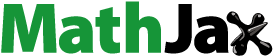
Abstract
Beyond classic martensitic transformation-induced plasticity (TRIP), consecutive hierarchical twinning in the primary martensite is observed providing additional tensile strain about two-to-three times higher than the original transformation strain. The hierarchical twinning, operating in two different modes, contributes to at least one quarter of the plasticity in TRIP/TWIP Ti-12Mo alloy at early deformation stage. During tensile unloading, a peculiar multi-step detwinning process reverses the mechanically twinned martensite to its original orientation, resulting in rubber-like behavior. The complex reversible twinning process is investigated by combining in-situ EBSD/TEM experiments and theoretical rationalization with respect to Schmid factors and twin variant selection.
GRAPHICAL ABSTRACT
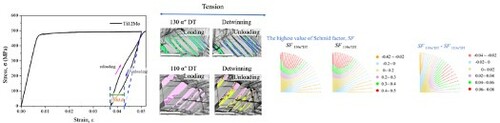
IMPACT STATEMENT
The complex hierarchical twinning-detwinning mechanism in α″−martensite and the associated rubber-like behavior have been clarified in TRIP/TWIP Ti-12Mo unprecedentedly via comprehensive in-situ investigations.
Stress-Induced Martensitic transformation (SIM) (ortho-rhombic martensite α″) and mechanical twinning play an important role in the improvement of strain-hardening rate and the subsequent large uniform elongation during tensile plastic flow in TRIP (Transformation Induced Plasticity)/TWIP (Twinning Induced Plasticity) Titanium alloys [Citation1–3]. The {130}<10>α″ martensitic mechanical twinning (here after called 130α″ DT) system formed in martensite at plastic stage during tensile stress has been widely observed in metastable β titanium alloys [Citation2–5]. Due to the crystallographic equivalences of the twinning elements between 130α″ DT in α″ and {332}<11
>β in the parent β phase, the reversed transformation from 130α″ DT to β has been proposed to possibly be the origin of {332}<11
>β twinning in β metastable alloys [Citation3–6].
Besides, in-situ observations in TRIP/TWIP Ti alloys have evidenced a detwinning process from 130α″ DT to its parent SIM α″ during tensile unloading [Citation1]. Regarding the twinning activation, Yang et al. reported that the selection variant of 130α″ DT activated in primary SIM α″ obey the Schmid law in Ti2448 alloy [Citation7], and similar phenomena were observed by Bertrand et al. in Ti-25Ta-20Nb alloy [Citation4]. Additionally to the 130α″ DT, the {110}<10>α″ mechanical twinning (here after called 110α″ DT), with twinning elements equivalent to {112}<11
>β mode in the parent β phase, has also been reported to be operational in plastic deformation stage near the surface of TRIP/TWIP Ti alloys [Citation7,Citation8]. Yang et al. [Citation9] suggested that the reversion of 110α″ DT may be one of the origin of {112}<11
>β twinning in β metastable alloys.
However, the twinning mode selection, the twinning process and the detwinning mechanisms are still unclear due to the fact that the SIMα″ twinning products can be mechanically unstable. The twin products have been observed to transform back to the parent SIMα″ or β phase during stress release [Citation1,Citation3–6]. The instability of α″ twinning products, therefore, results in difficulties and uncertainties of the ex-situ studies (EBSD and TEM [Citation10]). In order to clarify unambiguously the operative mechanisms, studies have to investigate the deformation mechanisms of the martensitic mechanical twinning at the micro-length-scale of these materials and under in-situ conditions. Hence, the aim of present study is to clarify the evolution of the two modes of martensitic mechanical twinning in Ti-12Mo alloy with in-situ SEM and in-situ TEM observations. The selection criteria between the two mechanical twinning modes are quantitatively characterized by crystallographical transformation strain analysis. Then the study is extended to clarify the twinning/detwinning process via in-situ electron microcopies under tensile deformation. Regarding the macroscopic tensile behavior, the rubber-like behavior observed in the cyclic unloading test can be related to the detwinning of SIMα″.
The fabrication process of Ti-12Mo tensile samples and the In-situ tensile Electron backscatter diffraction (EBSD) method was reported in our recent paper [Citation11]. JEOL 2100plus transmission electron microscope (TEM) operating at 200 kV was used for in-situ traction test on a Gatan heating-straining holder. The specimens (11 × 2.4 × 0.2 mm3 plate with φ2 mm hole near each extremity) for in-situ TEM observations were machined from alloy sheet at recrystallized state without deformation. The center of the plates was thinned by twin-jet electropolishing using a solution of 4% perchloric acid in methanol at 250 K. The Schmid factor (SF) of twinning is calculated from EBSD measurements by:
(1)
(1)
where λ and φ is the angle between the tensile direction and the twinning plane normal, and the twinning direction, respectively.
