ABSTRACT
SnO2@SnS2 multi-core–shell heterostructures were synthesised via chemical conversion of mesoporous raspberry-like SnO2 nanocrystals. In the syntheses, different concentrations of a sulphide precursor (thioacetamide) were applied. The samples were analysed using scanning and high-resolution transmission electron microscopy, X-ray diffraction, Raman spectroscopy, energy-dispersive X-ray spectroscopy, and X-ray photoelectron spectroscopy. The results revealed the presence of surface defects (Sn2+, oxygen vacancies) on the raspberry-like surface, which play a crucial role in the chemical conversion reaction. With an increasing amount of thioacetamide, additional redox reactions occurred in the system (Sn4+/Sn2+, S2−/S0). The highest precursor concentration led to the microstructural disintegration and phase composition change.
GRAPHICAL ABSTRACT
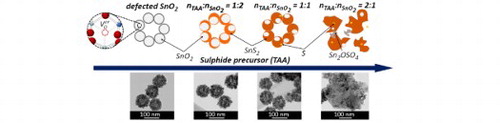
IMPACT STATEMENT
This paper demonstrates the role of defects on the formation of SnO2@SnS2 multi-core–shell heterostructures in mesoporous SnO2 nanomaterials.
KEYWORDS:
Introduction
In recent years, semiconducting metal sulphides have attracted much attention as promising candidates for renewable energy-related applications [Citation1]. Due to the narrow bandgap, they can work as visible-light-driven photocatalysts for solar hydrogen generation, CO2 conversion into fuels, wastewater treatment, and air purification [Citation1–3]. Among these compounds, tin disulphide (SnS2) is a non-toxic, relatively inexpensive, layered n-type semiconductor with a band gap of 2.0–2.35 eV, and a conduction band edge enabling H2 generation [Citation2,Citation4]. However, this material is vulnerable to photocorrosion, which hinders its long-term efficiency [Citation4,Citation5]. To date, various strategies have been investigated to suppress photocorrosion [Citation5]. The formation of heterostructures with a wide-bandgap semiconductor and morphology control represent some of them. The former allows increasing the photostability due to the separation of charge carriers. Depending on the interface structure between coupled materials, core–shell configurations can be distinguished [Citation5,Citation6]. In these systems, the core material is typically a dense single sphere or connection of several small spheres. However, it can also possess 1D, 2D, or 3D morphology. Likewise, there are also different types of shell materials (i.e. continuous and discontinuous, porous and dense, and possessing various shapes) [Citation7]. Herein, we demonstrate the formation of uncommon multi-core–shell heterostructures obtained via chemical conversion of SnO2-based mesoporous hollow-sphere core material. In the literature, numerous synthetic routes of mesoporous SnO2 using various structure-directing agents have been proposed [Citation8–11]. The synthesis of raspberry-like SnO2 hollow spheres for sensing applications was reported by Zhao et al. [Citation11]. This mesoporous morphology of hollow nanospheres may also be advantageous for photocatalytic processes due to enhanced light-harvesting and an increased number of active sites for surface redox reactions [Citation12]. The combination of morphology design and SnO2@SnS2 type-II heterojunction may result in obtaining materials with enhanced photoactivity and photostability [Citation2–5]. To obtain SnO2/SnS2 heterostructures, various approaches have been analysed. Overall, two main strategies can be distinguished. The first one is SnS2 oxidation, which can be conducted using heat treatment [Citation4], ball-milling in the air [Citation13], and hydrothermal route via self-hydrolysis [Citation14], or with the addition of substances such as H2O2 [Citation2], and ascorbic acid in the presence of HCO3− ions [Citation15]. The second strategy is SnO2 conversion, which can be performed via solid-state reaction or hydrothermally using a sulphide precursor such as thioacetamide (TAA), thiourea, or Na2S [Citation3,Citation10,Citation16]. Herein, we applied a hydrothermal SnO2-conversion approach using TAA as an SnS2 precursor. We aimed to synthesise mesoporous SnO2@SnS2 heterostructures composed of multi-core–shell particles. To the best of our knowledge, this is the first time that a formation mechanism of such structures has been proposed. Our motivation for selecting the SnO2-conversion strategy was to utilise the SnO2 raspberry-like hollow spheres as a substrate stabilising SnS2. This well-defined morphology offers numerous active centres for adsorption/redox reactions, enhanced light harvesting, and the coverage of SnO2 with visible-light-driven SnS2. The proposed multi-core–shell SnO2@SnS2, combining the advantages of a mesoporous hollow-sphere morphology and type-II heterojunction, may be promising candidates for solar energy-related applications, such as hydrogen generation in photoelectrochemical cells, carbon dioxide conversion into fuels, and air/water purification. Moreover, they may also be applied as sensing materials in electrochemical and resistive-type sensors.
