. The magnets were cut into 10 mm × 10 mm × 4 mm thin slices and coated with TbH3 nanoparticles by dipping the slices into a TbH3 nanoparticles solution. This solution is a mixture of inert-gas condensed TbH3 nanoparticles and ethyl alcohol with a ratio of 1:1. The coated slices were dried in hot air, wrapped with nickel film and then heat-treated in vacuum at 940°C for 7 h followed by a further annealing at 500°C for 5 h. For comparison, TbH3 and TbF3 microparticles were also applied to the diffusion treatment under same conditions.
Figure 1. Demagnetization curves (solid line) of a high-performance Nd–Fe–B sintered magnet prepared with three kinds of particles (TbH3 nanoparticles, TbH3, and TbF3 microparticles) by GBD technique, and the demagnetization curve (dash line) of the pre-treated magnet was also shown for comparison. Inset table: magnetic properties of the four magnets.
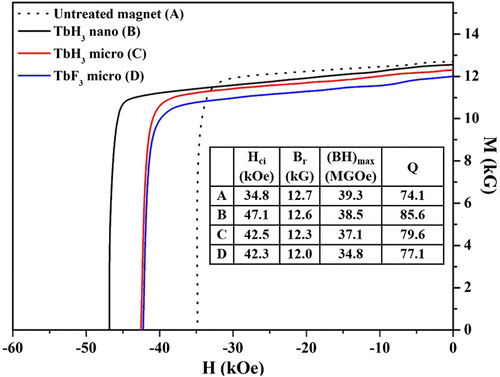
The microstructure of the magnets was studied using scanning electron microscope (SEM) with energy-dispersive X-ray spectroscopy and electron-probe micro-analyzer (EPMA) with a wavelength-dispersive X-ray detector. Magnetic domains and their evolution during the magnetization and demagnetization processes were observed by a magneto-optical Kerr optical microscope (MOKE). For comparison, Nd–Fe–B sintered magnets with a commercial grade of N52 [(BH)max ≈ 52 MGOe and Hci ≥ 12 kOe] were also observed with MOKE. The observation plane of the samples with SEM, EPMA, and MOKE was perpendicular to the c-axes direction of the magnets. Magnetic properties of the slice-like magnets were measured using a B–H hysteresis loop tracer. The magnets were slightly polished to remove a rough surface layer with thickness of tens of micrometers before magnetic measurement.
3. Results and discussion
shows the demagnetization curves (solid line) for a high-performance Nd–Fe–B sintered magnet prepared by the GBD technique with three kinds of Tb-containing particles, namely TbH3 nanoparticles, TbH3 microparticles, and TbF3 microparticles. Demagnetization curve (dash line) of the corresponding pre-treated magnet was shown together for comparison. It shows that all of the three treated magnets bear remarkably higher coercivity but slightly lower remanence than those of the pre-treated magnet. By contrast, TbH3 nanoparticles-treated magnets exhibit best properties: the maximum energy product and intrinsic coercivity of the magnet are 38.5 MGOe and 47.1 kOe, respectively, leading to an OMP of (BH)max (MGOe) + Hci (kOe) = 85.6. Compared with the pre-treated magnets, the coercivity of the magnet increases remarkably by 12.3 kOe (35.3%), and the energy product drops slightly by 0.8 MGOe (2.0%), leading to a value of the OMP increases by 11.5 (15.5%). To the best of our knowledge, the high OMP value exceeds the highest reported value in the literature for Nd–Fe–B sintered magnets, demonstrating the validity and feasibility of TbH3 nanoparticles GBD in preparing new Nd–Fe–B sintered magnets with both high Hci and high (BH)max.