The cyclic loading-unloading tensile tests at room temperature are carried out until fracture with the increments of 5% strain, and the first cycle is presented in Figure . A rubber-like behavior (RLB) is observed in the unloading curve with a 0.6% recover strain (ϵRLB).
Figure 1. The engineering stress-strain curve of first cyclic loading-unloading test is presented, εRLB: rubber-like behavior recover strain at room temperature after unloading.
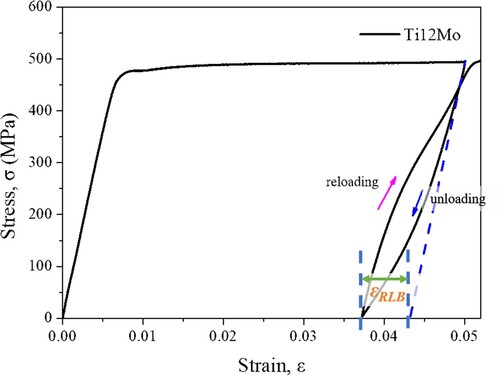
More than 1000 grains in solution treated Ti-12Mo alloy (same initial microstructure as in our previous study [Citation11]) are analyzed by in-situ EBSD mapping under tension. In order to clarify the activation and evolution of the SIMα″ and its twinning, the beta grains that deform primarily by SIMα″ (>300 grains) are further analyzed. According to our statistics, 130α″ DT is more likely to be activated than 110α″ DT even for the orientations where SF130α″ DT < SF110α″ DT or SF130α″ DT = SF110α″ DT. The orientations in this paper are for the tensile axis to the orthorhombic system (α″) of the primary SIMα″. Five cases with different orientations and their associated Schmid factors all possible twinning variants are selected and listed in to analyze the martensitic twinning selection mechanism. The activated variant among the two systems of 130α″ DT and 110α″ DT is marked by blue color. Two representative cases of martensitic twinning, orientation I (130α″ DT dominant) and orientation II (110α″ DT dominant), are shown in details in the following, to further investigate the microstructural characteristics of 130α″ DT and 110α″ DT formation, respectively.
Table 1. Schmid factor for each martensitic mechanical twinning according to the primary SIMα″.
Figure shows in-situ EBSD mapping of orientation I, which is believed to be representative of orientations I, III, IV and V where SIM displays 130α″ DT, according to . Plate-like deformation bands with two different color contrasts (blue and green) appear in the β matrix at ε = 0.05 (Figure (a)). These deformation bands are identified to be SIMα″. According to the crystallographic analysis (common poles marked by red cycles in the pole figures in Figure (c)), the blue SIMα″ is the primary phase directly transformed from β matrix following the classic orientation relationship: {001}β//{100}α″, {110}β//{010}α″, and {10}β//{001}α″ [Citation13]. The green SIMα″, although being predominant within the total SIMα″ analyzed surface, does not orientate in classic relationship to the β matrix. Analysis of the orientation relationships (Figure (c)) shows that both the two variants have a common {130}α″ pole, which suggested that the green variant yields 130α″ DT relationship with primary blue variant. Therefore, the results suggest that a mechanical twinning transformation could have probably occurred before ε = 0.05 in the primary SIMα″. The sample is unloaded from ε = 0.05 by fully releasing the tensile stress to investigate the detwinning process of the 130α″ DT during unloading. The EBSD scan at the same zone is shown in Figure (b) at the unloaded state. It can be noticed that some of the 130α″ DT (the green variant) detwinning to primary blue variant. The surface ratio of the primary martensite increased from 0.09 to 0.55 suggesting a partial detwinning process due to the release of tensile stress.
Figure 2. IPF (Inverse pole figure) of SIMα″ phase + IQ (image quality) maps of a grain with Orientation I taken from the same region at (a) strain ε = 0.05 (loading) and (b) 0.05 (unloading); (c) pole figures of β matrix and SIMα″.
