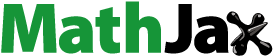
Abstract
CH3NH3SnI3 perovskites, which can be created using printing technology, are environmentally friendly thermoelectric materials, but their applications are limited by unsatisfactory thermoelectric efficiency and structural stability. In this work, CH3NH3SnI3 perovskites are processed by applying high pressure with shear strain for the first time, resulting in better structural stability, enhanced electrical conductivity and the Seebeck coefficient with CH3NH3SnI3 tube structures after processing. First-principles calculations verified the reasonable changes in lattice constants, electronic band structures, electrical conductivity and the Seebeck coefficient. The present study demonstrates a potential strategy to improve the structural and thermoelectric properties of CH3NH3SnI3 and uncovers the possible mechanism.
GRAPHICAL ABSTRACT
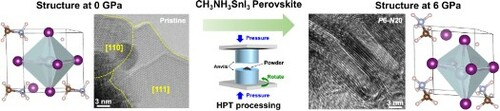
IMPACT STATEMENT
Better structural stability and slightly improved thermoelectric properties are achieved in the CH3NH3SnI3 samples processed by high pressure with shear strain. DFT calculations disclose the possible mechanism.
1. Introduction
Thermoelectric materials can convert waste heat into electricity, and thermoelectric devices have been applied in many fields because they are reliable, integrable, stable and compact [Citation1–4]. CH3NH3SnI3 (hereafter MASnI3), a lead-free halide perovskite, has been recognized as a potential thermoelectric material for printed electronics, due to its large absorption coefficient, high charge carrier mobility, long diffusion length of charge carriers and ultralow thermal conductivity [Citation5–9]. However, the thermoelectric efficiency and structural stability of MASnI3 are unsatisfactory and need to be improved. The performance evaluation of thermoelectric materials involves the use of a dimensionless figure of merit (ZT) [Citation1], ZT = S2σT/κ, where S is the Seebeck coefficient, T is the absolute temperature, σ is the electrical conductivity and κ is the thermal conductivity. Maximizing ZT is the primary goal of thermoelectric materials research, which requires enhancing σ and S while minimizing κ.
Both theoretical [Citation10,Citation11] and experimental [Citation12,Citation13] studies show that the ZT value of MASnI3 could rise with suitable doping, such as chemical doping, photoexcitation and hole doping. But the structural stability of MASnI3 is still very low. To modify the structural and electronic properties, it was found that the key roles are chemical pressure induced by lattice mismatch and synthetic temperature [Citation14]. The external pressure is an alternative to chemical pressure because it can directly adjust the interatomic distances to impact the crystal structure and electronic properties. High-pressure techniques have already been performed on different kinds of materials to study the relationships between structures and properties [Citation15–17]. Lü et al. [Citation18] processed MASnI3 by applying a high hydrostatic pressure up to 31 GPa and proved that the structural stability and electrical conductivity of MASnI3 improved after compression. Furthermore, a significantly enhanced emission of photoluminescence [Citation19] and a meaningful increase in electron transport and photocurrent [Citation20,Citation21] were also observed in different kinds of halide perovskites under high pressure.
The high-pressure torsion (HPT) method [Citation22,Citation23], as schematically shown in Figure (a), is an effective technique for simultaneously introducing high pressure and large shear strain to a sample by rotating the two anvils with respect to each other [Citation24]. The HPT method has already been used on various oxides [Citation25–33], semiconductors [Citation34], perovskites [Citation35], metallic and intermetallic compounds [Citation36–40] and oxynitride [Citation41]. It has been proved that HPT processing can improve many functional properties such as photocatalytic [Citation25–31,Citation35,Citation41], electronic [Citation32], superconductive [Citation42,Citation43] and thermoelectric [Citation39,Citation40] properties. Here, to enhance the structure stability, electrical conductivity and Seebeck coefficient, MASnI3 powder is processed by the HPT method for the first time. First-principles calculations are also used to investigate the lattice constants, electronic band structures and thermoelectric properties under different hydrostatic pressures.
2. Materials and methods
Experiment. The HPT process was conducted on MASnI3 powders that were prepared by solid state reactions. The phase transformation, lattice constants and stability test of the MASnI3 samples were studied by X-ray diffraction (XRD) using Cu Kα. Raman spectra were collected by using a Raman spectrometer with a 532 nm excitation laser. The microstructure of the samples was observed by high-resolution transmission electron microscopy (HRTEM). The bandgap of the samples was studied by ultraviolet–visible light diffuse reflectance spectroscopy (UV-Vis). The electrical conductivity was measured by a conventional four-probe method and the Seebeck coefficient was measured by a dc method. The detailed sample preparation and HPT conditions are in the Supplemental Materials.