(a) shows the microstructure of GBD-treated Nd–Fe–B sintered magnet with TbH3 nanoparticles. The observation plane is 200 µm beneath the surface of the magnet. The white Nd-rich phase, shown in (a), distributes homogeneously around the gray Nd2Fe14B matrix phase. Moreover, also shown in the figure, each Nd2Fe14B grain is composed of a dark-gray core area with a light-gray surface region. To clarify the distribution of the diffused Tb element in the Nd–Fe–B magnet, the concentrations of Tb elements in the same observation plane were examined, as shown in (b). It clearly shows that Tb elements are enriched mainly along the surface region of every Nd2Fe14B grains and form the (Nd,Tb)2Fe14B phase. A similar phenomenon was carefully investigated and reported for GBD process of Dy-vapor treated Nd–Fe–B sintered magnet [Citation24]. Further quantitative investigation of Tb distribution in the Nd-rich phase, Nd2Fe14B grain center area, and surface region indicates that little Tb exists in the Nd-rich phase, while the Tb concentration in the surface region of Nd2Fe14B grains is three to four times higher than that in the center of the Nd2Fe14B grains. In a recent first-principles study, Liu et al. found that Tb prefers to enter the Nd2Fe14B matrix phase rather than the Nd-rich phase when it was diffused into the Nd–Fe–B sintered magnet, showing the thermodynamic origin for the selective occupation of Tb in the magnet [Citation25]. Therefore, the enrichment of Tb was expected to result from the substitution of Nd by Tb atoms in the Nd2Fe14B compound during the sintering and subsequent heat treatment process.
Figure 2. EPMA micrograph of the GBD-treated Nd–Fe–B sintered magnet 200 µm beneath the surface of the magnet (a) and concentration distribution mapping of Tb in the corresponding position (b).
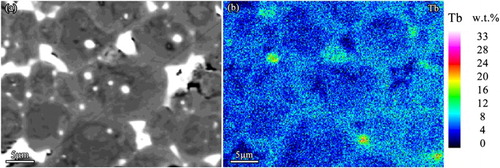
shows the concentration distribution of Tb in the GBD-treated Nd–Fe–B sintered magnets with TbH3 nanoparticles, TbH3 microparticles, and TbF3 microparticles. It shows that in all of the three magnets, Tb concentration decreases gradually from the surface (bottom) to the inside (top) of the magnets. For comparison, higher Tb concentration and longer diffusion distance were achieved simultaneously in the TbH3 nanoparticles-treated magnet, demonstrating the key advantage of nanoparticles in the GBD treatment. In detail, the smaller size of TbH3 nanoparticles makes their diffusion process along the grain boundary of Nd–Fe–B sintered magnets much easier than their micrometer-size counterpart, and the diffusion distance is also longer. Moreover, it is worth to note that terbium hydride possesses another advantage to terbium fluoride in the GBD treatment. That is, the hydrogen atoms in the particles release as hydrogen gas upon heating before the GBD process, so there will be no contamination of fluorine entering into the magnets with Tb. As a result, the undesirable magnetic dilution by fluorine, which will lead to considerable reduction of remanence and deterioration of squareness of the demagnetization curve, can be avoided. The above two advantages make TbH3 nanoparticles more desirable than TbH3 and TbF3 microparticles in the GBD treatment, as demonstrated by the corresponding magnetic performance shown in .
Figure 3. Concentration distribution mapping of Tb in the GBD-treated Nd–Fe–B sintered magnets with (a) TbH3 nanoparticles, (b) TbH3 microparticles, and (c) TbF3 microparticles, the bottom and the top of the figure corresponding the surface and inside of the magnets, respectively.
