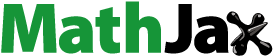
Abstract
Hydrogel electrolytes, which feature intriguing characteristics of being soft and wet, accompanied with high solubility of zinc salts, are especially advantageous for fabricating flexible Zn-based batteries. Furthermore, the abundant and tunable chemistries of hydrogels offer opportunities to bestow novel affiliated functionalities, such as super toughness, extreme temperature tolerance, stimuli-responsive properties, and self-healing capability, by engineering polymer chains and chemical structures to fabricate flexible batteries with unprecedentedly scintillating functions. Herein, the design principles of functional hydrogel electrolytes including polymer chemistry, synthesis methods, characterisation and versatility demonstration for flexible Zn-based batteries are comprehensively reviewed. Relevant challenges and perspectives are also analysed and discussed.
GRAPHICAL ABSTRACT

IMPACT STATEMENT
This review provides a comprehensive understanding for recent advances of flexible Zn-based batteries with hydrogel electrolytes, with a focus on smart batteries with multifunctionalities. Challenges and perspectives for future wearable applications are also discussed.
1. Introduction
In recent years, flexible batteries have received great research interest as the development of wearable intelligent electronics, which are predicted to become indispensable in our future lives owing to their vast applied foreground [Citation1–3]. Ideal flexible battery systems are expected to possess the merits of stable electrochemical properties, small size, and mechanical adaptability to frequent deformations that wearables may encounter in practical usage. Although Li-ion batteries (LIBs) dominate the market at present and have been identified to be promising for flexible batteries owing to their high voltage, superior energy density and mature manufacturing technologies, the safety issues derived from the flammable and toxic organic electrolytes have seriously hindered the research progress of flexible LIBs for wearable applications [Citation4,Citation5]. Therefore, significant efforts to transfer the accumulated experience into the research frontier of alternative batteries with inherent safety and high electrochemical performance for flexible batteries are highly expected [Citation6,Citation7].
In consideration of safety issues of flexible battery design, rechargeable aqueous Zn-based batteries are particularly advantageous for developing flexible and wearable batteries by virtue of their inherent safety, good chemical stability, cost-effectiveness and satisfactory energy density compared with traditional LIBs [Citation8–11]. However, there are still some persistent challenges existed in rechargeable aqueous Zn-based batteries: (1) Zn dendrites would inevitably occur in the aqueous electrolyte environment when zinc is directly used as anode, leading to short circuit [Citation12]. (2) The aqueous electrolyte may arise side reactions of hydrogen evolution reaction (HER) and oxygen evolution reaction (OER), causing the exhaustion of water and capacity deterioration [Citation13]. (3) Some cathode materials for Zn-based battery would be dissolved in the aqueous solution to varying extent, resulting in a gradual decline in battery performance [Citation14–16]. Fortunately, these issues can be alleviated by using hydrogel electrolytes. Due to their unique quasi-solid-state characteristics that combining the merits of aqueous and solid electrolytes, hydrogel electrolytes can not only effectively prevent the dissolution of active materials and the leakage of liquid but also suppress the growth of Zn dendrites to some extent. The water-saturated hdyrogel matrix can dissolve various salt ions, guaranteeing the ion conduction required for the electrochemical reaction. Moreover, the mechanical strength enables the hydrogel electrolyte to be directly used as a separator to avoid short circuits; meanwhile, the soft and moist interfaces of hydrogel electrolytes can contact intimately with electrodes. Benefited from the aforementioned merits, hydrogel electrolytes exhibit good compatibility with flexible Zn-based batteries.
The traditional hydrogel electrolytes are dominantly based on poly(acrylamide) (PAAm/PAM), polyacrylic acid (PAA), polyvinyl alcohol (PVA), sodium polyacrylate, gelatin, and so on [Citation17]. However, these hydrogel electrolytes only provide physical frameworks to support the ion transportation, which could not provide considerable improvements in electrochemical performance and additional functions to satisfy the needs of diverse practical scenarios. The development of polymeric hydrogel electrolytes is still at the primary stage, and the number of available optional approaches is limited. In practical application, flexible zinc-based batteries are vulnerable to extreme working conditions, especially the hydrogel electrolytes are more susceptible to ambient environment due to their water-saturated nature and relative fragility compared to electrodes [Citation18]. Firstly, during daily use, the flexible batteries have a large chance to encounter local stress and extreme deformation, which may cause deadly breaking and battery failure [Citation19]. Secondly, while under subzero temperatures, nullification of hydrogel electrolytes would happen due to the freezing of water in hydrogel matrix and the decrease of ionic conductivity [Citation20]. Thirdly, water inevitably evaporates from inner hydrogel matrix even at room temperature, and higher temperature would accelerate the dehydration process, leading to the battery performance deterioration [Citation21]. These aforementioned issues are still a stumbling block to the popularisation of flexible batteries in practical applications. To this end, by engineering the polymer chains, such as grafting functional groups, modifying hydrogel network, or doping additives, novel hydrogels with affiliated functionalities have been developed and become a main theme, leading to a new revolution in the research progress of flexible batteries [Citation22,Citation23].
