ABSTRACT
Room-temperature ferromagnetism was obtained in SrSn0.5Co0.5O3 (SSCO) epitaxial film on SrTiO3 substrate by pulsed laser deposition, and the effects of oxygen pressure (PO2) on the microstructure, optical and magnetic properties were examined. An interesting hybrid epitaxial film embedded with polycrystalline SSCO and SrCoOx nanoparticles was observed. The increase of PO2 relaxed the film/substrate compressive strain, promoted the growth of crystallites and reduced the optical band gap (from 2.43 to 1.68 eV). The SSCO film deposited at 0.1 Pa exhibited good ferromagnetism at 100–300 K, while the ferromagnetism in the film with higher and lower oxygen pressure (1 Pa and 10−4 Pa) was weak.
GRAPHICAL ABSTRACT
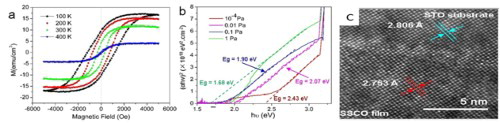
IMPACT STATEMENT
Room-temperature ferromagnetism and tunable optical band gap (2.34–1.68 eV) were demonstrated in Co-doped SrSnO3 thin film grown on SrTiO3 single-crystal substrate by pulsed laser deposition with varying oxygen pressure.
Introduction
Perovskite oxide semiconductors in ABO3 structure are the long-lasting hot spots among functional oxides due to their excellent optical, electronic and magnetic properties [Citation1], and vast applications in superconductors [Citation2], piezoelectric/ferroelectric/thermoelectric devices [Citation3–5], etc. Recently, the rapid rise of the room-temperature transparent ferromagnetism in Co-doped La0.5Sr0.5TiO3−δ [Citation6] and Mn-doped BaSnO3 [Citation7] has spurred interest on visible-light absorption perovskites and diluted magnetic perovskites [Citation8,Citation9]. The combination of tunable transparency and ferromagnetism will provide more optical/electrical dimensions to the spintronic devices, thus more suitable for the function integration. However, it is not easy to precisely modulate the band gap and magnetic properties of perovskite oxides. In ABO3-type perovskites, slight buckling and distortion of chemical bonds can reduce the coordination number of ‘A’ cations and/or ‘B’ cations, producing lower-symmetry distortions, and accordingly resulting in significant variations in the electronic and magnetic properties [Citation10]. Compared with the preparation techniques of bulk materials, the thin film epitaxy is more favorable for the optical and magnetic modulation of perovskites. The substrate can induce film lattice distortions, which greatly affect the optical/electrical/magnetic properties [Citation11,Citation12]. Moreover, the films via vacuum-based deposition can generate oxygen vacancies easily, which play important roles in tuning the electric and magnetic behaviors [Citation13].
Strontium stannate (SrSnO3) is a typical wide band gap (4.27 eV) and diamagnetic perovskite [Citation14]. For the thin films of SrSnO3, previous studies mainly focused on the doping technique used in the transparent conductive films, photocatalysis and afterglow phosphors [Citation15,Citation16]. The efforts on the band gap modulation and ferromagnetism are very limited. Shein et al. [Citation17] predicted that the band gap of SrSnO3 could be as low as 0.7 eV by doping Fe and Co. The optical band gap of 2.36 eV was realized in epitaxial SrSn1−xFexO3 films on MgO [Citation18] and 2.44 eV in epitaxial SrSn1−xCoxO3 films on SrTiO3 (STO) [Citation19]. There is no report on the ferromagnetism of thin films up to now, though weak ferromagnetism was reported in bulk SrSnO3 doped by Fe or Cr [Citation20,Citation21].
Herein, we took SrSnO3 as an example and demonstrated the potential of the thin film epitaxial in tuning the microstructure, optical and magnetic properties of the perovskite materials. By simply varying the oxygen pressure, we obtained distinctive nanoparticles embedded in epitaxial thin films and achieved tunable band gap down to 1.68 eV and the room-temperature ferromagnetism with the saturated magnetization of 12 emu cm−3.