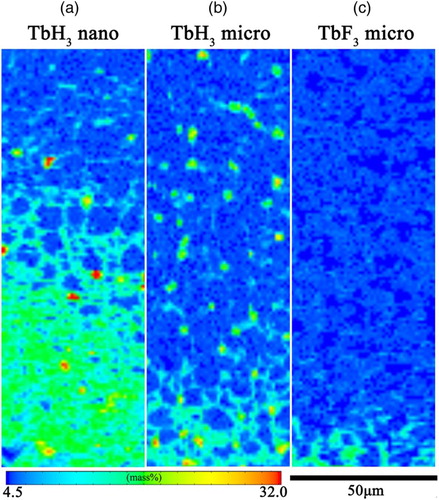
To understand the effect of the terbium GBD treatment to the coercivity enhancement of Nd–Fe–B sintered magnet, the magnetic domain reversal of fully magnetized GBD-treated Nd–Fe–B sintered magnet in the field from 1.5 T to −1.5 T was observed with Kerr microscopy. For comparison, the commercial Nd–Fe–B sintered magnet with low coercivity was investigated as well. At 1.5 T, both magnets exhibit fully magnetized states, i.e. single domains. As the field is reduced to 0 T, a maze domain pattern is found in some grains in the commercial magnet, as indicated by arrows in (b), indicating that the nucleation of reverse domains starts before the demagnetization process due to the demagnetization field. As the reverse field increases, the reverse domains expand gradually, as shown in (c). At −0.5 T, the first fully reversed grain was observed in the magnet, as indicated by the arrow in (d). As the reverse field increases to −1.5 T, most grains are now fully reversed. On the other hand, no reversed grains were observed in the GBD-treated Nd–Fe–B sintered magnet until the reverse filed increased to −0.4 T. The successful restraint of magnetic reversal of the grains before the remanent point is beneficial to high Mr/Ms ratio, leading to relatively high remanence in the magnet. At −0.5 T, several grains were suddenly reversed, as indicated by the arrows in (i). It is important to note that magnetic domain expansion cannot be observed in the suddenly reversed grains, suggesting that the nucleation field of a reversed domain is stronger than the field needed to overcome the drag force of domain wall motion. Even at −1.5 T, domain reversal was observed in only a few grains, while most of the grains kept their original magnetized state, indicating that the corresponding demagnetization process has not reached the coercive field. It is therefore concluded that the GBD treatment has a substantial influence on the magnetic domain reversal of Nd–Fe–B sintered magnets. As a result, the coercivity of the magnet was greatly enhanced. Moreover, it is worth to note that few misoriented grains can be observed in the magnets, although most grains are well aligned during the fabrication process. Such grains are found to keep distinct contrast during the reversal process, and example was circled with a blue square at the left bottom in (f).
Figure 4. MOKE images of magnetic domain evolution of the commercial Nd–Fe–B sintered magnet (upper row) and GBD-treated Nd–Fe–B sintered magnet (lower row) in the field of 1.5 T to −1.5 T.
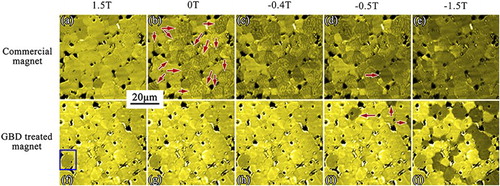
To gain a more detailed insight on the magnetic domain reversal process, we investigated the magnetic domain evolution of individual grains in both commercial and GBD-treated Nd–Fe–B sintered magnets, as shown in . For the commercial magnet, the grain is in a fully magnetized state at 1.5 T. As the field is reduced to 0.8 T, reversed domains were observed near the surface region of the grains, as indicated by the arrows in (b). We note that the magnetic reversal nucleation sites can seldom be found in our observation due to the time resolution of the equipment. As the field is reduced to zero, the reversed domains (dark pattern in the grain) gradually enlarge. Upon the application of a reverse field, the reversed domains finally approach their fully magnetized state once the reverse field reaches −1.4 T. On the other hand, the domain evolution process in the grains of GBD-treated magnet is significantly different. Although the grains also show a fully magnetized state at 1.5 T, it can keep that state even when the applied reverse field reaches −1.4 T. After this point, the magnetization of the entire grain reverses suddenly at −1.5 T without nucleation and expansion of the reverse domain. As the domain wall motion is expected to be fast under a magnetic field as high as 1.5 T, the duration of the whole magnetic reversal process of the grain is too fast to be captured with our equipment.
Figure 5. MOKE images of magnetic domain evolution of individual grain in the commercial Nd–Fe–B sintered magnet (upper row) and GBD-treated Nd–Fe–B sintered magnet (lower row) in the field of 1.5 T to −1.5 T.