In recent years, substantial achievements of smart flexible batteries with multifunctionality, which are referred to that one battery not only possesses the intrinsic energy storage function but also features other intriguing and practical functions, such as extreme deformations tolerance [Citation24,Citation25], environmental adaptability involving anti-freezing and anti-dehydration properties [Citation26,Citation27], and external stimuli responsiveness like thermo-responsiveness and self-healing [Citation23,Citation28–30], have been achieved through the precise design of functional hydrogel materials based on polymer chemistry and polymer engineering technologies, opening up exciting opportunities for developing versatile power systems [Citation31,Citation32]. In the following sections, flexible Zn-based batteries based on hydrogel electrolyte are summarised and discussed with a focus on multifunctional systems, hoping to enlighten the future research directions.
2. Fundamentals of hydrogel electrolytes
Hydrogel materials are a kind of elastic crosslinked hydrated polymer that swollen with tunable water. This structure feature endows the polymeric matrices with unique characteristics of being both soft and wet, and the containing water can dissolve mobile electrolyte ions for supporting electrochemical reactions [Citation33,Citation34]. Nowadays, hydrogel electrolytes have been intensively studied and considered as ideal candidates of electrolyte materials for flexible batteries owing to their good compatibility with electrodes and high ionic conductivity. What is more, the abundant and tunable molecular groups on the polymer chains can be modified and designed elaborately via polymer chemistries and polymer engineering technology, which allow the introduction of intriguing functionalities into existing hydrogel materials. Therefore, it is feasible and exciting to fabricate unprecedented hydrogel electrolyte-based batteries with multiple affiliated functions.
2.1. Traditional hydrogel electrolytes
In hydrated hydrogel matrix, the dissolved electrolytic ions can be attracted and localised by the surrounding charged functional groups on the polymeric chains in the network. In addition, the interstitial spaces within the elastic crosslinked network can be filled with a significant amount of water for guaranteeing the flexibility of hydrogel matrix [Citation35,Citation36]. These particular features endow the hydrogel materials with a unique liquid-like ionic conductivity and meanwhile preserve the dimensional stability of quasi-solid state, making them as ideal candidates for flexible batteries.
The ionic conductivity of hydrogels is generally related to the kind and the concentration of zinc salts, as well as the water content of the hydrogel matrix. Besides, under high salt and water saturation, diver hydrogel materials will display different characteristics and ionic conductivity. Some representative hydrogel materials used for fabricating electrolyte are summarised in , which systematically presents their molecular structures, general characteristics and ionic conductivity for energy storage applications. Typically, the most popularly used homopolymers for hydrogel electrolyte synthesis are PVA, PAA and PAAm/PAM, which contain abundant hydrophilic groups of hydroxyl, carboxylic and amide groups on the side chains, respectively [Citation37]. The formation of the crosslinking networks of hydrogel matrix through these three polymeric hosts is relatively different. For instance, the PVA can be intrinsically crosslinked by physical H-bonding or through chemical crosslinking agents like glutaraldehyde and borax, which can endow the pristine rigid PVA hydrogel with excellent stretchability benefiting from the reversible interaction [Citation38–40]. By contrast, PAA and PAAm hydrogels are normally crosslinked by the chemical crosslinking originated from double bonds through free radical polymerisation. Furthermore, the abundant carbonyl and hydroxyl groups on the polymer side chains can endow the monomers with a great potential of gelation by hydrogen bonding and good affinities with aqueous electrolytes [Citation41,Citation42]. In addition, sodium polyacrylate, gelatin and xanthan gum are common thickener agents that contain long chains of monosaccharide/peptide units. As a result, these hydrogel monomers are easy to change into hydrogel state so that they can exert effectiveness as quasi-solid-state electrolyte for energy storage applications.
Table 1. Structural features of the hydrogel materials.
2.2. Functional hydrogel electrolytes
With the development of polymer synthetic technology and polymer chemistry, it is feasible to design hydrogel materials with distinctive properties. Typical synthesised methods such as materials selection, self-assembly, crosslinking points introduction, construction of interpenetrating polymeric network and other gelation methods could be employed to realise specific functionalities involving rubbery stretchability, self-healing ability and thermo-sensitivity [Citation34,Citation53,Citation54]. In addition, the intrinsic ionic conductivity, interface compatibility and mechanical flexibility of the designed hydrogel electrolytes should be also carefully considered to guarantee the electrochemical properties (Figure (a)). For instance, the dual-network crosslinking design can endow the original weak hydrogels with a high rubbery mechanical elasticity compared with non-crosslinked ones, showing both super tough yet soft features. Sun et al. [Citation25] reported a super tough PAAm-alginate hydrogel with hierarchical dual-network crosslinked structure, in which the first network consisted of chemically covalent-crosslinking PAAm chains could dissipate extensive mechanical energy during various deformations, while the second physical ionic bonds-crosslinked alginate network was responsible to the recovery of the deformation. The resultant dual-network crosslinked hydrogel exhibited superior stretchability with fractural energy of 9000 J m−2, outperforming the common elastic hydrogels. Liu et al. [Citation24] fabricated a super tough Al3+-crosslinked alginate/PAAm hydrogel electrolyte and successfully applied it into supercapacitor, which displayed extraordinary ductility against extreme deformations, as presented in Figure (b,c).