DFT calculations. A pseudo-cubic MASnI3 structure was used in the DFT calculations at 0, 2 and 6 GPa hydrostatic pressure performed in QuantumATK [Citation44] using norm-conserving pseudopotentials and the plane-wave basis projector augmented wave (PAW) potentials. The structure optimization was performed, and the band structure and density of states (DOS) were carried out, by using DFT combined with the Perdew–Burke–Ernzerh (PBE) variant of generalized-gradient approximation (GGA) for exchange–correlation energy. The Heyd–Scuseria–Ernzerhof (HSE) exchange–correlation functional was combined with the DFT calculations to investigate the bandgap for comparing with the experimental data. The electrical conductivity and the Seebeck coefficient were obtained by the calculations of the electron transmission spectrum. The detailed calculation methods are explained in the Supplemental Materials.
3. Results and discussion
Structural characterization. In the hydrostatic pressure treatment up to 31 GPa [Citation18], in situ XRD characterization showed that phase transformation occurred at 0.7 GPa in the compression process and disappeared in the decompression process, which indicated the existence of a high-pressure phase. However, no new peak was observed in the XRD profiles, as shown in Figure (b). This indicates that no phase transformation occurred due to the HPT processing. It is possible that the high-pressure phase was formed during the processing but disappeared after unloading the pressure. If the in-situ structural measurement of HPT processing on MASnI3 samples can be achieved in the future, the high-pressure phase and other exciting structural changes could be well studied. A small peak shifting to a high Bragg angle (Figure S1(a)) was found in the HPT-processed samples, which suggests that the lattice constant was reduced by the HPT processing. It should be noticed that peak boarding was not appreciable even after the HPT processing, and this indicates that the amount of lattice defects introduced in MASnI3 by the HPT processing was less than that in tantalate perovskites [Citation35].
The bulk modulus of MASnI3 is reported as 12.3 GPa [Citation18], which is much smaller than that of tantalate perovskites like LiTaO3 (the bulk modulus is 123 GPa, Materials Project id mp-3666 [Citation45]). This means that MASnI3 behaves in a highly compressive manner. Furthermore, the change in Raman spectra (Figure S1(b)) in the librational motions of MA cations is not obvious, which indicates a similar local bonding of MA [Citation46]. The torsional modes of the MA cations become weak after HPT processing and also reveal some changes in the orientation of organic cations [Citation46]. The SnI6 octahedra may tilt and the Sn cations may have a larger off-centering position in the octahedra due to the lone pair effect after HPT processing [Citation17]. The variation in these local structures may impact the electrical and optical properties of HPT-processed MASnI3. Thus, the influence of HPT processing on the whole crystal structure of MASnI3 is minor compared with the HPT-processed tantalate perovskite [Citation35]. As shown in Figure (c), the lattice constant is obtained from the XRD data by the least-square fitting method using a cubic MASnI3 structure with a reduction of up to 0.011 Å.
The stability test was also performed by XRD characterization on all the samples under different aging times in air at room temperature. In Figure (a), there is no obvious change on all the samples after aging for 5 min. But clear peaks at 25.72° and 29.74° appear in the XRD profile of pristine MASnI3 powders after 2 h of aging (Figure (b)), which means the occurrence of oxidation and/or decomposition. The aging and XRD measuring conditions were kept the same in all the samples to compare the peak intensity. The intensities of these two peaks are very low in all the HPT-processed samples; especially, these two peaks are not observed in the P6-N20 sample (Figure (b)). All the samples clearly show the two peaks at 25.72° and 29.74° when the aging time increases to 12 h (Figure S1(c)). Therefore, the structural stability of MASnI3 is effectively enhanced after HPT processing.
Figure 2. Stability test by aging in air. XRD files of different MASnI3 samples after aging (a) 5 min and (b) 2 h.