Materials and methods
SnO2@SnS2 multi-core–shell mesoporous heterostructures were prepared via chemical conversion of SnO2 raspberry-like hollow spheres (RS). In the syntheses, three different concentrations of the sulphide precursor (thioacetamide, TAA) were applied. Depending on the molar ratio of TAA to RS, the samples were labelled RSS-1:2, RSS-1:1, and RSS-2:1. The obtained materials were characterised using scanning and high-resolution transmission electron microscopy (SEM, HRTEM), X-ray diffraction (XRD), Raman spectroscopy, BET/BJH analysis, energy dispersive spectroscopy (EDX), X-ray photoelectron spectroscopy (XPS), and UV-Vis diffuse reflectance spectroscopy (DRS). The details are described in the Supplemental Material.
Results and discussion
The microstructural analysis was performed using SEM and TEM (Figure ). SEM micrographs indicate that the RS sample consists of well-dispersed spherical nanoparticles with a diameter of about 78 nm. TEM analysis, in turn, revealed that RS are hollow-sphere structures. These nanospheres are composed of 12 nm elements that form a 0D-0D raspberry-like shape. In the case of SnO2@SnS2 hybrid materials, the core morphology is preserved in RSS-1:2 and RSS-1:1 samples. The latter is characterised by a slightly larger particle size than RSS-1:2 (87 and 79 nm, respectively). This may indicate that the sulphide layer is thicker for RSS-1:1. In the case of RSS-2:1, SEM images suggest that the raspberry-like core is fully covered with the shell material, forming a flower-like structure of about 167 nm in size. Surprisingly, TEM examination revealed that the core is completely disintegrated. It turned out that the sample is composed of hexagon-like plates with smaller elements randomly distributed on their surface.
Figure 1. (a-d) SEM and (e-h) TEM images of the obtained samples: (a, e) RS, (b, f) RSS-1:2, (c, g) RSS-1:1, and (d, h) RSS-2:1.
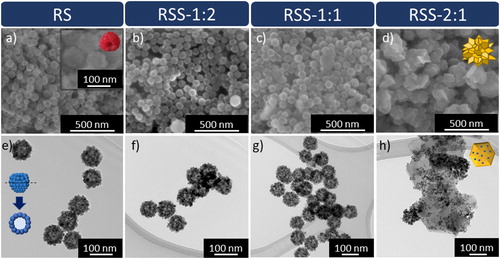
The TEM images also suggest that the raspberry-like hollow spheres (RS, RSS-1:2, RSS-1:1) are mesoporous structures. To confirm this assumption, BET/BJH analysis was performed. The nitrogen adsorption–desorption isotherms (Figure S1), pore size distribution (Figure S2), calculated surface and pore parameters (Table S1), and the interpretation are given in the Supplemental Material.
Figure (a) presents the XRD patterns recorded for the obtained samples. For RS, all diffraction peaks can be assigned to tetragonal cassiterite SnO2 (PDF no. 98-016-9033). In the case of RSS-1:2, additional characteristic X-ray reflections related to (001), (101), and (102) facets of hexagonal SnS2 (PDF no. 98-065-9217) also appeared. Rietveld quantitative analysis revealed that the sample contains 97.4% of SnO2 and 2.6% of SnS2. With an increasing amount of TAA used in the synthesis, the intensity of the (001) diffraction peak from hexagonal SnS2 enhances continuously, and other characteristic peaks from this phase appear. This confirms the increasing SnS2 content from RSS-1:2 to RSS-2:1. The diffraction patterns of RSS-1:1 and RSS-2:1 also revealed the presence of SnS2 with two distinct preferred orientation directions ((001) and (110), respectively). The former contains 8.0 and 3.6% of differently oriented SnS2 phases (11.6% in total), and the latter – 19.0 and 13.9% (32.9% in total), respectively. In the case of RSS-2:1, diffraction peaks assigned to sulphur (PDF no. 00-013-0141) and tin oxide sulphate Sn2OSO4 (PDF no. 98-003-5101) were also detected.