Figure 1. (a) Design principles of the functional hydrogel electrolytes. (b) Schematic structure of the super tough Al3+-alginate/PAAm hydrogel. (c) Mechanical characterisations of the super tough hydrogel. Reproduced with permission from reference [Citation24]. (d) Fabrication and molecular structure of the glycerol–water hydrogel. (e) Anti-freezing performance of the glycerol–water hydrogel. Reproduced with permission from reference [Citation27]. (f) Mechanism of bonding dissimilar polymer networks by using silane coupling agents. (g) Deep-frying hydrogels with/without elastomer coating in mineral oil at 120°C. Reproduced with permission from reference [Citation56]. (h) Working mechanism of the thermoresponsive hydrogel electrolyte via sol–gel transition. (i) Photographs of the reversible sol–gel transition of the thermoresponsive hydrogel electrolyte during heating and cooling cycles. Reproduced with permission from reference [Citation57]. (j) Schematic structure and demonstration of the self-healing PAA-based hydrogel. (k) Self-healing mechanism of the PAA-based hydrogel electrolyte and its ionic conductivity after multiple cutting/healing cycles. Reproduced with permission from reference [Citation64].
![Figure 1. (a) Design principles of the functional hydrogel electrolytes. (b) Schematic structure of the super tough Al3+-alginate/PAAm hydrogel. (c) Mechanical characterisations of the super tough hydrogel. Reproduced with permission from reference [Citation24]. (d) Fabrication and molecular structure of the glycerol–water hydrogel. (e) Anti-freezing performance of the glycerol–water hydrogel. Reproduced with permission from reference [Citation27]. (f) Mechanism of bonding dissimilar polymer networks by using silane coupling agents. (g) Deep-frying hydrogels with/without elastomer coating in mineral oil at 120°C. Reproduced with permission from reference [Citation56]. (h) Working mechanism of the thermoresponsive hydrogel electrolyte via sol–gel transition. (i) Photographs of the reversible sol–gel transition of the thermoresponsive hydrogel electrolyte during heating and cooling cycles. Reproduced with permission from reference [Citation57]. (j) Schematic structure and demonstration of the self-healing PAA-based hydrogel. (k) Self-healing mechanism of the PAA-based hydrogel electrolyte and its ionic conductivity after multiple cutting/healing cycles. Reproduced with permission from reference [Citation64].](/cms/asset/d89311d5-ece9-4b7b-8112-852fc5de1c59/tmrl_a_2059412_f0001_oc.jpg)
Almost all of the hydrogel materials cannot resist extremely cold environment that both the ionic conductivity and mechanical softness will seriously deteriorate due to the freezing of water in hydrogel matrix [Citation55]. To this end, many strategies have been proposed to depress the freezing point of hydrogel electrolytes by limiting the formation of intermolecular hydrogen bonding among free water molecules in the cold condition, such as introducing polyol/water binary solvent and hydrated ions from salt solutions. For example, glycerol, a well-known nontoxic anti-freezing agent, was introduced into hydrogel matrix by using the glycerol–water binary solvent to improve low-temperature tolerance of traditional PAA-PAM hydrogel materials (Figure (d)). The freezing temperature of the glycerol–water hydrogel was highly dependent on the volume percentage of glycerol (Figure (e)) [Citation27]. Besides, dehydration is another critical issue for hydrogel materials. This is because that even in the ambient environment, water will inevitably evaporate from inner hydrogel matrix, seriously affecting the long-term stability of hydrogels. Integrating a hydrophobic elastomer layer onto the surface of hydrogels by a silane coupling agent-assisted approach can effectively prevent the water evaporation from hydrogel and the exchange of solutes (Figure (f)) [Citation56]. As a result, the elastomer coating enables hydrogels to survive elevated temperatures in mineral oil when even heated at 120°C (Figure (g)).
Apart from extreme temperature tolerance, thermoresponsive copolymer-based hydrogel materials have been developed and applied in the field of energy storage, such as the poly(isopropylacrylamide)-based microgels with the intriguing property of sol–gel transition (Figure (h)). This kind of hydrogel is normally in the flowing liquid state at/or below room temperature, while upon heating above critical temperature, it can transform into stationary gel state, displaying interesting temperature-dependent properties (Figure (i)) [Citation57]. This reversible sol–gel transition provides a new perspective for thermal self-protection strategy in electrochemical storage devices.
In addition, to improve the reliability and long-term usability of hydrogel materials, modification of polymer chemistry can bestow hydrogels with intriguing self-healability, which is referred to a feature that a broken material can automatically heal the damaged parts to its pristine state. Typically, self-healing mechanisms of healable hydrogels in many published articles can be clarified as hydrogen bonding [Citation58], supramolecular interactions [Citation59,Citation60], molecular diffusion [Citation61], and reversible ionic interactions [Citation62,Citation63]. For example, owing to the formation of abundant hydrogen bonding by the interactions of carbonyl and hydroxyl groups, the crosslinked PAA-based hydrogel presents efficient self-healing property (Figure (j)) [Citation64]. After a couple of repeated cutting/healing cycles, both the ionic conductivity and decent mechanical performance were well retained, showing its promising application in flexible energy storage (Figure (k)). The aforementioned functional hydrogel materials have been used as quasi-solid-state electrolytes in designing smart energy storage, endowing them with different functionalities to satisfy the needs of diverse practical scenarios.
