Abstract
The synthesis of coordination polymers (e.g. Prussian blue) is as old as modern chemistry itself, but never stops surprising people. We here report superconductivity observed of a designer coordination polymer system sporting the chemically soft mercaptan and hard carboxyl groups. The mercapto-carboxyl (dubbed Mercarb, or QiuSuo in Mandarin Pinyin) synergy hints at Daoism and here carries over from the molecular to the solid state: the soft sulfur donors bond with Co2+/Ni2+ (or other transition metal ions) to afford 2D sheets for charge transport, while the interlayer metal-carboxylate domain is more ionic and mediates the transition into the superconductive state.
GRAPHICAL ABSTRACT
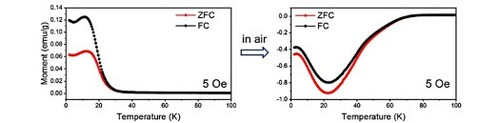
IMPACT STATEMENT
A versatile open layered platform for potential superconductivity, this coordination polymer system is suited for doping: micropores for O2 oxidation, and μ2-OH2 units to effect charge balance (by forming μ2-OH−).
The two major classes of superconductors of cuprates [Citation1] and iron pnictides [Citation2] both feature covalent charge carrier layers alternating with more ionic layers (e.g. Ln-O) as charge reservoirs. In a formal sense, the chemically soft, polarizable Fe-P or Fe-As layer, in juxtaposition to the chemically hard Ln-O layer, is reminiscent of our long-standing pursuit in synergizing the soft mercaptan (–SH) with the hard carboxyl functions for coordination network design (as in the dfdmt and DMBD molecules) [Citation3–6]. Ideally, to parallel the lamellar character of inorganic superconductors, one would hope for the polarizable metal-thiolate links to enable the charge transport layer, and the ionic metal-carboxylate domain to serve as charge reservoir for modulating the conductive property. However, perhaps due to the proximity of the thiol and carboxyl groups, such spatial and functional division into layered motifs has not been achieved, and the coordination solids had been found to be semiconductive [Citation3,Citation7] instead of superconductive. Incidentally, in Lu’s semiconducting layered structure [Citation8], the carboxylate and the thiolate layers appear to be too separate. In general, superconductivity has been observed in coordination networks only at very low temperatures (e.g. 0.25 K) [Citation9]. Here, part with work, part with luck, we have accessed an integrated layered motif in a new class of coordination polymers from the molecules of dfdmt (2,5-difluoro-3,6-dimercaptoterephthalic acid) and DMBD (2,5-dimercapto-1,4-benzenedicarboxylic acid, Scheme 1), and we are pleased to report the preliminary yet encouraging evidence for their superconductivity behaviors (e.g. with a critical temperature Tc above 50 K achieved already at this opening stage).
The coordination polymers of Ni-dfdmt, Co-dfdmt, CoNi-dfdmt, Co-DMBD and CoNi-DMBD share the formula Co2−xNix(C8F2S2O4)(H2O)2 or Co2−xNix(C8H2S2O4)(H2O)2 (x = 0, 1 or 2), and similar crystalline structure (as indicated by powder X-ray diffraction patterns; Figures and ) [Citation10]. All five were prepared from heating in a sealed glass tube (140°C) the respective linker molecule (H4dfdmt or H4DMBD) and the metal salts (e.g. NiCl2·6H2O), together with water and DMF as the mixed solvent. For illustration, we here describe the crystal structure of Ni-dfdmt (Figure ), which has been solved by the MicroED (microcrystal electron diffraction) method (see also SI). Ni-dfdmt is triclinic (P-1; a = 3.3200, b = 8.440, c = 9.650 Å, α = 74.48, β = 84.29, γ = 84.27°), and features two types of octahedrally coordinated Ni ions (Figure ): Ni1 is chelated by the thiol and carboxyl groups, with four equatorial S atoms (Ni-S distances: 2.360 and 2.272 Å) and two apical O atoms (Ni-O distance: 2.008 Å); by sharing two opposite equatorial edges (i.e. with the μ2-S atoms straddling two Ni atoms), straight rows of edge-sharing octahedra is formed along the a axis, and these integrate the benzenoid units into a hybrid metal-thiolate sheet (Figure B). The remaining carboxyl O atoms (these are not coordinated to Ni1) protrude on both sides of the layer and bond to the two apical sites of the Ni2 ion (Ni-O distance: 1.961 Å). Ni2 is also bonded to four μ2-aqua units (Ni-O distances: 2.132 and 2.117 Å), featuring, parallel to the Ni1 chains, rows of edge-sharing octahedra. The Ni2-O chains almost fill up the interlayer space, with only small opening (<3 Å) existing between the layers. The Ni1-thiolate layer and the Ni2-O domain thus respectively correspond to the covalent/conducting part and the ionic part in the inorganic superconductors.