To determine the structural changes of the raspberry-like SnO2 and its heterostructures, Raman spectroscopy was applied (Figure (b,c)). This technique allows the analysis of the materials’ vibrational modes. However, in the case of SnO2, it can be used to designate the presence of lattice defects and disclose the surface-related ones. In nature, bulk SnO2 exhibits three main vibrational modes: Eg (474 cm−1), A1g (627 cm−1), and B2g (768 cm−1) [Citation17]. With an increased surface area, an additional Raman band may appear in the range of 542–575 cm−1 [Citation17,Citation18] assigned to the presence of surface-related defects (oxygen vacancies). The second characteristic band may appear at about 693 cm−1 and is associated with the occurrence of the local lattice disorder [Citation17,Citation18]. All five vibrational modes can be detected for RS (Figure (c)). The mesoporous structure of RS characterises a large surface area. Moreover, the raspberry-like shape comprises 0D elements of about 12 nm in diameter. These two factors may affect the formation of surface and lattice defects, which act as high-energy active centres. During the synthesis of RSS, the ion exchange mechanism starts after the adsorption of S2− ions on these centres. Therefore, the intensity of the defect-related bands decreases (RSS-1:2). For RSS-1:1 and RSS-2:1, the band at 317 cm−1 assigned to SnS2 appeared (Figure (b)). It is worth noting that for RSS-1:1, the intensity of the defect-related band at 565 cm−1 increased (compared to RSS-1:2). This behaviour may be attributed to the growth of SnS2 in two distinct preferred orientation directions or/and weakening the bonds that connect the individual elements forming a raspberry-like structure, as also evidenced by the shift of the aforementioned band toward higher wavenumbers. This bond-weakening results in complete structural disintegration of RSS-2:1, which is also related to the presence of accessible surface area.
More detailed microstructural and structural studies were performed using high-resolution transmission electron microscopy (HRTEM) and a PDF database. Figure (a, b) presents HRTEM images of RS. The observed lattice fringes of about 3.35 Å (marked in white) can be attributed to the (110) crystal facet of tetragonal SnO2, which is consistent with the XRD results. Differently oriented (110) facets come from smaller elements that form hollow-sphere structures. Moreover, two additional lattice spacings of about 3.45 and 2.72 Å (marked in blue) can be observed. These lattice spacings may correspond to the (0–11) and (030) facets of mixed-valence anorthic Sn2O3, or the (−120) and (210) ones of anorthic Sn3O4, respectively. The amount of these intermediate phases in the samples is below the detection limit of XRD. Thus, the HRTEM results confirm the presence of defects (Sn2+) on the RS surface. Figure (c, d) presents HRTEM images of RSS-1:1. For this sample, the d-spacing values that correspond to SnO2 and intermediate tin oxide phases can also be observed. Moreover, HRTEM examination confirms the presence of 5.88 and 3.17 Å lattice fringes (marked in yellow) that can be ascribed to (001) and (100) facets of hexagonal SnS2, respectively. The lattice spacing of about 3.32 Å (marked in orange) may correspond to (222) facet of monoclinic sulphur, which presence in the case of this sample was also not detected using XRD analysis. During the synthesis, the S2− ions may be oxidised to S0 due to the reduction of Sn4+ to Sn2+ ions on the raspberry-like surface.
Figure 3. HRTEM images and enlarged areas with determined d-spacing values of (a, b) RS and (c, d) RSS-1:1.