3. Hydrogel electrolytes for flexible Zn-based batteries
3.1. Aqueous rechargeable Zn-based batteries
An aqueous Zn-based battery, in particular, consists of Zn anode, cathode, aqueous electrolyte and a separator, which can be classified into primary alkaline system and rechargeable system. Energy storage mechanisms are related to the selection of electrolyte and cathode materials. For instance, with the alkaline liquid electrolyte, a proton-induced conversion reaction generally occurs in the Mn–/Ni–/Co–/air-based cathode, while the Zn anode undergoes a stripping/plating process (), as illustrated in Figure (a). Due to the alkaline Zn-based battery is a primary system that would continuously consume Zn during operation, the utilisation efficiency of zinc resources is relatively low. Moreover, the irreversible side reactions during electrochemical process in alkaline system usually result in rapid capacity fading [Citation65].
Figure 2. Schematics of working principle and basic structure of (a) alkaline Zn-based batteries and (b) rechargeable aqueous Zn-based batteries.
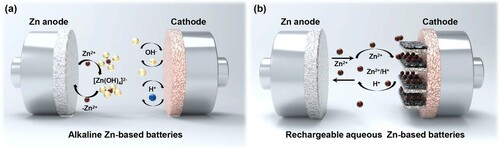
In addition, the mechanism of Zn metallic anode for rechargeable Zn-based batteries in neutral or mild acidic electrolytes depends on the reversible dissolution-deposition of Zn2+ (). Meanwhile, the electrochemical reaction in cathodes can be classified into four categories: Zn2+ intercalation/extraction, H+/Zn2+ co-insertion, chemical conversion reaction, and ionic coordination (Figure (b)). Taking the intercalation/extraction mechanism as example, during the discharging process, the Zn dissolves into electrolyte from anode and soluble Zn2+ transfers through electrolyte and inserts into cathode materials. While during the charging process, Zn2+ inversely extracts from cathode materials to electrolyte. If Zn2+ ions gained electrons in the electrolyte, they would plate onto the surface of Zn anode [Citation66].
While metallic Zn is directly utilised as anode of ZIBs, irregular Zn dendrites are easily formed during the charging process, resulting in battery performance degradation and short circuiting. Moreover, some persistent challenges exist in the aqueous liquid electrolyte involving limited voltage window caused by HER and OER, which would lead to the consumption of water and capacity deterioration [Citation67,Citation68]. In addition, some cathode materials, such as manganese-based oxides [Citation69–71], vanadium-based oxides [Citation72,Citation73], and Prussian blue analogues [Citation10,Citation74], would be dissolved in the aqueous electrolyte to the varying extent, resulting in a gradual decline in battery performance. These aforementioned problems can be resolved by using hydrogel electrolytes to improve the electrochemical performance. Hydrogel electrolyte can not only effectively suppress the Zn dendrite growth but also alleviate the dissolution of active materials owing to their unique quasi-solid state with the limited water content. Furthermore, its intrinsic safety, chemical stability, and mechanical flexibility make the hydrogel electrolyte particularly advantageous for constructing flexible and wearable Zn-based batteries [Citation75,Citation76].
3.2. Flexible Zn-based batteries with hydrogel electrolytes
The replacement of liquid-state electrolyte with gel polymer electrolyte to fabricate solid-state rechargeable Zn-based batteries highly boosts their applications as flexible power accessories for various portable/wearable electronics. In particular, owing to its intrinsic safety, eco-friendliness, softness, and good compatibility, hydrogel electrolytes are particularly advantageous for flexible aqueous Zn-based battery, which has been considered as a promising alternative to traditional flammable aprotic battery systems in wearable electronics. In this section, the recent advances of flexible Zn-based batteries using hydrogel electrolyte are briefly reviewed and discussed with a highlight on the potential research directions for future.
3.2.1. Thin-film flexible Zn-based batteries
For conventional batteries with sandwiched layered structure containing electrodes, electrolyte and separator, the use of hydrogel electrolyte of replacing the liquid electrolyte highly boosts aqueous batteries for wearable applications [Citation77,Citation78]. The hydrogel electrolyte can not only provide ionic conductivity for supporting electrochemical behaviours but also serve as a reliable separator sandwiched between electrodes to avoid short circuit. For instance, Zeng et al. [Citation79] proposed a novel flexible Zn–MnO2@poly(3,4-ethylenedioxythiophene)(PEDOT) battery based on a freestanding PVA/ZnCl2/MnSO4 hydrogel electrolyte, accompanied with the soft MnO2@PEDOT and Zn-deposited carbon cloth serving as electrodes, which exhibited a high specific capacity of 366.6 mAh g−1 at the current density of 0.74 A g−1, as well as a maximum energy density of 504.9 Wh kg−1 and power density of 8.6 kW kg−1, respectively (Figure (a)). As mechanical durability demonstrations, when the flexible battery was subjected to various deformations, such as bending and twisting, the discharging profile could keep almost invariant. For wearable applications, three flexible Zn–MnO2@PEDOT batteries were connected in series to illuminate a neon sign with 45 light-emitting diodes (LEDs) and a wristwatch with LED lights (Figure (b,c)). These results highly demonstrated the promising potential of the hydrogel electrolyte-based flexible battery as the next-generation energy storage for powering wearable electronics. In addition, Li and coworkers [Citation80] also fabricated a flexible Zn–MnO2 battery with PVA–ZnCl2–MnSO4 hydrogel electrolyte, in which MnO2 nanorod arrays and Zn nanoparticles were deposited onto N-doped porous carbon cloths to obtain the cathode and anode. Benefiting from the superior ionic conductive PVA-based hydrogel electrolyte, the resultant flexible Zn–MnO2 battery exhibited admirable energy storage behaviours of high energy density (440 Wh kg−1) and high power density (7.9 kW kg−1), as well as superior cyclic stability. Furthermore, the battery also displayed excellent mechanical flexibility against diverse bending deformations with well-preserved electrochemical performances.