Methods
Ceramic targets of SrSnO3 and SrSn0.5Co0.5O3 (SSCO) were prepared via high-temperature solid-state reaction from SrCO3, SnO2 and Co2O3 powders (Aladdin, 99.8%). Corresponding thin films were grown via the pulsed laser deposition on STO single-crystal substrates, which were ultrasonically rinsed in water, alcohol and acetone successively. The laser source was a 248-nm KrF excimer laser (Lambda physik Compex 201, Germany) with the repetition rate of 5 Hz and the energy density of 200 mJ per pulse. The substrate temperature was fixed at 650°C. The deposition lasted for 1.5 h. The oxygen pressure (PO2) ranged from 10−4 to 1 Pa for SSCO films and was fixed at 0.1 Pa for SrSnO3 film.
The crystallinity was characterized by a two-circle Bruker D8 diffractometer with the Cu-Kα1 radiation. The microstructures were measured by atomic force microscopy (AFM, SΙΙ SPI-3800I, Japan) and FEI Tecnai G2 F20 field emission transmission electron microscope (TEM). The film composition was analyzed on ESCALAB 250 X-ray photoelectron spectrometer (XPS). The optical transmittance spectra were measured with a spectrophotometer (Hitachi, U-4100, Japan). The ferromagnetic properties were measured by Quantum Design MPMS XL5 electrical magnetic measurement system at temperatures of 100, 200, 300 and 400 K.
Results and discussion
Figure (a) presented X-ray diffraction (XRD) patterns of SSCO films with PO2 of 10−4–1 Pa. Only the reflections from the (00l) planes of SSCO and STO (00l) planes were observed, and no other phase or randomly oriented grains were detected. Figure (a) inset showed the shifting trend of the (001) peak towards higher 2θ direction with the PO2 increasing, approaching to the (001) peak of STO, indicating the decrease of the lattice constant and the relaxation of the compressive strain at the film/substrate interface. With the increase of PO2, the concentration of oxygen vacancies in the film decreased, and the coulombic repulsion between cationic Sr and Sn decreased correspondingly [Citation22], which resulted in the reduced lattice constant.
Figure 1. (a) XRD patterns and (b) AFM images of SSCO films on STO (001) substrate with varied PO2. The intensity was in the logarithmic form. The inset of (a) indicated the shift of (001) peak.
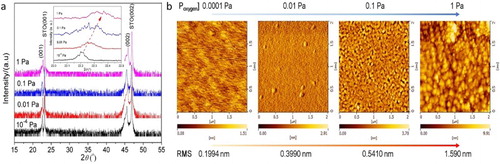
The ω-scan rocking curves and the φ scans were also measured and given in Figure S1. The small full width at half maximum (FWHM) values in the (001) peak of the SSCO films (PO2: 0.1 Pa) (film: 0.04°, substrate: 0.02°) further confirmed the high crystallinity of the film. The appearance of four distinct peaks at an interval of 90° in the φ scans indicated the cubic epitaxial structure of the SSCO films on STO(001) substrates.
The AFM images (Figure (b)) showed that the crystallite size and the overall roughness of the SSCO film increased significantly with PO2. The film deposited at 10−4 Pa was much smoother (root mean square (RMS) roughness: 0.199 nm) than that deposited at 1 Pa (RMS roughness: 1.590 nm), in which large particles with the average size of 100–200 nm were observed. The PO2 of 0.1 Pa seemed to be a turning point where the crystallites started to grow (5–10 nm) and the film still exhibited smooth morphology (RMS roughness: 0.541 nm). This behavior was related to the film growth mode and the mobility of atoms on the SSCO film surface during pulsed laser deposition process [Citation23]. When PO2 was low, the film was grown in a layer to layer mode and the atoms deposited on the surface of film preserved a suitable mobility. In contrast, the higher PO2 hindered the atoms’ mobility effectively, and correspondingly the island growth mode was preferred [Citation24].