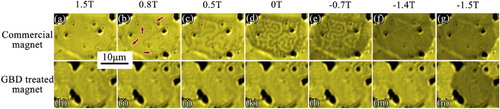
Based upon the above observations, it is clear that the magnetic domain reversal mode of GBD-treated Nd–Fe–B sintered magnet, namely the kinetics of the magnetic domain switching, undergoes significant changes compared to that of the commercial magnet. For the conventional Nd–Fe–B sintered magnet, the typical microstructure is composed of the Nd2Fe14B matrix phase and the Nd-rich grain boundary phase. The magnetic reversal of the magnet involves the magnetic domain nucleation followed by expansion. According to our previous study [Citation18], the Nd2Fe14B grain surface regions usually have low crystal anisotropy and are prone to act as nucleation sites, and once the reversed magnetic domain nucleation occurs, the reversed domains quickly expand via domain wall motion with the increase of reversed field, as is observed in (a)–(g). Therefore, the magnetic reversal nucleation field, HN, has a critical influence on the coercivity of the magnet. During the GBD process, the partial replacement of Nd by Tb takes place in the surface regions of the Nd2Fe14B grains by atomic diffusion, as shown in . Both our previous investigation [Citation18] and theoretical study by Liu et al. [Citation25] suggest that the Terbium enriched in the grain surface regions exists in the form of Tb2Fe14B and/or (Nd, Tb)2Fe14B phases. Since the HA of 22 T for the Tb2Fe14B compound is much higher than that (6.7 T) for the Nd2Fe14B compound [Citation4], the HN of the surface region is substantially enhanced, leading to a strong resistance to the nucleation of reverse domains. As a result, the GBD-treated Nd–Fe–B sintered magnets exhibit extra-high coercivity as well as a record-high OMP. The phenomenological illustrations to the magnetic domain switching kinetics shown in Figures and are beneficial to understand coercivity enhancement in GBD-treated Nd–Fe–B sintered magnets.
4. Conclusion
In summary, magnetic properties, structure, and magnetic domain evolution have been studied for novel Nd–Fe–B sintered magnets with a record high OMP prepared by conventional sintering and subsequent GBD treatment. A core/shell type rare-earth element distribution, i.e. Tb is enriched as (Nd,Tb)2Fe14B phase in the surface regions of the Nd2Fe14B grains was observed in these magnets. Furthermore, magnetic domain observations indicate that the modified surface regions have a substantial influence to the nucleation process of reversed magnetic domains, leading to the remarkable enhancement of the coercivity as well as to the OMP of the magnets.
Acknowledgement
MY is in debt to Prof. Z. Altounian for very helpful discussions.
Disclosure statement
No potential conflict of interest was reported by the authors.
Additional information
Funding
References
- Sagawa M, Fujimura S, Togawa N, et al. New material for permanent magnets on a base of Nd and Fe (invited). J Appl Phys. 1984;55(6):2083–2087. doi: 10.1063/1.333572
- Croat JJ, Herbst JF, Lee RW, et al. Pr-Fe and Nd-Fe-based materials: a new class of high-performance permanent magnets (invited). J Appl Phys. 1984;55(6):2078–2082. doi: 10.1063/1.333571
- Matsuura Y. Recent development of Nd–Fe–B sintered magnets and their applications. J Magn Magn Mater. 2006;303(2):344–347. doi: 10.1016/j.jmmm.2006.01.171
- Hirosawa S, Matsuura Y, Yamamoto H, et al. Magnetization and magnetic anisotropy of R2Fe14B measured on single crystals. J Appl Phys. 1986;59(3):873–879. doi: 10.1063/1.336611
- Koon NC, Das BN, Rubinstein M, et al. Magnetic properties of R2Fe14B single crystals. J Appl Phys. 1985;57(8):4091–4093. doi: 10.1063/1.334681
- Lileev AS, Ayuyan AG, Steiner W, et al. Low-temperature magnetization reversal processes in permanent magnets based on R(2)T(14)B. J Magn Magn Mater. 1996;157-158:373–375. doi: 10.1016/0304-8853(95)01209-5
- Boltich EB, Oswald E, Huang MQ, et al. Magnetic characteristics of R2Fe14B systems prepared with high purity rare earths (R = Ce, Pr, Dy, and Er). J Appl Phys. 1985;57(8):4106–4108. doi: 10.1063/1.334633
- Park KT, Hiraga K, Sagawa M. Effect of metal-coating and consecutive heat treatment on coercivity of thin Nd-Fe-B sintered magnets. Proceedings of the 16th Workshop Rare-Earth Magnets and Their Applications; 2000. p. 257–264.