Figure 3. (a) Schematic illustration of the configuration of flexible Zn–MnO2@PEDOT battery. Digital photographs of three flexible Zn–MnO2@PEDOT batteries connected in series powering (b) a neon sign with 45 LEDs and (c) a watch with LED lights. Reproduced with permission from reference [Citation79]. (d) Schematic illustration of the structure of the extremely safe and wearable solid-state ZIB. (e) Cutting test of the flexible solid-state ZIB. (f) Demonstrations of the wearable applications of flexible solid-state ZIBs. Reproduced with permission from reference [Citation81].
![Figure 3. (a) Schematic illustration of the configuration of flexible Zn–MnO2@PEDOT battery. Digital photographs of three flexible Zn–MnO2@PEDOT batteries connected in series powering (b) a neon sign with 45 LEDs and (c) a watch with LED lights. Reproduced with permission from reference [Citation79]. (d) Schematic illustration of the structure of the extremely safe and wearable solid-state ZIB. (e) Cutting test of the flexible solid-state ZIB. (f) Demonstrations of the wearable applications of flexible solid-state ZIBs. Reproduced with permission from reference [Citation81].](/cms/asset/f17c43e8-ac4b-4a81-a760-f05759d130ca/tmrl_a_2059412_f0003_oc.jpg)
What is more, an extremely safe and wearable rechargeable Zn–MnO2 battery fabricated by a designed hierarchical polymer electrolyte (HPE) was developed (Figure (d)) [Citation81]. In this work, the HPE hydrogel was synthesised by in situ polymerising PAM and gelatin onto a polyacrylonitrile (PAN) membrane, which exhibited an exceptional mechanical strength and a high ionic conductivity of 1.76 × 10−2 S cm−1. The resultant flexible Zn–MnO2 battery equipped with HPE delivered a high specific capacity of 306 mAh g−1 at a current density of 61.6 mA g−1, as well as high areal energy and power density of 6.18 mWh cm−2 and 148.2 mW cm−2, respectively. By virtue of HPE with strong mechanical strength, the flexible Zn–MnO2 battery achieved exceptional safety and environmental adaptability, which could maintain stable electrochemical performances while being bent, cut, hammered, punctured and sewed (Figure (e)). For wearability demonstration, three batteries connected in series could work as an energy wristband to power a smart watch and be integrated into a commercial smart insole to monitor the parameters for sport analysis, as illustrated in Figure (f), showing the great potential applications for portable and wearable electronics.
Besides the Zn–MnO2 chemistry, a flexible nickel-zinc battery (Ni-Zn battery) was proposed by Liu et al. [Citation43] prepared by utilising ZnO nanoparticles as a negative electrode material and NiO nanoflake-deposited carbon cloth-carbon fibre as a positive electrode material, accompanied with a PVA–KOH gel as the electrolyte. The resulting flexible Ni-Zn battery exhibited a high energy density of 323.3 Wh kg−1, which are comparable to that of 355.7 Wh kg−1 of the contrastive battery with liquid electrolyte. In addition, the flexible Ni-Zn battery delivered a high specific capacity of 0.35 mAh cm−2 with a good cyclic stability of 91.45% capacity retention after 1000 cycles. In the mechanical test, the flexible Ni-Zn battery could well maintain electrochemical performances upon being bent to different conditions, showing its remarkable mechanical and electrochemical stability.
3.2.2 Fibre/yarn-shaped flexible Zn-based batteries
The fibre-shaped batteries with unique 1D architecture is another common configuration of flexible batteries. Typically, the use of intrinsically soft hydrogel electrolytes, which can be easily coated onto the surface along a fibre electrode, significantly simplifies the coating and hermetic sealing process. Moreover, due to its good mechanical flexibility and stability, the hydrogel electrolyte can not only act as a reliable separator to avoid short circuiting and leakage but also serve as a protective framework to prevent the exfoliation of cathode materials from the 1D fibre substrate during varying deformations of the fibre-shaped batteries, highly satisfying the requirements of flexible electrolytes [Citation82,Citation83].
Recently, many high performance fibre-shaped Zn-based batteries equipped with hydrogel electrolytes have been proposed and arouse great research interests. For example, a tailorable yarn-shaped Zn–MnO2 battery based on a PAM hydrogel electrolyte has been constructed, in which two double-helix CNT fibres were coated with α-MnO2 and Zn to use as the cathode and anode, respectively (Figure (a)) [Citation46]. This crosslinked PAM-based hydrogel electrolyte was swollen in a mixed solution containing ZnSO4 and MnSO4 and thus exhibited a high ionic conductivity of 17.3 × 10−3 S cm−1 (Figure (b)). The resultant yarn-shaped Zn–MnO2 battery delivered a high specific capacity of 302.1 mAh g−1 with superior stability of capacity retention over 98.5% after 500 cycles. As wearable demonstration, an energy fabric woven by a 1.1 m long fibre-shaped battery was fabricated to power a long LED belt and various electroluminescent panels (Figure (c)).