The TEM image in Figure (a) showed that the SSCO film was dense and uniform, free from any detectable pores or defects, with the thickness of ∼261 nm. The typical energy-dispersive spectrum (Figure (c)) showed that the film was composed of four elements with the atomic ratio of Sr:Sn:Co = 1:0.426:0.361. The Sn and Co content were slightly lower than the stoichiometric ratio of SSCO, possibly due to their different partial volatilization in the plasma plume during the deposition process. The selective area electron diffraction pattern (Figure (b)) indicated three sets of diffraction patterns, the single-crystal SSCO spot array, the polycrystalline diffraction rings of SSCO and SrCoOx. The ‘x’ value in SrCoOx was deduced between 2.5 and 3.0 based on the detailed examination on the diffraction features of SrCoOx with ‘x’ values of 2.52, 2.61, 2.80 and 3. The high-resolution TEM image in Figure (d) showed that discontinuous polycrystalline SrCoOx nanoparticles existed in the bulk epitaxial film. The high-resolution TEM image of the film/substrate interface (Figure (e)) showed the high epitaxial quality around the film/substrate interface. The detailed microstructure of an embedded SrCoOx particle (Figure (f)) showed an obviously polycrystalline feature in both particle and transitional region between particle and bulk film. Furthermore, we carried out the electron dispersive spectrum line-scanning analysis to the film, and the result is shown in Figure S2. The element distribution of Sr, Co and O both along the perpendicular and parallel scanning lines generally maintained a stable level, ∼60% for Sr, ∼25% for O and ∼ 15% for Co. However, the distribution of element Sn along two lines was discontinuous, and some ‘zero Sn content’ zones were detected, which further proved that the polycrystalline particles embedded in the film were SrCoOx. The formation mechanism of embedded SrCoOx nanoparticles may be explained by the limited solid solubility of Co atom in SrSnO3 lattice. Previous studies on SrSn1−xCoxO3 (x = 0, 0.16, 0.33, 0.5) ceramics proved that the SrCoO2.52 (JCPDS No. 40-1018) phase could be formed in a high Co-substituting SSCO sample [Citation25], which indicated the low solubility of Co atom in the lattice of SrSnO3.
Figure 2. (a) TEM image, (b) selective area electron diffraction pattern and (c) electron dispersive spectrum spectrum of SSCO films on STO (001) substrate (0.1 Pa); (d) high-resolution TEM image of the interface between SSCO films on STO substrate, with the insets illustrating the fast fourier transformation patterns of the red rectangle area in substrate and in film; (e) enlarged view of the film–substrate interface; (f) typical polycrystalline SrCoOx particle embedded in the epitaxial film.
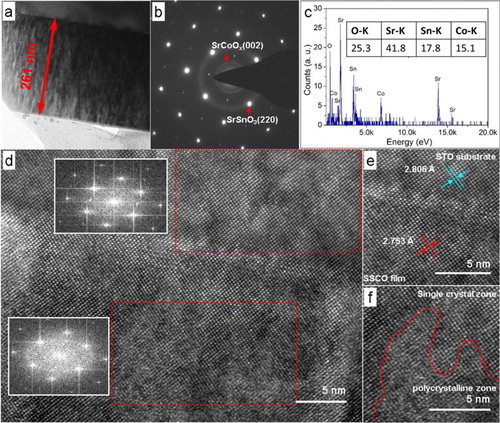
Figure (a) displayed the XPS spectrum of Co 2p electron of the SSCO film grown at 0.1 Pa. Two distinct peaks located at 780.80 and 796.02 eV with a separation of 15.22 eV were recorded, corresponding to the Co 2p3/2 and Co 2p1/2 core peaks. Two satellite shoulders located at 787.2 and 803.2 eV were also the characteristic of Co2+, which originated from the charge-transfer band structure of the 3d transition metal monoxides [Citation26]. There was no signal at 778.3 eV, indicating the nonexistence of free Co clusters in the film. The O1s spectra of SrSnO3 and SSCO films (Figure (b)) exhibited an obviously asymmetric shape, indicating that the oxygen on grain surfaces was in different chemical conditions, and the right peak at 529.85 eV originated from the lattice oxygen and the left one at 531.7 eV originated from the defective oxygen ions on the grain surface [Citation27]. The peak intensity related to the oxygen vacancies in the SSCO film was higher than that in the pure SrSnO3 film, implying that more oxygen vacancies were introduced by Co doping.