Figure 1. Crystal structure of Ni-dfdmt. (A) An overview along the a axis; (B) A 2D Ni-thiolate network viewed along the c axis. H atoms on the aqua sites are omitted for clarity. Grey spheres: C; red: O; cyan: F; yellow: S; green: Ni.
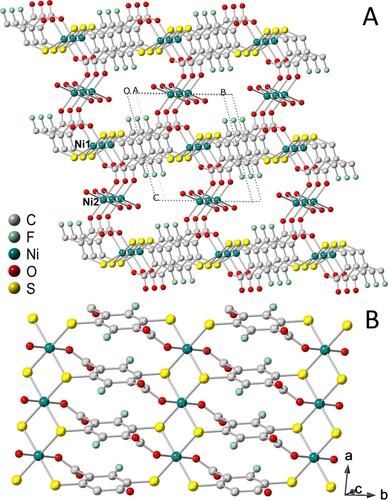
Figure 2. PXRD patterns (Cu Kα, λ = 1.5418 Å) of: (a) Ni-dfdmt as calculated from the crystal structure; (b) Ni-dfdmt; (c) Co-dfdmt; (d) CoNi-dfdmt, (e) Co-DMBD; (f) CoNi-DMBD. The diffraction profiles are not sensitive to air exposure.
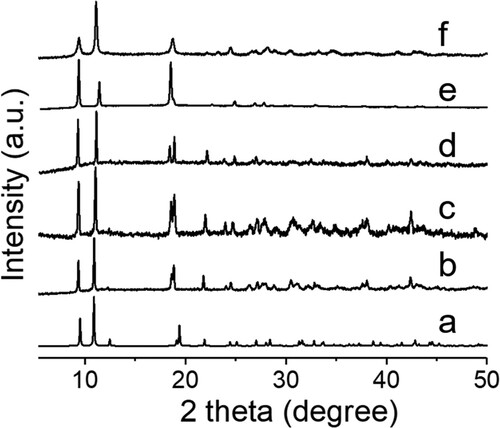
The Ni-dfdmt solid has not been as clearly found to be superconductive yet, while Co-dfdmt, CoNi-dfdmt, Co-DMBD and CoNi-DMBD all exhibit strong diamagnetism indicative of superconductors. Figure shows their magnetic susceptibilities (χ) as a function of temperature, with those of CoNi-DMBD-10 h exhibiting the highest critical temperature (Tc). Under the external magnetic field H = 5 Oe (field cooling; FC), the susceptibility starts to decrease at about 55 K and then quickly descends into a value of −0.58 emu g−1 at 16 K, followed by slight increase to peak at 7 K. In the zero-field cooling (ZFC) process, the susceptibility starts to decrease slowly at about 60 K, but the sharp descent occurs at a similar temperature (about 50 K) as the FC plot, reaching −0.81 emu g−1 at 16 K, followed by slight increase to peak at 7 K. It is not clear yet if this small hump at the cold end is caused by some phase transition or by impurity. A complete shielding (4Π χ < −1) is observed, indicating a high-quality superconducting phase. From the inflection point of the χ–T plots, we tentatively ascribe the superconductivity transition temperature (Tc) to be about 50 K for this sample of CoNi-DMBD-10 h. The distinct diamagnetic transitions are consistently observed of different batches of samples in repeated tests. For illustration, we include in the SI the ZFC and FC data for the temperature dependence of magnetic susceptibility at 10 Oe (Figure S7).