Figure 4. (a) Fabrication process of the yarn-shaped Zn–MnO2 battery. (b) Synthesis and molecular structure of the crosslinked PAM-based electrolyte. (c) Photographs of eight yarn-shaped Zn–MnO2 batteries connected in series to power various electroluminescent panels and a long LED belt. Reproduced with permission from reference [Citation46]. (d) Schematic structure of the weavable yarn-shaped NiCo/Zn battery. (e) Demonstration of the industrial weavability and knittability of the textile batteries. (f) Wearable demonstrations of the textile batteries being woven into a wrist band. Reproduced with permission from reference [Citation84]. (g) Schematics of the structure and fabrication of the fibre-shaped Ag–Zn battery with PVA electrolyte. Adapted from Arias et al. [Citation85]. (h) Schematics of the fabrication of the fiber-shaped Zn–air battery with NiCo2O4@N-OCNT-based air electrode and a gel electrolyte. Reproduced with permission from reference [Citation86].
![Figure 4. (a) Fabrication process of the yarn-shaped Zn–MnO2 battery. (b) Synthesis and molecular structure of the crosslinked PAM-based electrolyte. (c) Photographs of eight yarn-shaped Zn–MnO2 batteries connected in series to power various electroluminescent panels and a long LED belt. Reproduced with permission from reference [Citation46]. (d) Schematic structure of the weavable yarn-shaped NiCo/Zn battery. (e) Demonstration of the industrial weavability and knittability of the textile batteries. (f) Wearable demonstrations of the textile batteries being woven into a wrist band. Reproduced with permission from reference [Citation84]. (g) Schematics of the structure and fabrication of the fibre-shaped Ag–Zn battery with PVA electrolyte. Adapted from Arias et al. [Citation85]. (h) Schematics of the fabrication of the fiber-shaped Zn–air battery with NiCo2O4@N-OCNT-based air electrode and a gel electrolyte. Reproduced with permission from reference [Citation86].](/cms/asset/1e530ce4-e81c-4396-bc0d-f62b4c035593/tmrl_a_2059412_f0004_oc.jpg)
Apart from the Zn–MnO2 chemistry, a yarn-shaped nickel/cobalt–zinc (NiCo/Zn) battery equipped with PVA-based hydrogel electrolyte was developed, which delivered high energy density of 0.12 mWh cm−2 and ultrahigh rate capability of 116 C (Figure (d)) [Citation84]. In this research, ultrathin stainless steel 316L filaments were employed as yarn substrates to fabricate highly conductive electrodes (Figure (e)). The PVA-based gel electrolyte provided strong adhesion to electrodes, offering the assembled yarn batteries with excellent stability to sustain extreme deformations. The resultant yarn-shaped NiCo/Zn battery could achieve over 70% retention of the initial capacity under consecutive deformation of bending and twisting for over 1000 times. For wearable application, an energy wristband was fabricated by waving the battery fibres into textile to serve as energy fabric to power a digital watch, a LED belt, or a pulse sensor (Figure (f)). In addition, to extend the battery lifetime, Zamarayeva et al. [Citation85] applied the 2 m potassium hydroxide (KOH)-PVA-based hydrogel electrolyte into the silver-zinc (Ag–Zn) battery to alleviate the dissolution of silver ions from electrode into electrolyte (Figure (g)). A fibre-shaped Ag–Zn battery with coaxial structure was constructed, which exhibited a 170 stable cycle life with high volumetric energy density of 53.4 Wh L−1.
Furthermore, flexible fibre-shaped zinc-air batteries (ZABs) are also in rapid development, which possess ultrahigh theoretical energy density (1086 Wh kg−1). For instance, Zeng et al. [Citation86] developed a fibre-shaped ZAB by a PVA–KOH–Zn(OAc)2 gel electrolyte and a freestanding multifunctional air electrode prepared by adhering NiCo2O4 nanosheets onto the surface of the nitrogen and oxygen dual-doped CNT (N-OCNT) films (Figure (h)). The assembled flexible fibre-shaped ZAB delivered stable discharging and charging potentials of 1.1 and 1.8 V, respectively. To demonstrate the mechanical flexibility and stability, two fibre-shaped ZABs with the length of 20 cm were connected in series to illuminate an LED in straight, wavy bending, 90° folded, and spiral deformation states.
4. Advanced flexible Zn-based batteries with functional hydrogel electrolytes
Although intensive efforts have been devoted for the fundamental innovations of flexible Zn-based batteries, it is still difficult to satisfy the needs of various practical scenarios, such as flexible/wearable demand, comfortness and durability, safety requirements, and weavability. In recent years, advanced Zn-based batteries with functional hydrogel electrolytes are receiving increasing research interest for providing smart functionalities originated from polymer chemistry research. In other words, apart from electrochemical energy storage functions, those functional batteries are capable to endure or respond to external stimuli so that rendering them versatility for complicated operating environment [Citation3,Citation87]. To this end, functional hydrogel materials should be elaborately designed and integrated into a single device to achieve smart functionalities. In the following sections, recent advances in the Zn-based batteries with special functions equipped with smart and functional hydrogel electrolytes are summarised and discussed.