Figure 3. XPS spectra of element (a) Co and (b) O in SSCO film and SrSnO3 films (oxygen pressure: 0.1 Pa). The color lines were fitting curves of Co 2p peaks and dash lines were fitting curves of O1s peaks. The charge shifted spectra were corrected using the adventitious C 1s photoelectron signal at 284.6 eV.
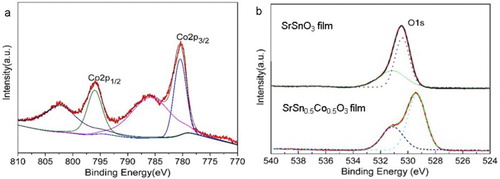
The magnetization of the SSCO film grown at 0.1 Pa measured as a function of magnetic field (M vs. H) at 100–400 K are presented in Figure (a). The hysteresis loops were observed at all four investigated temperatures and testified the room-temperature ferromagnetism of the SSCO film. Especially, the magnetic properties of the film at room temperature were fairly good compared with previous reports [Citation7,Citation20–22], and exhibited the saturated magnetization (Ms) of 12 emu cm−3, the remnant magnetization (Mr) of 4.3 emu cm−3, the coercive field (Hc) of 316 Oe and saturated field (Hs) of 5000 Oe. In view of the relatively high Mr/Ms ratio (300 K: 0.358; 100 K: 0.57) and Hc/Hs ratio (300 K: 0.12; 100 K: 0.40), the film was more close to the hard magnetic material. The oxygen pressure had significant effects on the magnetic properties of the SSCO film; the film deposited at 1 Pa and 10−4 Pa showed very weak magnetic properties (Figure (b)).
Figure 4. Magnetic hysteresis loops of SSCO film deposited at oxygen temperature of (a) 0.1 Pa and (b) 1 Pa on STO (001) substrate. The diamagnetic signals from the STO substrate were subtracted.
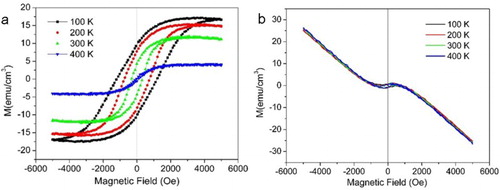
The magnetic moments in the film originated from the cobalt–oxygen vacancy complexes with Co in the +2 valence state [Citation28]. The ferromagnetism of the SSCO could be interpreted using the ‘bound magnetic polaron’ model [Citation29,Citation30], in which the Co ions were spin polarized through the mediation of adjacent oxygen vacancies, resulting in the formation of a bound magnetic polaron. Based on this mode, the ferromagnetism of the SSCO film was affected greatly by two underlying factors: the antiferromagnetic coupling of Co pairs and the concentration of oxygen vacancies (Ov) [Citation31,Citation32]. The antiferromagnetic coupling of Co pairs was suppressed at low temperature and became manifest at high temperature [Citation33], which resulted in the hard to soft magnetic transition with the temperature increase. The concentration of Ov was mainly affected by the PO2. The higher the PO2 was in the deposition atmosphere, the lower the concentration of Ov was in the film. For the film deposited at 1 Pa, weak ferromagnetism was ascribed to the much decreased concentration of Ov and the much lower magnetic moment than that in films with lower PO2. At moderate PO2, the concentration of Ov in the film maintained a good balance with that of Co2+ ions, forming the bound magnetic polaron [Citation34] and improving the magnetic moment [Citation35]. So good ferromagnetism was observed in the film grown at moderate PO2 of 0.1 Pa. At low PO2, though the concentration of Co–Ov complexes was high due to the high concentration of Ov, the ferromagnetism was weakened by the large film–substrate stains in these films. It has been reported that the strain could induce the ferromagnetic to antiferromagnetic phase transition in SrCoOx films [Citation36,Citation37] and that the large interfacial strain at the SrCoOx film/substrates could deteriorate the film ferromagnetism [Citation38]. Thus, very weak ferromagnetism in the film with PO2 of 10−4 Pa was observed in our work.
We further carried out the sheet resistivity at room temperature of SSCO film (Table S2). All films showed a high sheet resistance of >108Ω/square, indicating that the SrSn1−xCoxO3 films were intrinsically insulating.