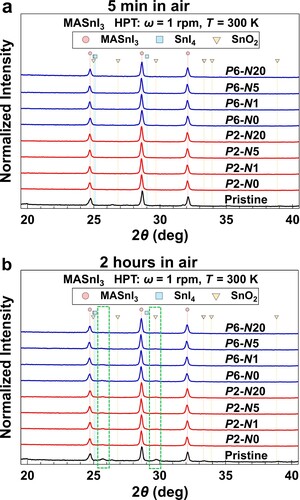
To study the microstructure of the samples before and after HPT processing, TEM observations were done on the MASnI3 powders for the pristine, P2-N0, P2-N20, P6-N0 and P6-N20 samples. In the low-magnification TEM images (Figure (a,b)), a layered structure and nanoparticles are observed in the pristine and N0 samples (Figures (a) and S2(a)), but many tube-like structures are found in N20 samples (Figures (b) and S2(d)). In Figure (c,d), HRTEM images give a clear description of the structures of the pristine and P2-N20 samples. The [110] and [111] crystal planes are indexed in the pristine MASnI3 sample and a clear tube structure is found in the P2-N20 sample. More TEM images of P2-N0, P6-N0 and P6-N20 samples are shown in Figure S2. The TEM images reveal a possible trend that tube-like structures are more likely to be formed in the samples processed after a larger number of turns. The shear strain (γ) introduced by HPT processing is given by γ = 2πrN/t [Citation47], where r is the distance from the disc center, N is the number of turns and t is the thickness of the disc. Hence, it is surmised that a large shear strain induces the transformation from layered structures and nanoparticles to tube-like structures of MASnI3 during HPT processing. The images of selected area diffraction in Figure S3 additionally reveal the increased distortion of the structures with the increasing of pressure and shear strain. The partial closed, C-tube like microstructures have a smaller active surface, which may affect electronic transportation and chemical stability.
Figure 3. Microstructure change in MASnI3 samples after HPT processing. (a) and (b) low magnification, (c) and (d) high-resolution TEM images of pristine and P2-N20 MASnI3 samples.
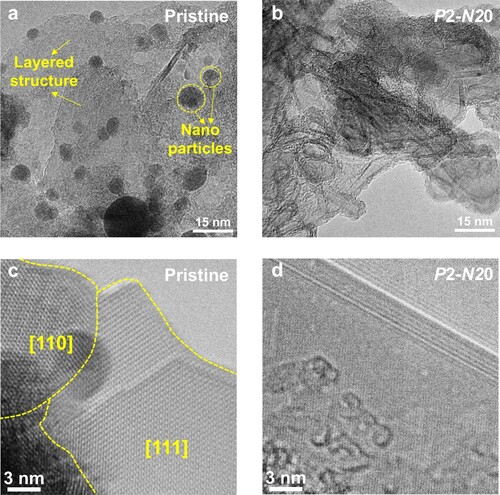
Bandgap and thermoelectric properties. The influence of HPT processing on the bandgap of MASnI3 was investigated by UV-Vis spectroscopy, as shown in Figure (a). The UV-Vis spectra show that the light absorbance increases with increasing the pressure and the number of turns. An evaluation of UV-Vis data using the Kubelka–Munk [Citation48] analysis shows that the bandgap of MASnI3 is in the range of 1.31–1.34 eV. The bandgap is estimated as 1.33 eV for the pristine MASnI3 and all the P6 samples, and for the P2 samples, it is 1.34, 1.31 and 1.32 eV after N0, N1, N5 and N20, respectively. It appears that the bandgap is little affected by the HPT processing as the difference in the measured bandgap is as minor as 0.03 eV, and there is no systematic change with respect to the HPT processing conditions.
Figure 4. Increased light absorbance and thermoelectric properties of MASnI3 samples after HPT processing. (a) UV-Vis spectra of MASnI3 samples processed under different conditions. Straight dot lines are used to estimate the bandgap. (b) Electrical conductivity and (c) Seebeck coefficient of the MASnI3 samples processed under different conditions.
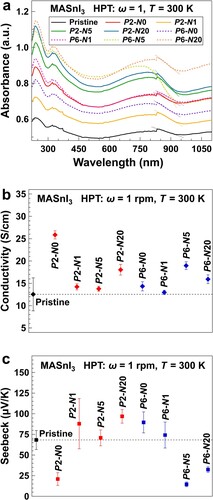
Measurements of electrical conductivity and the Seebeck coefficient are performed to characterize the thermoelectric properties of MASnI3, as shown in Figure (b,c). Electrical conductivity is invariably enhanced by the HPT processing. The Seebeck coefficient also increases by the HPT processing, except for the conditions of P2-N0, P6-N5 and P6-N20. The highest is achieved with P2-N20, which is 1.4 times as high as the pristine MASnI3. It is, therefore, suggested that the best thermoelectric property of MASnI3 is reached in the sample with a lower pressure and a large shear strain.