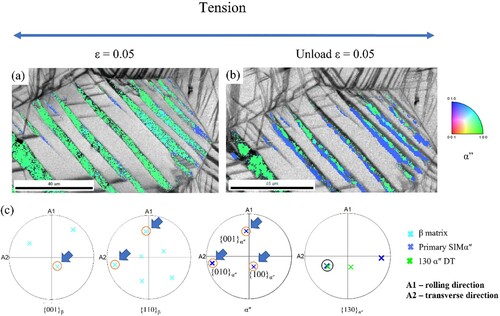
The other case is shown in Figure . At ε = 0.05, SIMα″ bands can be observed in the Figure (a), in which some of the bands are composed by two variants different in color (the pink ones are in majority, and the purple ones are indicated by arrows in Figure (a)). By performing similar crystallographic analysis (Figure (c)) as shown in the previous case, it is found that none of the two α″ variants at this loaded state (pink and purple in Figure (a)) orientates in classic relationship with respect to the β matrix. The pink orientation can be explained by the 110α″ DT twinning of a primary SIMα″ precursor However, no residual precursor SIMα″ is observed in these SIMα″ bands at loaded state. Regarding the purple variant, it matches the orientation relationship of 130α″ DT product transformed from pink variant (note the common {130}α″ plane marked by blue cycle in Figure (c)). By unloading the sample, the EBSD scan at the same zone (Figure (b)) identifies the missing primary SIMα″ precursor (yellow variant), in which process a classic relationship with the β matrix [Citation13] (marked by black cycles and blue arrows in Figure (c)). This classic orientation relationship suggests that the yellow variant SIMα″ could arise through a direct transformation from the β matrix. The crystallographic relationship between the pink variant and SIMα″ note the common {110}α″ plane marked by red cycle in Figure (c), confirms a 110α″ DT relationship between the two variants. The finding corroborates the assumption that the pink variant may probably be a secondary 110α″ DT from the primary SIMα″ (yellow variant), revealing its orientation relationship with the initial precursor after a detwinning process occurring upon unloading. In fact, the detwinning effect is also observed in the 130α″ DT couple between the pink and purple variants. As direct experimental evidence, it can be noticed that the purple variants (marked by blue arrows in Figure (a)) disappear completely in the Figure (b) after unloading. Regarding the entire transformation pathway in a loading/unloading cycle, these results suggest that primary SIMα″ occurred during loading and could be fully transformed to secondary 110α″ DT, then this secondary 110α″ DT could partly transform to 3rd 130α″ DT. Thus, a transformation cascade of β→α″→110α″ DT→130α″ DT and its reversion are captured by in-situ EBSD mapping. During unloading, the detwinning process could occur in reversion of the twinning sequence, which is 3rd 130α″ DT→secondary 110α″ DT→primary SIMα″.
Figure 3. IPF maps of SIMα″ phase + IQ maps of a grain with Orientation II taken from the same region at (a) strain ε = 0.05 (loading) and (b) 0.05 (unloading). (c) pole figures of β matrix and SIMα″.
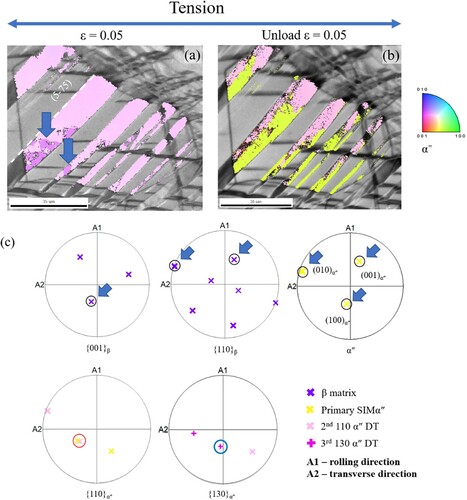
It worth noting that, the non-classic α″/β orientation relationships can be induced via different pathways [Citation14]. For case I, the observed pathway is β→classic primary SIMα″ (detected by EBSD) → secondary 130α″ DT and the alternative pathway is β→{332}<11>β twinning → secondary classic SIMα″ in {332}<11
>β twinning [Citation2]. Similarly in case II, two different pathways can achieve the specific non-classic orientation relationship via (a) secondary SIMα″ transformation in primary {112}<11
>β twinning [Citation9] or via (b) secondary 110α″ DT in primary α″ (proposed in this study). In order to clarify the new pathway b, i.e. the primary β-α″ transformation process and the subsequent 110α″ DT process, in-situ TEM observation under traction is performed to reveal the real-time transformations.
Figure (a and b) shows the bright-filed images under loading. A set of primary SIMα″ bands about 100 nm in width, identified by diffraction patterns and dark-field imaging, are observed during their initial nucleation and growth processes from a pre-existing boundary under tensile loading. Since the very early stage of the primary α″ formation as shown in Figure (a), the 110α″ DT is found to be activated immediately after the nucleation of primary α″ band. The habit plane (75) of primary SIM α″ is marked in Figure by orange dash line. The twinning plane is 110α″ marked by white dash lines in Figure . The in-situ TEM observations confirm the transformation pathway proposed based on the EBSD studies that the primary SIMα″ is activated firstly from β matrix then twins almost immediately to form a deformation band composed by primary and secondary α″. The fraction of 110α″ DT increases along with the growth of primary α″. It can be seen from Figure (b) that one extremity of the SIMα″ band (white arrows in Figure (b)) has already fully twinned, presumably because the twinning is favorable to provide efficiently extra shear strain, to accommodate the local deformation.