The optical transmittance spectra of four SSCO films grown on STO substrate are given in Figure (a). In the visible band, the transmittance was affected significantly by the oxygen pressure. For the film with PO2 of 10−4 Pa, the absorption edge started from ∼500 nm and the transmittance of 44% was maintained at ∼400 nm. The absorption edge of other three films with higher PO2 started from 640 nm, and the visible transmittance decreased with the increase of PO2. The film with the PO2 of 1 Pa exhibited the lowest transmittance, lower than 20% at 400 nm. The optical direct allowed band gap of the SSCO films was calculated from the (αhν)2∼photon energy hν curves (Figure (b)) according to the established protocol, where α is the absorption coefficient. With the increase of PO2 from 10−4 to 1 Pa, the band gap of the SSCO film decreased from 2.43 to 2.07 eV, 1.90 eV and finally 1.68 eV, which was much lower than previous reports (2.36 and 2.44 eV) [Citation21,Citation22]. The band gap of ABO3 perovskites was determined by the 3d orbit of B cation(s) (conduction band) and the 2p orbit of oxygen (valence band) [Citation39]. The decrease of the oxygen vacancies concentration with PO2 increasing might slightly raise the energy level of O 2p orbit or pull down the level of Co2+ by partially changing its oxidation status, thus reducing the optical band gap. Similar results were also reported in other perovskite materials [Citation40].
Figure 5. (a) Optical transmittance and (b) (αhν)2∼hν curves of SSCO film on STO (001) substrate deposited at different oxygen temperatures.
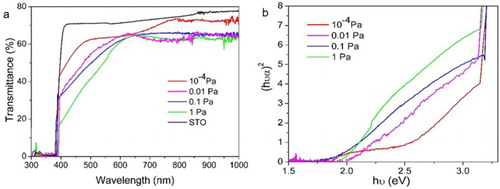
In summary, we prepared room temperature ferromagnetism SSCO film on STO substrate via pulsed laser deposition. By simply changing the oxygen pressure, we realized the optical band modulation from 2.43 to 1.68 eV, room temperature ferromagnetism with the saturated magnetization of 12 emu cm−3. The concentration of oxygen vacancies, the embedded polycrystalline nanoparticles and the interfacial strain at the film/substrate were responsible for the film optical band gap changes and ferromagnetism properties. The work demonstrated a novel film with hybrid microstructure and introduced a general and effective route to tune the optical and magnetic properties of perovskite materials, which would be a benefit to the development of various perovskite-based photovoltaic/photocatalysis/ferromagnetic devices.
SupplementalInformation-01.31.doc
Download MS Word (1.8 MB)Disclosure statement
No potential conflict of interest was reported by the authors.
Additional information
Funding
References
- Rao NR, Meyers RA. Perovskites in reference module in chemistry, molecular sciences and chemical engineering, encyclopedia of physical science and technology. 3rd ed. San Diego, CA: Academic; 2013.
- Maeno Y, Hashimoto H, Yoshida K, et al. Superconductivity in a layered perovskite without copper. Nature. 1994;372:532–534. doi: 10.1038/372532a0
- Lee YB, Han JK, Noothongkaew S, et al. Toward arbitrary-direction energy harvesting through flexible piezoelectric nanogenerators using perovskite PbTiO3 nanotube arrays. Adv Mater. 2017;29:1–8.
- Li M, Pietrowski MJ, De Souza RA, et al. A family of oxide ion conductors based on the ferroelectric perovskite Na0.5Bi0.5TiO3. Nat Mater. 2014;13:31–35. doi: 10.1038/nmat3782
- Sugahara T, Ohtaki M. Structural and semiconductor-to-metal transitions of double-perovskite cobalt oxide Sr2-xLaxCoTiO6-δ with enhanced thermoelectric capacity. Appl Phys Lett. 2011;99:062107. doi: 10.1063/1.3623476
- Wongsaprom K, Swatsitang E, Maensiri S, et al. Room temperature ferromagnetism in co-doped La0.5Sr0.5TiO3-δ nanoparticles. Appl Phys Lett. 2007;90:162506. doi: 10.1063/1.2724772
- Liu Q, He Y, Li H, et al. Room-temperature ferromagnetism in transparent Mn-doped BaSnO3 epitaxial films. Appl Phys Express. 2014;7:1–5.