DFT calculations. During HPT processing, not only hydrostatic pressure but also shear strain are simultaneously introduced into samples, but it is difficult to mimic shear strain from different directions in the DFT calculations. To simplify these calculations, only hydrostatic pressure is applied in MASnI3 structures. It has also been found that the cubic model is more reasonable in the analysis of XRD data by the least-square fitting method than the tetragonal model. Therefore, hydrostatic pressure is applied in the pseudo-cubic MASnI3 model in the DFT calculations and an obvious reduction of the lattice constant is shown in Table , which is partially consistent with experimental data.
Table 1. Calculated lattice constant, cell volume and effective mass of MASnI3 under different hydrostatic pressures.
The electronic band structure of MASnI3 at 0, 2 and 6 GPa is characterized by the HSE and GGA exchange–correlation functionals, along with the DFT calculations, and given in Figures and S4. From the band plots in Figure , it is found that MASnI3 shows a direct bandgap at the R-point (0.5, 0.5, 0.5) for the samples processed under 0 and 2 GPa and at the T-point (0, 0.5, 0.5) for the 6 GPa sample. The calculated bandgaps using the HSE exchange–correlation functional are 0.953, 0.349 and 1.600 eV for MASnI3 at 0, 2 and 6 GPa, respectively, compared with the calculated bandgaps using the GGA exchange–correlation, which are 0.703, 0 and 1.389 eV at 0, 2 and 6 GPa, respectively. The bandgap initially decreases with increasing the pressure up to 2 GPa, which is due to the hybridization wave function between Sn and I atoms caused by the decrease in bond length. The distance between the neighboring Sn and I atoms becomes shorter when the pressure is increased to 6 GPa, which leads to an enhancement of Coulomb repulsion between electrons [Citation49]. The SnI6 octahedra tilts and Sn-I-Sn bond angles deviate to compensate for the increased Coulomb repulsion, which causes a weakening of the coupling effects between Sn and I and a shifting of the conduction band minimum to a high-energy edge. Thus, the bandgap subsequently continues to increase with the applied pressure of up to 6 GPa, which is consistent with that of the reported work [Citation50]. It can be explained that the bandgap of MASnI3 is affected by two factors: bandgap narrowing by Sn-I contraction and bandgap enlarging by SnI6 octahedral tilting. From the PDOS information in Figure S4, it can be known that the conduction band minimum is composed of Sn p-orbitals and I p-orbitals, while the valence band maximum is mainly composed of I p-orbitals.
Figure 5. Calculated HSE band structures and DOS of a pseudo-cubic MASnI3 model at (a) 0 GPa, (b) 2 GPa and (c) 6 GPa. The dashed lines indicate the Femi level.
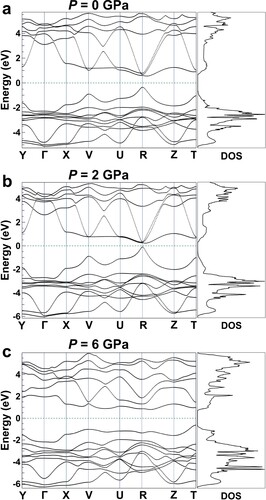
The electrical conductivity and the Seebeck coefficient are also calculated under different pressures, as shown in Figure S5. The electrical conductivity enhances after applying hydrostatic pressure and also increases with the increasing of pressure, while the absolute value of the Seebeck coefficient reduces under 2 and 6 GPa. The electrical conductivity (σ) [Citation51] and the Seebeck coefficient (S) [Citation52] are intrinsically interrelated, and they can be defined in the form of the following equations:
(1)
(1)
(2)
(2) where n, e, µ, kB, h and m* are the carrier concentration, electron charge, carrier mobility, Boltzmann constant, Planck constant and carrier effective mass, respectively.