4.1. Super tough and super stretchy Zn-based batteries
To facilitate the long-term usage of flexible and wearable batteries, mechanical durability of device-level toughness is essential, which involves both electrochemical and mechanical stabilities against external dynamic stimuli. Towards this target, a super tough flexible Zn–MnO2 battery was developed, comprising a highly durable alginate-based hydrogel electrolyte with an ionically crosslinked network structure (Figure (a)) [Citation88]. In this research, the synthesised super tough hydrogel consisted of the interpenetrating network, in which one network is the chemically crosslinked PAM matrix and the other is the Zn2+-crosslinked alginate ionic network. These two polymeric networks were concordantly interacted with each other against the extremely induced or released external stress during deformation, highly facilitating the mechanical durability of the hydrogel electrolyte, as illustrated in Figure (b). The resultant super tough Zn–MnO2 battery displayed high capacity retention of 82% after 500 cycles at 0.88 A g−1. When put a super tough battery in shoe and imposed the pressure, its capacity increased due to the reduction of interlayer distance between electrodes. More impressively, even after the extreme compact of unceasing run-over by 20 random cars, this super tough Zn–MnO2 battery still displayed stable electrochemical performance without notable deterioration in capacity (Figure (c)). While the dissociated multivalent ions are contacted with the guluronic acid groups of alginate molecular chains, substantial ionic crosslinks would be formed where metallic ions are confined within the periodic chelation sites between the alginate chains, thus forming the interpenetrating network. With the increase of the valence of the dissociated metallic ions, the crosslinking degree of the molecular chains in hydrogel matrix that formed between the multivalent ions and the guluronic acid units of alginate would increase, which significantly enhance its toughness.
Figure 5. (a) Schematic diagrams of the structural evolution of PAM-alginate-based super tough hydrogel electrolyte. (b) Energy dissipation mechanism of the super tough hydrogel electrolyte. Reproduced with permission from reference [Citation88]. (c) The performance of super tough Zn–MnO2 battery tested under foot treading or even under car run-over. (d) Synthesis of the super stretchy PANa hydrogel electrolyte. (e) Schematics of the assembly of the intrinsically super stretchy NiCo/Zn battery. (f) Capacity enhancement ratio of the intrinsically super stretchy battery from 0% to 400% strain. Reproduced with permission from reference [Citation49].
![Figure 5. (a) Schematic diagrams of the structural evolution of PAM-alginate-based super tough hydrogel electrolyte. (b) Energy dissipation mechanism of the super tough hydrogel electrolyte. Reproduced with permission from reference [Citation88]. (c) The performance of super tough Zn–MnO2 battery tested under foot treading or even under car run-over. (d) Synthesis of the super stretchy PANa hydrogel electrolyte. (e) Schematics of the assembly of the intrinsically super stretchy NiCo/Zn battery. (f) Capacity enhancement ratio of the intrinsically super stretchy battery from 0% to 400% strain. Reproduced with permission from reference [Citation49].](/cms/asset/274403be-7009-4c1e-a1df-8f5ac3fac5a0/tmrl_a_2059412_f0005_oc.jpg)
To accommodate dynamic motions and substantial deformation in realistic wearable applications, flexible battery with intrinsic stretchability is also significant for modern wearable electronics. In this regard, a super stretchy NiCo/Zn battery was proposed based on a sodium polyacrylate (PANa) hydrogel electrolyte, with using the deposited zinc nanosheets and nickel cobalt hydroxide on Au@CNT papers as anode and cathode, respectively (Figure (d,e)) [Citation49]. The synthesised PANa hydrogel electrolyte exhibited an exceptional intrinsic stretchability even up to 1700% strain. The resulting flexible batteries exhibited high capacity retention of 86% after 340 cycles with the Coulombic efficiencies maintained about 93% during repeated cycles. Even under 400% stretching state, the battery still performed well and retained a capacity retention of 87% at 23 A g−1 after 500 strain cycles by virtue of the high mechanical density of the PANa hydrogel matrix, verifying the excellent mechanical and electrochemical stabilities (Figure (f)).
4.2. Low-temperature tolerant Zn-based batteries
For the Zn-based batteries with hydrogel electrolyte working at subzero temperatures, it is imperative to suppress the freezing of water in hydrogel, maintaining both the mechanical flexibility and high ionic conductivity [Citation89,Citation90]. There are two common strategies to depress the freezing point of hydrogel electrolytes: (1) Introducing additives including hydrated ions or polyol solvent into hydrogel matrix to break the intermolecular H-bonding between free water molecules; (2) strengthening the interactions such as grafting hydrophilic functional groups between hydrogel networks and surrounding water molecules to reduce the free water content. For example, Wang et al. [Citation20] proposed a concentrated PANa hydrogel electrolyte, which could enable NiCo//Zn battery to work at −20°C (Figure (a)). After being immersed in the high concentrated (6 M) KOH solution, the freezing temperature of PANa hydrogel significantly decreased due to the existence of concentrated ions (K+, OH− and Zn2+) decimating the H-bonding between water molecules. This concentrated hydrogel electrolyte could be easily stretched by 900% even in the extremely cold condition of −50°C. Moreover, its ionic conductivity maintained a high level of 5.7 S m−1 at −20°C. As a result, the flexible NiCo//Zn battery equipped with the concentrated hydrogel delivered a high capacity of 130 mAh g−1 with high capacity retention of 87% after 10,000 cycles. Besides the concentration, various kinds of zinc salts also significantly affect the freezing temperature of hydrogel electrolytes. Liu et al. [Citation91] found that even at the same concentration of 3 M, the PAm hydrogel electrolyte with the ZnCl2 exhibited better freeze tolerance than that with ZnSO4 owing to the higher ion concentration (Figure (b)). The anti-freezing PAm hydrogels with varying geometries displayed superior freestanding and flexible capacity, as shown in Figure (c).