- Wang X, Huang H, Zhao M, et al. Oxygen-impurity-induced direct–indirect band gap in perovskite SrTaO2N. J Phys Chem C. 2017;121:6864–6867. doi: 10.1021/acs.jpcc.7b01279
- Wang FG, Grinberg I, Rappe AM, et al. Band gap engineering strategy via polarization rotation in perovskite ferroelectrics. Appl Phys Lett. 2014;104:152903. doi: 10.1063/1.4871707
- Kan D, Shimakawa Y. Controlled cation stoichiometry in pulsed laser deposition-grown BaTiO3 epitaxial thin films with laser fluence. Appl Phys Lett. 2011;99:081907. doi: 10.1063/1.3628461
- Jin S, Tiefel TH, McCormack M, et al. Thousandfold change in resistivity in magnetoresistive La-Ca-Mn-O films. Science. 1994;264:413–415. doi: 10.1126/science.264.5157.413
- Horak L, Kriegner D, Liu J, et al. Structure of epitaxial SrIrO3 perovskite studied by interference between X-ray waves diffracted by the substrate and thin film. J Appl Crystallogr. 2017;50:385–398. doi: 10.1107/S1600576717000541
- Pemmaraju CD, Hanafin R, Archer T, et al. Impurity-ion pair induced high-temperature ferromagnetism in Co-doped ZnO. Phys Rev B. 2008;78:59. doi: 10.1103/PhysRevB.78.054428
- Endo T, Masuda T, Takizawa H, et al. Eu2+ luminescence in Srn+ 1SnnO3n+ 1 with layered perovskite structure. J Mater Sci Lett. 1992;11:1330–1332. doi: 10.1007/BF00742193
- Nomura K, Suzuki S, Koike Y, et al. Magnetic property and Mossbauer analysis of SrSn1-xFexO3 prepared by sol-gel method. Hyperfine Interact. 2016;237:857.
- Muralidharan M, Anbarasu V, Perumal AE, et al. Room temperature ferromagnetism in Cr doped SrSnO3 perovskite system. J Mater Sci Mater El. 2017;28:4125–4137. doi: 10.1007/s10854-016-6032-x
- Shein IR, Kozhevnikov VL, Ivanovskii AL. Energy-band structure of the A(Sn1-xMx)O (A = Ca, Sr, Ba; M = Mn, Fe, Co) perovskite-type phases: a search for new magnetic semimetals. Semiconductors. 2006;40:1261–1265. doi: 10.1134/S1063782606110030
- Liu QZ, Li H, Li B, et al. Structure and band gap engineering of Fe-doped SrSnO3 epitaxial films. EPL. 2014;108:37003. doi: 10.1209/0295-5075/108/37003
- Hu XM, Gao XD, Li XM, et al. Microstructure and band gap modulation of SrSn1-xCoxO3 epitaxial thin films via pulsed laser deposition. Acta Phys Chim Sin. 2016;32:828–833.
- Prathiba G, Venkatesh S, Harish Kumar N. Structure, magnetic and semiconducting properties of Fe doped SrSnO3. Solid State Commun. 2010;150:1436–1438. doi: 10.1016/j.ssc.2010.05.021
- Roh KS, Ryu KH, Yo CH. Nonstoichiometry and physical properties of the SrSn1-xFexO3-y system. J Solid State Chem. 1999;142:288–293. doi: 10.1006/jssc.1998.8033
- Perez-Casero R, Perrière J, Gutierrez-Llorente A, et al. Thin films of oxygen-deficient perovskite phases by pulsed-laser ablation of strontium titanate. Phys Rev B. 2007;75:5100. doi: 10.1103/PhysRevB.75.165317
- Hiltunen J, Seneviratne D, Tuller HL, et al. Crystallographic and dielectric properties of highly oriented BaTiO3 films: influence of oxygen pressure utilized during pulsed laser deposition. J. Electroceram. 2009;22:395–404. doi: 10.1007/s10832-008-9443-0
- Chen AP, Khatkhatay F, Zhang W, et al. Strong oxygen pressure dependence of ferroelectricity in BaTiO3/SrRuO3/SrTiO3 epitaxial heterostructures. J Appl Phys. 2013;114:124101. doi: 10.1063/1.4821643
- Yu CJ, Yu AM, Zhang Y, et al. Preparation and microstructure of Co doped SrSnO3 ceramics via solid state reaction. China Ceram. 2017;53:43–48.