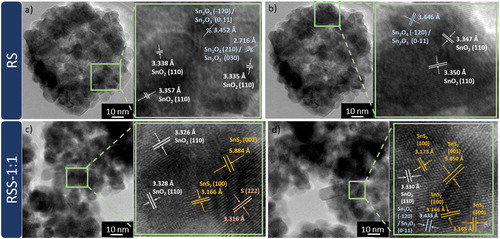
The distribution of chemical elements in the samples was analysed using energy-dispersive X-ray (EDX) mapping (Figure (a,b)). The results reveal that tin, oxygen, and sulphur are homogeneously distributed in the raspberry-like SnO2/SnS2 heterostructures (RSS-1:2, RSS-1:1). The surface chemistry of the selected elements and surface defects were studied by XPS. Figure (c-e) presents the Sn 3d5/2, S 2p, and O 1s high-resolution spectra. The peak of Sn 3d5/2 exhibits two components at 486.5 and 484.8 eV that are assigned to Sn4+ and Sn2+, respectively. For the RS, RSS-1:2, and RSS-1:1 samples, the Sn2+ contribution is insignificant and amounts to about 1.2–1.9%. Starkly different results were obtained for the sample synthesised using the highest molar ratio of TAA to SnO2 (RSS-2:1), for which the Sn2+ contribution is close to 22%. The higher amount of Sn2+ is related to the presence of Sn2OSO4 phase. For RSS-1:2, the S 2p peak is fitted by two components at 162.58 and 161.38 eV, which are ascribed to S2− in SnS2. In the case of RSS-1:1 and RSS-2:1, two more components with higher binding energy corresponding to elemental sulphur (S0) are fitted. For the O 1s spectra, the XPS peak is deconvoluted into two components. The lower-energy component is related to the lattice oxygen in tin dioxide (530.3 eV), while the higher-energy one is associated with surface defects. According to the literature reports [Citation19], this binding energy close to 531.35–531.75 eV can be attributed to oxygen vacancies in the oxide lattice. Based on the analysis of the area ratio of S, Sn, and O species from the fitted components, the fraction of Sn2+, S0, and surface defects is the highest for RSS-2:1. The XPS results confirm the presence of Sn2+, S0 and oxygen vacancies that were detected by Raman spectroscopy and HRTEM (Sn2+, S0).
Figure 4. (a-b) EDX mapping images for raspberry-like SnO2@SnS2 heterostructures, (c-e) XPS spectra of Sn 3d5/2, S 2p, and O 1s.
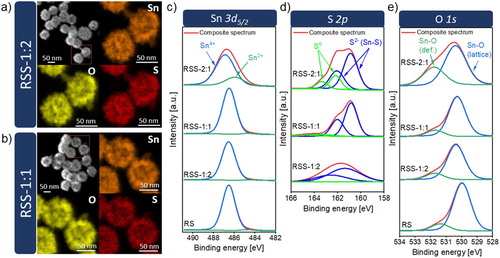
The UV-Vis DRS measurements were performed to study the optical properties of the materials, as they are potential candidates for photocatalysts (Figure S3). The results are described in the Supplemental Material.
Based on the obtained results, the growth mechanism of multi-core–shell SnO2@SnS2 heterostructures can be proposed (Figure ). The surface of mesoporous raspberry-like SnO2 is characterised by the presence of defects, such as Sn2+ cations and oxygen vacancies. These defects play a crucial role as high-energy active sites in the chemical conversion process. In aqueous solutions, TAA undergoes hydrolysis (CH3CSNH2 + H2O ↔ CH3CONH2 + H2S). The released S2− ions adsorb on the defective surface centres of RS. Thereafter, the exchange of ions occurs. RSS-1:2 contains 2.6% of SnS2. As the concentration of TAA increases, the SnS2 content in the samples is higher. In the case of RSS-1:1, HRTEM and XPS measurements also revealed the presence of elemental sulphur. For RSS-2:1, an increased amount of S0 and Sn2+ was detected. This may indicate that with an increasing amount of TAA, Sn4+ cations are reduced to Sn2+, while S2− ions are oxidised to S0. For RSS-2:1, some elemental sulphur was further oxidised to S6+ in Sn2OSO4. Moreover, the growth of two distinctly oriented SnS2 phases and bond-weakening between the individual elements forming mesoporous SnO2 result in the complete disintegration of the raspberry-like shape. In this sample, SnS2 exhibits the morphology of hexagon-like layers surrounded by smaller SnO2 crystals.
Conclusions
The multi-core–shell structure of SnO2@SnS2 is determined by the defective surface of the mesoporous core SnO2 materials, which comprise very small (12 nm) individual elements that form the raspberry-like shape. The RSS-1:2 and RSS-1:1 samples (with the SnS2 content of 2.6% and 11.6%, respectively) exhibit multi-core–shell structure, while the raspberry-like shape of RSS-2:1 (32.9% of SnS2) was completely disintegrated. In addition to the ion exchange, the reduction of Sn4+ cations and the oxidation of S2− anions occur during the chemical conversion of SnO2 to SnS2. The higher the sulphide precursor (TAA) concentration used in the synthesis, the increased amount of Sn2+ and S0 was detected in the samples. The examination of (micro)structural, surface, and optical properties revealed that the proposed multi-core–shell SnO2@SnS2 are promising candidates for visible-light-driven photocatalysts in various devices, adsorbents and sensing materials.