It is considered that electrical conductivity (σ) can be improved by increasing the carrier mobility (µ). The cell volume of MASnI3 reduces under high pressure and after HPT processing, which indicates shorter Sn-I bonds. The shorter Sn-I bonds result in better orbital overlap and broader conduction and valence bands [Citation53,Citation54], which are favorable for high carrier mobility. The Seebeck coefficient (S) can be enhanced by improving the effective mass (m*) and reducing the carrier concentration (n) as shown in Equation (2). It was reported that a smaller effective mass implies a higher carrier mobility (µ), since µ is proportional to 1/m* [Citation55]. The calculated effective mass is shown in Table , where the absolute value initially decreases and then increases with increasing the applied pressure, which is the trend similar to the bandgaps. The intrinsic carrier concentration is impacted by the bandgap of the materials and large bandgap materials have a lower intrinsic carrier concentration [Citation56]. The reduction of the calculated Seebeck coefficient under 2 GPa is a result of the reduced m* and increased n, and the calculated Seebeck coefficient under 6 GPa is reduced by the decreased n and enhanced m*. Hence, there should be a competition between higher carrier mobility and lower carrier concentration to impact the Seebeck coefficient. This also leads to an enhancement or reduction of the Seebeck coefficient of HPT-processed MASnI3. Similarly, the electrical conductivity of MASnI3 is influenced by the competition between higher carrier mobility and lower carrier concentration. But the high carrier mobility dominates the enhancement of electrical conductivity after high hydrostatic pressure and HPT processing.
In summary, the HPT-processed MASnI3 shows no phase transformation but a slight change in the atomic and electronic structures. MASnI3 with tube-like structures is often observed in the TEM images of large shear strain samples. The electrical conductivity and the Seebeck coefficient increase and the thermoelectric properties slightly improve after HPT processing. The DFT calculations disclose the possible mechanism that the competition between carrier mobility and concentration impacts the electrical conductivity and Seebeck coefficient simultaneously. Combined with the increased structure stability, all these features reveal that HPT-processed MASnI3 is a potential material in thermoelectric applications. This will inspire researchers to investigate the in-situ measurement of HPT processing to unravel the effect of shear strain under high pressure on high-performance thermoelectric materials.
Supplemental Material
Download MS Word (19.4 MB)Acknowledgements
The authors wish to thank Mr. N. Wakayama of the Center for Instrumental Analysis, Kyushu Institute of Technology, for the TEM observations.
Disclosure statement
No potential conflict of interest was reported by the author(s).
Additional information
Funding
References
- Snyder GJ, Toberer ES. Complex thermoelectric materials. Nat Mater. 2008;7:105–114.
- Bell LE. Cooling, heating, generating power, and recovering waste heat with thermoelectric systems. Science. 2008;321:1457–1461.
- Mao J, Zhu H, Ding Z, et al. High thermoelectric cooling performance of n-type Mg3Bi2-based materials. Science. 2019;365:495–498.
- He W, Wang D, Wu H, et al. High thermoelectric performance in low-cost SnS0.91Se0.09 crystals. Science. 2019;365:1418–1424.
- Hao F, Stoumpos CC, Cao DH, et al. Lead-free solid-state organic-inorganic halide perovskite solar cells. Nat Photonics. 2014;8:489–494.
- Huang YQ, Su J, Li QF, et al. Structure, optical and electrical properties of CH3NH3SnI3 single crystal. Physica B: Condens Matter. 2019;563:107–112.
- Wu LJ, Zhao YQ, Chen CW, et al. First-principles hybrid functional study of the electronic structure and charge carrier mobility in perovskite CH3NH3SnI3. Chinese Phys B. 2016;25:107202.
- Feng J, Xiao B. Effective masses and electronic and optical properties of nontoxic MASnX3 (X = Cl, Br, and I) perovskite structures as solar cell absorber: a theoretical study using HSE06. J Phys Chem C. 2014;118:19655–19660.
- Sharma VK, Kanchana V, Gupta MK, et al. Ultra-low thermal conductivity of orthorhombic CH3NH3SnI3: a first principles investigation. J Solid State Chem. 2020;290:121541.
- He Y, Galli G. Perovskites for solar thermoelectric applications: a first principles study of CH3NH3AI3 (A = Pb and Sn). Chem Mater. 2014;26:5394–5400.
- Zhao T, Wang D, Shuai Z. Doping optimization of organic-inorganic hybrid perovskite CH3NH3PbI3 for high thermoelectric efficiency. Synth Met. 2017;225:108–114.