Figure 3. Temperature dependence of magnetic susceptibility for the solid samples of Co-dfdmt (30 h, 14.7 mg), CoNi-dfdmt (20 h, 11.3 mg), Co-DMBD (20 h, 9.2 mg) and CoNi-DMBD (10 h, 10.5 mg). Data are shown for zero-field cooled (ZFC) and field-cooled (FC) measurements at 5 Oe. The number of hours refer to the air exposure time of the powder sample.
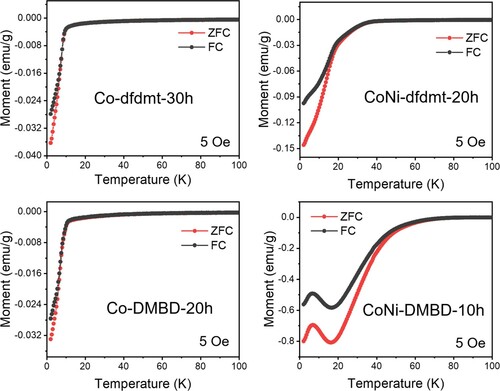
Air exposure is crucial for inducing the superconductivity properties. Namely, the fresh, as-made samples exhibit positive magnetization with long-range ferromagnetic transitions at low temperatures (e.g. below 40 K, Figure S8); while the air-exposed samples of Figure generally display diamagnetism throughout, which can be ascribed to doping (by air oxidation) and the itinerant nature of the Co(II) d electrons in these semiconductors (above the superconductive Tc). Generally, doping semiconductors is known to decrease the effective mass of the charge carriers, so that the Landau diamagnetism becomes dominant over the Pauli paramagnetism [Citation11]. Air exposure time also affects the superconductive Tc: as shown in Figure a–c, CoNi-DMBD-46 h (46-hour exposure) already exhibits diamagnetic increase above 70 K, pointing to a higher Tc than the CoNi-DMBD-10 h sample; while too long exposure may over-dope the system, not helping the superconductivity properties (e.g. 168-hour exposure does not further improve the Tc, as shown by the CoNi-DMBD-168 h data in Figure ). The ripening rate is, however, likely subject to the crystallite sizes/surface area and other sample conditions.
Figure 4. Panels a–c: Temperature dependence of magnetic susceptibility for the solid samples of CoNi-DMBD (10 h, 10.5 mg), CoNi-DMBD (46 h, 10.7 mg) and CoNi-DMBD (168 h, 11.2 mg). Data are shown for zero-field cooled (ZFC) and field-cooled (FC) measurements at 5 Oe. Panels d–f: Temperature dependence of resistivity of CoNi-DMBD (14 h), CoNi-DMBD (29 h) and CoNi-DMBD (192 h). Resistivity measurements were performed using a two-probe setup. The inset amplifies the 0–100 K region. The number of hours refer to the air exposure time of the powder sample. The values of mm refer to the thickness of the pellet.