Figure 4. In-situ tensile TEM investigations of SIMα″ at Ti12Mo: (a, b) bright field image: at (a) ε = 0.03, (b) ε = 0.05; (c and d) SAED pattern taken from region indicated by circle in (b), and corresponding dark-field images of (e) primary SIMα″ and (f) 110α″ DT, respectively.
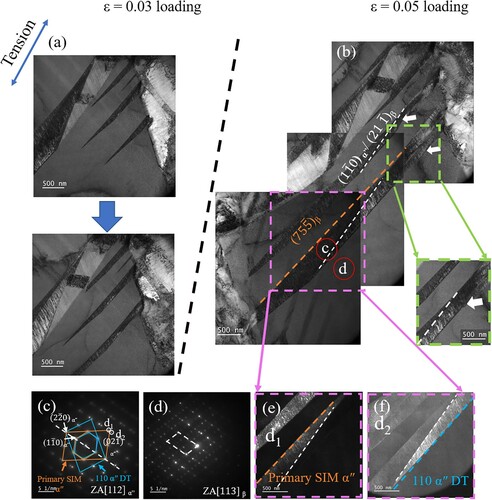
In order to rationalize the DT mode selection in α″ in terms of orientation dependence criteria, calculations of the Schmid factor for random grain orientations (Equation (1)) are performed for an orthorhombic α″ structure (Lattice parameters were measured under tensile loading at ε = 0.04 [Citation2]). The 130α″ DT and 110α″ DT plane and the associated invariant strain direction [Citation12] are used for Schmid factor calculation. For each random grain orientation, the Schmid factor values for every 130α″ DT variants and every 110α″ DT variants are calculated and ranked, respectively. The highest values of Schmid factor for each grain orientation among all variants of the two twinning modes, 130α″ DT and 110α″ DT, are drawn in Figure (a and b) respectively. It can be seen from these figures that the distributions of the highest values of Schmid factor are similar. To further see the difference between the Schmid factor of 130α″ DT and 110α″ DT, the differences of Schmid factor (SF130α″ DT – SF110α″ DT) for random grain orientations are shown in Figure (c).
Figure 5. Inverse pole figure showing the distribution of the highest Schmid factor, SF, of (a) 130α″ DT, (b) 110α″ DT and (c) the values of SF130α″DT minus SF110α″DT.
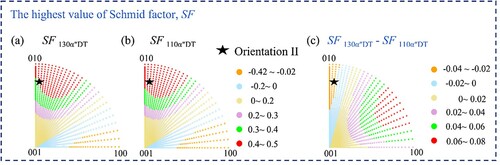
According to the experimental statistics (5 orientation details are listed in ) of the secondary DT selection in α″ at random grains, the 130α″ DT is more likely (98.33% in more than 300 cases) to be occurred compared to 110α″ DT (1.67%). The twin variant selection could be dominated by the Schmid factor of the variant and the critical resolved shear stress (CRSS) of the twinning modes. The selection of the twin variant in each twinning system (130α″ DT or 110α″ DT) obeys the Schmid law as shown in , which consist with the results reported by Yang et al. in Ti2448 [Citation7]. Regarding the twin variant selection between both twinning systems, the activation of 110α″ DT requires a superior Schmid factor as the necessary condition. The associated Schmid factor of 110α″ DT can be superior to that of 130α″ DT in a narrow orientation range near to [010]α″ (see orange and light blue in Figure (c)). The orientation II investigated experimentally is in this range, marked by star in Figure . However, it should be noticed that 130α″ DT is always observed as the operating twinning mode instead of 110α″ DT in this orientation range, where SF130α″ DT is similar or slightly inferior to SF110α″ DT (e.x. Orientation I, III, IV, and V). It suggests that the Schmid factor is not the only factor determining the selection between two twinning systems. Tobe et al. [Citation15] reported that {130}t<10>t is expected to occur more easily compared to {110}t<
10>t due to the smaller magnitude of shuffle in tetragonal structure. A similar result was also reported by Chen et al. in the orthorhombic martensite α″ phase [Citation12]. The smaller magnitude shuffle of 130α″ DT in orthorhombic martensite α″ phase leads to a lower CRSS of 130α″ DT compared to 110α″ DT, which could explain the prevalence of 130α″ DT twinning over than 110α″ DT in metastable titanium alloy.