- Wang ZH, Geng DY, Guo S, et al. Ferromagnetism and supperparamagnetism of ZnCoO:H nanocrystals. Appl Phys Lett. 2008;92:242505. doi: 10.1063/1.2948863
- Yan S, Ge S, Zuo Y, et al. Effects of carbothermal annealing on structure defects, electrical and magnetic properties in Fe-doped In2O3. Scripta Mater. 2009;61:387–390. doi: 10.1016/j.scriptamat.2009.04.022
- Agham BP, Chandrima M, Chungwei L. Et al. Oxygen vacancy-mediated room-temperature ferromagnetism in insulating cobalt-substituted SrTiO3 epitaxially integrated with silicon. Phys Rev B. 2013;87:144422. doi: 10.1103/PhysRevB.87.144422
- Coey JMD, Venkatesan M, Fitzgerald CB. Donor impurity band exchange in dilute ferromagnetic oxides. Nat Mater. 2005;4:173–179. doi: 10.1038/nmat1310
- Mitra C, Lin C, Posadas AB, et al. Role of oxygen vacancies in room-temperature ferromagnetism in cobalt-substituted SrTiO3. Phys Rev B. 2014;90:278. doi: 10.1103/PhysRevB.90.125130
- Xie CK, Nie YF, Wells BO, et al. Magnetic phase separation in SrCoOx (2.5 ≤ x ≤ 3). Appl Phys Lett. 2011;99:052503. doi: 10.1063/1.3622644
- Lee JH, Rabe KM. Large spin-phonon coupling and magnetically induced phonon anisotropy in SrMO3 perovskites (M = V, Cr, Mn, Fe, Co). Phys Rev B. 2011;84:104440. doi: 10.1103/PhysRevB.84.104440
- Xu W, Yang J, Bai W, et al. Oxygen vacancy induced photoluminescence and ferromagnetism in SrTiO3 thin films by molecular beam epitaxy. J Appl Phys. 2013;114:154106. doi: 10.1063/1.4825257
- Roberts KG, Varela M, Rashkeev S, et al. Defect-mediated ferromagnetism in insulating Co-doped anatase TiO2 thin films. Phys Rev B. 2008;78:969.
- Zhang AM, Zhang WC, Wu XS, et al. Abnormal enhancement of ferromagnetism for LaMnO3+δ thin films with decreasing oxygen pressure. AIP Adv. 2017;7:055837. doi: 10.1063/1.4978657
- Callori SJ, Hu S, Bertinshaw J, et al. Strain-induced magnetic phase transition in SrCoO3−δ thin films. Phys Rev B. 2015;91:140405. doi: 10.1103/PhysRevB.91.140405
- Hao L, Zhang ZF, Xie XN, et al. Preparation of SrCoOx thin films on LaAlO3 substrate and their reversible redox process at moderate temperatures. J Cryst Growth. 2015;427:36–41. doi: 10.1016/j.jcrysgro.2015.07.010
- Jonathan RP, Chandrima M, Hyoungjeen J, et al. Strain control of oxygen vacancies in epitaxial strontium cobaltite films. Adv Funct Mater. 2016;26:1564–1570. doi: 10.1002/adfm.201504868
- Pari G, Jaya SM, Subramoniam G, et al. Density-functional description of the electron structure of LaMO3 (M = Sc, Ti, V, Cr, Mn, Fe, Co, Ni). Phys Rev B. 1995;51:16575–16581. doi: 10.1103/PhysRevB.51.16575
- Chang L, Wang L, You L, et al. Band gap tuning of nickelates for photovoltaic applications. J Phys D. 2016;49:44LT02. doi: 10.1088/0022-3727/49/44/44LT02