- Wu P, Xiong Y, Sun L, et al. Enhancing thermoelectric performance of the CH3NH3PbI3 polycrystalline thin films by using the excited state on photoexcitation. Org Electron. 2018;55:90–96.
- Pisoni A, Jaćimović J, Barišić OS, et al. Ultra-low thermal conductivity in organic-inorganic hybrid perovskite CH3NH3PbI3. J Phys Chem Lett. 2014;5:2488–2492.
- Zheng F, Saldana-Greco D, Liu S, et al. Material innovation in advancing organometal halide perovskite functionality. J Phys Chem Lett. 2015;6:4862–4872.
- Zeng Q, Sheng H, Ding Y, et al. Long-range topological order in metallic glass. Science. 2011;332:1404–1406.
- Sun L, Chen XJ, Guo J, et al. Re-emerging superconductivity at 48 K in iron chalcogenides. Nature. 2012;483:67–69.
- Bu K, Luo H, Guo S, et al. Pressure-regulated dynamic stereochemical role of lone-pair electrons in layered Bi2O2S. J Phys Chem Lett. 2020;11:9702–9707.
- Lü X, Wang Y, Stoumpos CC, et al. Enhanced structural stability and photo responsiveness of CH3NH3SnI3 perovskite via pressure-induced amorphization and recrystallization. Adv Mater. 2016;28:8663–8668.
- Lü X, Stoumpos C, Hu Q, et al. Regulating off-centering distortion maximizes photoluminescence in halide perovskites. Natl Sci Rev. 2021;8:nwaa288.
- Guo S, Zhao Y, Bu K, et al. Pressure-suppressed carrier trapping leads to enhanced emission in two-dimensional perovskite (HA)2(GA)Pb2I7. Angew Chem Int Ed. 2020;59:17533–17539.
- Guo S, Bu K, Li J, et al. Enhanced photocurrent of all-inorganic two-dimensional perovskite Cs2PbI2Cl2 via pressure-regulated excitonic features. J Am Chem Soc. 2021;143:2545–2551.
- Zhilyaev AP, Langdon TG. Using high-pressure torsion for metal processing: fundamentals and applications. Prog Mater Sci. 2008;53:893–979.
- Edalati K, Horita Z. A review on high-pressure torsion (HPT) from 1935 to 1988. Mater Sci Eng A. 2016;652:325–352.
- Bridgman PW. Effects of high shearing stress combined with high hydrostatic pressure. Phys Rev. 1935;48:825–847.
- Fujita I, Edalati P, Wang Q, et al. Novel black bismuth oxide (Bi2O3) with enhanced photocurrent generation, produced by high-pressure torsion straining. Scr Mater. 2020;187:366–370.
- Edalati P, Wang Q, Razavi-Khosroshahi H, et al. Photocatalytic hydrogen evolution on a high-entropy oxide. J Mater Chem A. 2020;8:3814–3821.
- Fujita I, Edalati K, Wang Q, et al. High-pressure torsion to induce oxygen vacancies in nanocrystals of magnesium oxide: enhanced light absorbance, photocatalysis and significance in geology. Materialia. 2020;11:100670.
- Wang Q, Edalati K, Fujita I, et al. High-pressure torsion of SiO2 quartz sand: phase transformation, optical properties, and significance in geology. J Am Ceram Soc. 2020;103:6594–6602.
- Wang Q, Watanabe M, Edalati K. Visible-light photocurrent in nanostructured high-pressure TiO2-II (Columbite) phase. J Phys Chem C. 2020;124:13930–13935.
- Hidalgo-Jimenez J, Wang Q, Edalati K, et al. Phase transformations, vacancy formation and variations of optical and photocatalytic properties in TiO2-ZnO composites by high pressure torsion. Int J Plast. 2020;124:170–185.
- Wang Q, Edalati K, Koganemura Y, et al. Photocatalytic hydrogen generation on low-bandgap black zirconia (ZrO2) produced by high-pressure torsion. J Mater Chem A. 2020;8:3643–3650.
- Edalati K, Wang Q, Eguchi H, et al. Impact of TiO2-II phase stabilized in anatase matrix by high-pressure torsion on electrocatalytic hydrogen production. Mater Res Lett. 2019;7:334–339.
- Razavi-Khosroshah H, Fuji M. Development of metal oxide high-pressure phases for photocatalytic properties by severe plastic deformation. Mater Trans. 2019;60:1203–1208.