Supplemental Material
Download MS Word (581.3 KB)Disclosure statement
No potential conflict of interest was reported by the author(s).
Additional information
Funding
References
- Chandrasekaran S, Yao L, Deng L, et al. Recent advances in metal sulfides: from controlled fabrication to electrocatalytic, photocatalytic and photoelectrochemical water splitting and beyond. Chem Soc Rev. 2019;48:4178–4280.
- Zhang YC, Du ZN, Li KW, et al. High-performance visible-light-driven SnS2/SnO2 nanocomposite photocatalyst prepared via in situ hydrothermal oxidation of SnS2 nanoparticles. ACS Appl Mater Interfaces. 2011;3:1528–1537.
- Sunaina, Yadav KK, Ankush, et al. Mechanistic insights of enhanced photocatalytic efficiency of SnO2-SnS2 heterostructures derived from partial sulphurization of SnO2. Sep Purif Technol. 2020;242:116835.
- Mu J, Teng F, Miao H, et al. In-situ oxidation fabrication of 0D/2D SnO2/SnS2 novel Step-scheme heterojunctions with enhanced photoelectrochemical activity for water splitting. Appl Surf Sci. 2020;501:143974.
- Chen S, Huang D, Xu P, et al. Semiconductor-based photocatalysts for photocatalytic and photoelectrochemical water splitting: will we stop with photocorrosion? J Mater Chem A. 2020;8:2286–2322.
- Bai S, Xiong Y. Some recent developments in surface and interface design for photocatalytic and electrocatalytic hybrid structures. Chem Commun. 2015;51:10261–10271.
- Srdic V, Mojic B, Nikolic M, et al. Recent progress on synthesis of ceramics core/shell nanostructures. Process Appl Ceram. 2013;7:45–62.
- Chandra D, Mukherjee N, Mondal A, et al. Design and synthesis of nanostructured porous SnO2 with high surface areas and their optical and dielectric properties. J Phys Chem C. 2008;112:8668–8674.
- Bhanja P, Mohanty B, Patra AK, et al. Iro2 and Pt doped mesoporous SnO2 nanospheres as efficient electrocatalysts for the facile OER and HER. ChemCatChem. 2019;11:583–592.
- Wang S, Li G, Leng Z, et al. Systematic optimization of promoters in trace SnS2 coating SnO2 nano-heterostructure for high performance Cr(VI) photoreduction. Appl Surf Sci. 2019;471:813–821.
- Zhao R, Wang Z, Yang Y, et al. Raspberry-like SnO2 hollow nanostructure as a high response sensing material of gas sensor toward n-butanol gas. J Phys Chem Solids. 2018;120:173–182.
- Xiao M, Wang Z, Lyu M, et al. Hollow nanostructures for photocatalysis: advantages and challenges. Adv Mater. 2019;31:1–23.
- Hu K, Ming C, Liu Y, et al. Introducing sulfur vacancies and in-plane SnS2/SnO2 heterojunction in SnS2 nanosheets to promote photocatalytic activity. Chinese Chem Lett. 2020;31:2809–2813.
- Mao L, Li J, Xie Y, et al. Controllable growth of SnS2/SnO2 heterostructured nanoplates via a hydrothermal-assisted self-hydrolysis process and their visible-light-driven photocatalytic reduction of Cr(vi). RSC Adv. 2014;4:29698–29701.
- Ma L, Xu L, Xu X, et al. Fabrication of SnO2/SnS2 hybrids by anchoring ultrafine SnO2 nanocrystals on SnS2 nanosheets and their photocatalytic properties. Ceram Int. 2016;42:5068–5074.
- Li YY, Wang JG, Sun HH, et al. Heterostructured SnS2/SnO2 nanotubes with enhanced charge separation and excellent photocatalytic hydrogen production. Int J Hydrogen Energy. 2018;43:14121–14129.
- Kar A, Kundu S, Patra A. Surface defect-related luminescence properties of SnO2 nanorods and nanoparticles. J Phys Chem C. 2011;115:118–124.
- Macchi C, Ponce MA, Desimone PM, et al. Vacancy-like defects in nanocrystalline SnO2: influence of the annealing treatment under different atmospheres. Philos Mag. 2018;98:673–692.
- Xu K, Li N, Zeng D, et al. Interface bonds determined gas-sensing of SnO2-SnS2 hybrids to ammonia at room temperature. ACS Appl Mater Interfaces. 2015;7:11359–11368.