- Nakamura H, Hirota K, Shimao M, et al. Magnetic properties of extremely small Nd-Fe-B sintered magnets. IEEE Trans Magn. 2005;41(10):3844–3846. doi: 10.1109/TMAG.2005.854874
- Hirota K, Nakamura H, Minowa T, et al. Coercivity enhancement by the grain boundary diffusion process to Nd–Fe–B sintered magnets. IEEE Trans Magn. 2006;42(10):2909–2911. doi: 10.1109/TMAG.2006.879906
- Suzuki H, Satsu Y, Komuro M. Magnetic properties of a Nd–Fe–B sintered magnet with Dy segregation. J Appl Phys. 2009;105(7):07A734. doi: 10.1063/1.3073830
- Soderžnik M, Rožman KŽ, Kobe S, et al. The grain-boundary diffusion process in Nd–Fe–B sintered magnets based on the electrophoretic deposition of DyF3. Intermetallics. 2012;23:158–162. doi: 10.1016/j.intermet.2011.11.014
- Popov AG, Vasilenko DY, Puzanova TZ, et al. Effect of diffusion annealing on the hysteretic properties of sintered Nd-Fe-B magnets. Phys Met Metallogr. 2011;111(5):471–478. doi: 10.1134/S0031918X11040107
- Watanabe N, Itakura M, Kuwano N, et al. Microstructure analysis of sintered Nd-Fe-B magnets improved by Tb-vapor sorption. Mater Trans. 2007;48(5):915–918. doi: 10.2320/matertrans.48.915
- Nakamura H, Hirota K, Ohashi T, et al. Coercivity distributions in Nd–Fe–B sintered magnets produced by the grain boundary diffusion process. J Phys D: Appl Phys. 2011;44(6):064003. doi: 10.1088/0022-3727/44/6/064003
- Li D, Suzuki S, Kawasaki T, et al. Grain interface modification and magnetic properties of Nd–Fe–B sintered magnets. Jpn J Appl Phys. 2008;47(10):7876–7878. doi: 10.1143/JJAP.47.7876
- Sepehri-Amin H, Ohkubo T, Hono K. Grain boundary structure and chemistry of Dy-diffusion processed Nd–Fe–B sintered magnets. J Appl Phys. 2010;107(9):09A745. doi: 10.1063/1.3351247
- Yue M, Liu WQ, Zhang DT, et al. Tb nanoparticles doped Nd–Fe–B sintered permanent magnet with enhanced coercivity. Appl Phys Lett. 2009;94(9):092501. doi: 10.1063/1.3093818
- Hu B-P, Niu E, Zhao Y-G, et al. Study of sintered Nd-Fe-B magnet with high performance of Hcj (kOe) + (BH)max (MGOe) > 75. AIP Adv. 2013;3(4):042136. doi: 10.1063/1.4803657
- Sepehri-Amin H, Ohkubo T, Hono K. The mechanism of coercivity enhancement by the grain boundary diffusion process of Nd–Fe–B sintered magnets. Acta Mater. 2013;61(6):1982–1990. doi: 10.1016/j.actamat.2012.12.018
- Hono K, Sepehri-Amin H. Strategy for high-coercivity Nd–Fe–B magnets. Scr Mater. 2012;67(6):530–535. doi: 10.1016/j.scriptamat.2012.06.038
- Kobayashi K, Urushibata K, Matsushita T, et al. Magnetic properties and domain structures in Nd–Fe–B sintered magnets with Tb additive reacted and diffused from the sample surface. J Alloys Compd. 2014;615:569–575. doi: 10.1016/j.jallcom.2014.06.170
- Helbig T, Loewe K, Sawatzki S, et al. Experimental and computational analysis of magnetization reversal in (Nd,Dy)-Fe-B core shell sintered magnets. Acta Mater. 2017;127:498–504. doi: 10.1016/j.actamat.2017.01.055
- Seelam UMR, Ohkubo T, Abe T, et al. Faceted shell structure in grain boundary diffusion-processed sintered Nd–Fe–B magnets. J Alloys Compd. 2014;617:884–892. doi: 10.1016/j.jallcom.2014.07.166
- Liu XB, Altounian Z. The partitioning of Dy and Tb in NdFeB magnets: a first-principles study. J Appl Phys. 2012;111(7):07A701. doi: 10.1063/1.3670054