- Ikoma Y. Severe plastic deformation of semiconductor materials using high-pressure torsion. Mater Trans. 2019;60:1168–1176.
- Edalati K, Fujiwara K, Takechi S, et al. Improved photocatalytic hydrogen evolution on tantalate perovskites CsTaO3 and LiTaO3 by strain-induced vacancies. ACS Appl Energy Mater. 2020;3:1710–1718.
- Valiev RZ, Islamgaliev RK, Alexandrov IV. Bulk nanostructured materials from severe plastic deformation. Prog Mater Sci. 2000;45:103–189.
- Tang Y, Murayama M, Edalati K, et al. Phase transformations in Al-Ti-Mg powders consolidated by high-pressure torsion: experiments and first-principles calculations. J Alloys Compd. 2021;889:161815.
- Tang Y, Goto W, Hirosawa S, et al. Concurrent strengthening of ultrafine-grained age-hardenable Al-Mg alloys by means of spinodal decomposition. Acta Mater. 2017;131:57–64.
- Ashida M, Sumida N, Hasezaki K, et al. Effects of low rotational speed on crystal orientation of Bi2Te3-based thermoelectric semiconductors deformed by high-pressure torsion. Mater Trans. 2012;53:588–591.
- Ashida M, Hamachiyo T, Hasezaki K, et al. Effect of high-pressure torsion on crystal orientation to improve the thermoelectric property of a Bi2Te3-based thermoelectric semiconductor. Adv Mat Res. 2010;89-91:41–46.
- Edalati K, Uehiro R, Takechi S, et al. Enhanced photocatalytic hydrogen production on GaN-ZnO oxynitride by introduction of nitrogen vacancy complexes. Acta Mater. 2020;185:149–156.
- Nishizaki T, Edalati K, Lee S, et al. Critical temperature in bulk ultrafine-grained superconductors of Nb, V and Ta processed by high-pressure torsion. Mater Trans. 2019;60:1367–1376.
- Mito M, Shigeoka S, Kondo H, et al. Hydrostatic compression effects on fifth-group element superconductors V, Nb and Ta subjected to high-pressure torsion. Mater Trans. 2019;60:1472–1483.
- Smidstrup S, Markussen T, Vancraeyveld P, et al. QuantumATK: an integrated platform of electronic and atomic-scale modelling tools. J Phys Condens Matter. 2020;32:015901.
- The Materials Project. Materials data on LiTaO3 by materials project [dataset]. 2020 Jul 16. Available from: https://doi.org/https://doi.org/10.17188/1207210.
- Quarti C, Grancini G, Mosconi E, et al. The Raman spectrum of the CH3NH3PbI3 hybrid perovskite: interplay of theory and experiment. J Phys Chem Lett. 2014;5:279–284.
- Valiev RZ, Estrin Y, Horita Z, et al. Producing bulk ultrafine-grained materials by severe plastic deformation. JOM. 2006;58:33–39.
- Kubelka P, Munk F. An article on optics of paint layers. Z Tech Phys. 1931;12:593609.
- Tan M, Wang S, Rao F, et al. Pressures tuning the band gap of organic-inorganic trihalide perovskites (MAPbBr3): a first-principles study. J Electron Mater. 2018;47:7204–7211.
- Zhang R, Cai W, Bi T, et al. Effects of nonhydrostatic stress on structural and optoelectronic properties of methylammonium lead bromide perovskite. J Phys Chem Lett. 2017;8:3457–3465.
- Tan G, Zhao LD, Kanatzidis MG. Rationally designing high-performance bulk thermoelectric materials. Chem Rev. 2016;116:12123–12149.
- Cutler M, Leavy J, Fitzpatrick R. Electronic transport in semimetallic cerium sulfide. Phys Rev. 1964;33:A1143–A1152.
- Ma Y, Eremets M, Oganov AR, et al. Transparent dense sodium. Nature. 2009;458:182–185.
- Peter Y, Cardona M. Fundamentals of semiconductors: physics and materials properties. Berlin: Springer Science & Business Media; 2010.
- Kittel C. Introduction to solid state physics. Hoboken (NJ): Wiley; 2005.
- Altermatt PP, Schenk A, Geelhaar F, et al. Reassessment of the intrinsic carrier density in crystalline silicon in view of band-gap narrowing. J Appl Phys. 2003;93:1598–1604.