It is worth noting that the calculation presents nearly one third of the standard triangle is of negative range (−0.42, 0) on the SF in both DT modes (Figure (a,b)). The fact suggests that both mechanical twinning modes are unfavorable for activation under tensile deformation. In this range, the reorientation of SIMα″, i.e. the transformation among correspondence α″ variants, could be preferentially operational to provide positive transformation strain, as reported in [Citation4].
The mechanical twinning products in primary α″ exhibit reverse transformation during tensile unloading through a detwinning process. Similar process has been reported in Ti-Mo and Ti-Mo-Zr systems [Citation1,Citation11]. The spontaneous detwinning contributes to the rubber-like recovery at room temperature during unloading (Figure ). Moreover, the detwinning process is found to be the major contribution to the rubber-like recovery since the SIM α″ volume fraction is almost unchanged before and after unloading (Figures and ). Such detwinning induced rubber-like behavior has not been reported.
In summary, the pathways of hierarchical twinning operated in two different modes (130α″ DT and 110α″ DT) during loading in primary martensite and their detwinning process during unloading are investigated combining by in-situ investigations. A theoretical rationalization with respect to Schmid factors and twin variant selection is then proposed. It is shown that the detwinning process reverses the mechanically twinned martensite to its original orientation, resulting in rubber-like recovery.
Supplemental Material
Download GIF Image (404.2 KB)Disclosure statement
No potential conflict of interest was reported by the author(s).
Additional information
Funding
References
- Qian B, Zhang J, Fu Y, et al. In-situ microstructural investigations of the TRIP-to-TWIP evolution in Ti-Mo-Zr alloys as a function of Zr concentration. J Mater Sci Technol. 2020;65:228–237.
- Sun F, Zhang J, Marteleur M, et al. Investigation of early stage deformation mechanisms in a metastable β titanium alloy showing combined twinning-induced plasticity and transformation-induced plasticity effects. Acta Mater. 2013;61:6406–6417.
- Cho K, Morioka R, Harjo S, et al. Study on formation mechanism of {332}<113> deformation twinning in metastable β-type Ti alloy focusing on stress-induced α” martensite phase. Scr Mater. 2020;177:106–111.
- Bertrand E, Castany P, Yang Y, et al. Deformation twinning in the full-α″ martensitic Ti–25Ta–20Nb shape memory alloy. Acta Mater. 2016;105:94–103.
- Castany P, Yang Y, Bertrand E, et al. Reversion of a parent {130}{310} α′′ martensitic twinning system at the origin of {332}{113} β twins observed in metastable β titanium alloys. Phys Rev Lett 2016;117(24):245501.
- Lai M, Tasan C, Raabe D. On the mechanism of {332} twinning in metastable β titanium alloys. Acta Mater. 2016;111:173–186.
- Yang Y, Castany P, Hao Y, et al. Plastic deformation via hierarchical nano-sized martensitic twinning in the metastable β Ti-24Nb-4Zr-8Sn alloy. Acta Mater. 194 2020;194:27–39.
- Ping D, Yamabe-Mitarai Y, Cui C, et al. Stress-induced α″ martensitic (110) twinning in β-Ti alloys. Appl Phys Lett 2008;93(15):151911.
- Yang Y, Castany P, Bertrand E, et al. Stress release-induced interfacial twin boundary ω phase formation in a β type Ti-based single crystal displaying stress-induced α” martensitic transformation. Acta Mater. 2018;149:97–107.
- Hao Y, Li S, Sun S, et al. Super-elastic titanium alloy with unstable plastic deformation. Appl Phys Lett. 2005;87(9):091906.
- Qian B, Lilensten L, Zhang J, et al. On the transformation pathway in TRIP/TWIP Ti–12Mo alloy. Mater Sci Eng. 2021;A2021(822):141672.
- Chen N, Kou H, Wu Z, et al. Stress-induced α″ martensitic phase transformation and martensitic twinning in a metastable β titanium alloy. J. Alloys Compd. 2021;859:157809.
- Kim H, Ikehara Y, Kim J, et al. Martensitic transformation, shape memory effect and superelasticity of Ti–Nb binary alloys. Acta Mater. 2006;54(9):2419–2429.
- Gao Y, Zheng Y, Fraser H, et al. Intrinsic coupling between twinning plasticity and transformation plasticity in metastable β Ti-alloys: a symmetry and pathway analysis. Acta Mater. 2020;196:488–504.
- Tobe H, Kim H, Inmura T, et al. Origin of {3 3 2} twinning in metastable β-Ti alloys. Acta Mater. 2014;64:345–355.