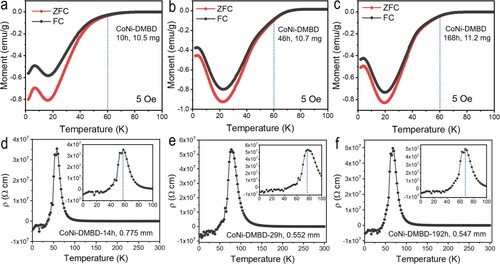
The temperature dependence of the electric resistivity (ρ) of a pressed pellets of air-exposed CoNi-DMBD powder samples has been measured by the two-probe setup (Figure d–f). As shown in Figure d, the resistivity of CoNi-DMBD-14 h exhibits an accelerated growth, reaching an inflection point at about 80 K with a steep upshoot indicative of an emerging insulator state to peak at 55 K, immediately followed by a sharp drop to cross the zero point and stay at the negative region. The sharp descent of resistivity suggests an insulator-to-superconductor transition [Citation12], possibly with the Cooper pairs formed first being localized (insulating), followed by global phase coherence of the Cooper pairs (bosons) occurring to effect the superconductive state. The negative resistivity is also observed of the longer-exposed CoNi-DMBD samples (29 and 192 h, see Figure e–f), which exhibit higher peak resistance temperatures (paralleling the higher Tc values seen in the magnetic susceptibility data, e.g. cf. Figure b,e). Is the negative resistance due to some junction effect (e.g. as uneven, gradient air exposure/doping results in the superconductive grains/components being interfaced by ferromagnet or insulator boundaries/components, or some other interplay between superconductivity (e.g. from the metal-thiolate layer) and ferromagnetism (e.g. from the interlayer metal-carboxyl chains)? We are still working for an answer. For this, four-probe measurement and broad-range I-V curve measurement (these are being conducted) will be informative, as compared with the current PPMS setup, in which the resistance is determined only from some small range of excitation currents applied to the sample.
Author contributions
J.H. and Z.X. designed and supervised the experiments and measurements. Z.L. and L.T. prepared the coordination polymers. Z.X. saw the link to superconductivity, urged the tests, analyzed the data and wrote the paper. X.Y. measured the magnetic and conductive properties. J.H. and J.Y. also co-edited the paper.
Supplemental Material
Download MS Word (1.5 MB)Acknowledgements
We thank Dr. Xiaofang Lai and Dr. Danfeng Li for helpful discussions on the resistance data.
Disclosure statement
No potential conflict of interest was reported by the author(s).
Additional information
Funding
References
- Bednorz JG, Müller KA. Possible high Tc superconductivity in the barium-lanthanum-copper-oxygen system. Z Physik B: Condensed Matter. 1986;64(2):189–193.
- Kamihara Y, Hiramatsu H, Hirano M, et al. Iron-based layered superconductor: LaOFeP. J Am Chem Soc. 2006;128(31):10012–10013.
- He J, Yang C, Xu Z, et al. Building thiol and metal-thiolate functions into coordination nets: clues from a simple molecule. J Solid State Chem. 2009;182(7):1821–1826.
- Zeng Q, Wang L, Huang Y, et al. An air-stable anionic two-dimensional semiconducting metal-thiolate network and its exfoliation into ultrathin few-layer nanosheets. Chem Commun. 2020;56(25):3645–3648.
- He J, Cheng S, Xu Z. Sulfur chemistry for stable and electroactive metal-organic frameworks: the crosslinking story. Chem Eur J. 2019;25:8654–8662.
- Xu Z. Uniting form and function. Stability and reactivity in open framework materials. Chem Lett. 2021;50(4):627–631.
- Sun L, Miyakai T, Seki S, et al. Mn2(2,5-disulfhydrylbenzene-1,4-dicarboxylate): a microporous metal-organic framework with infinite (-Mn-S-)∞ chains and high intrinsic charge mobility. J Am Chem Soc. 2013;135(22):8185–8188.
- Pathak A, Shen J-W, Usman M, et al. Integration of a (-Cu-S-)n plane in a metal-organic framework affords high electrical conductivity. Nat Commun. 2019;10(1):1–7.
- Huang X, Liu L, Xu W, et al. Superconductivity in a copper(II)-based coordination polymer with perfect kagome structure. Angew Chem Int Ed. 2018;57(1):146–150.
- A patent based on this study has been filed to the China National Intellectual Property Administration (on Feb. 15, 2022): 202210137451.4.
- Ashcroft N, Mermin N. Solid state physics. Fort Worth: Saunders College Publishing; 1976. p. 666.
- Phillips P, Dalidovich D. The elusive bose metal. Science. 2003;302(5643):243–247.