Figure 6. (a) The molecular structure and stretchability properties of the highly concentrated PANa hydrogel electrolyte. Reproduced with permission from reference [Citation20]. (b) Photographs of PAM hydrogels containing various zinc salts at room temperature and −20°C. (c) Photographs of PAM hydrogels with different geometries in different states. Reproduced with permission from reference [Citation91]. (d) Schematics of the synthesis process and multicomplexation of the 3D network structure of PVA-B-G hydrogel. Reproduced with permission from reference [Citation92]. (e) Molecular models of various hydrogels and the simulate interactions between different terminal groups in polymer chains and water molecules. (f) Photographs of the flexible ZAB working at −20°C under various deformation states. Reproduced with permission from reference [Citation90]. (g) Schematics of the strong hydrogen bonds among EG-waPUA, PAM and water molecules in the AF-gel. (h) Freeze-resistant performance of the AF-battery working at subzero temperatures. Reproduced with permission from reference [Citation93]. (i) Synthesis of the anti-freezing CT3G30 hydrogel electrolyte. (j) Photographs of CT3G30 with different geometries in different states. Reproduced with permission from reference [Citation94].
![Figure 6. (a) The molecular structure and stretchability properties of the highly concentrated PANa hydrogel electrolyte. Reproduced with permission from reference [Citation20]. (b) Photographs of PAM hydrogels containing various zinc salts at room temperature and −20°C. (c) Photographs of PAM hydrogels with different geometries in different states. Reproduced with permission from reference [Citation91]. (d) Schematics of the synthesis process and multicomplexation of the 3D network structure of PVA-B-G hydrogel. Reproduced with permission from reference [Citation92]. (e) Molecular models of various hydrogels and the simulate interactions between different terminal groups in polymer chains and water molecules. (f) Photographs of the flexible ZAB working at −20°C under various deformation states. Reproduced with permission from reference [Citation90]. (g) Schematics of the strong hydrogen bonds among EG-waPUA, PAM and water molecules in the AF-gel. (h) Freeze-resistant performance of the AF-battery working at subzero temperatures. Reproduced with permission from reference [Citation93]. (i) Synthesis of the anti-freezing CT3G30 hydrogel electrolyte. (j) Photographs of CT3G30 with different geometries in different states. Reproduced with permission from reference [Citation94].](/cms/asset/c04d0946-6202-4464-98f3-84fed972096e/tmrl_a_2059412_f0006_oc.jpg)
Apart from hydrated ions, polyol solvents including glycerol and ethylene glycol (EG) are also effective additives used as cryoprotectants for hydrogel electrolytes to improve their freezing tolerance. For instance, an anti-freezing borax-crosslinked PVA/glycerol (PVA-B-G) hydrogel was proposed and successfully applied into the flexible Zn–MnO2 battery [Citation92]. The PVA-B-G hydrogel was synthesised by the facile freeze/thaw method, in which abundant hydrogen bonds was formed among glycerol, PVA and water molecules, constructing integrated 3D networks, as illustrated in Figure (d). The PVA-B-G hydrogel displayed a remarkable mechanical strength against twisting, bending and even being stretched to 400–500% strain at extremely cold temperature of −35°C. In addition, the PVA-B-G hydrogel electrolyte possessed a high ionic conductivity of 10.1 mS cm−1, and the assembled Zn–MnO2 battery exhibited 90% capacity retention after 2000 cycles at −35°C. In the future research, hydrated ions and polyol are expected to combine their synergistic effects to further improve the freeze tolerance of the hydrogel electrolyte.
Hydrogel network modification, such as introducing hydrophilic groups (–OH, –NH2, –COOH, etc.) to interact with water molecules to form strong bound water, can significantly decrease the freezing temperature of hydrogel. Pei et al. [Citation90] developed an alkalified PAA hydrogel containing 10 wt% KOH solution, which showed a freezing point of −25°C. Through the density functional theory calculation, the calculated interaction energy (Eint) of the carboxyl group with water molecules in PAA was calculated to be −12.92 kcal mol−1 in its pristine state and increased to −16.96 kcal mol−1 after the hydrogel was alkalified, resulting in better freezing tolerance (Figure (e)). Consequently, the ionic conductivity of the anti-freezing hydrogel electrolyte reached 199 mS cm−1 at −20°C, and the assembled flexible ZAB retained outstanding electrochemical performances and low-temperature adaptability that they could sustain being bent, folded or twisted at −20°C (Figure (f)). In addition, Mo et al. [Citation93] proposed an EG-based waterborne anionic polyurethane acrylate/PAM (EG-waPUA/PAM) hydrogel electrolyte, in which the hydrophilic group hydroxyl from EG was introduced and firmly anchored onto the hydrogel networks by covalent bonding. This strong interaction between water molecules and the hydrophilic groups could prevent the growth of ice crystallisation within EG-waPUA/PAM hydrogel matrix (Figure (g)). As a result, the anti-freezing hydrogel (AF-gel) could maintain high ionic conductivity of 14.6 mS cm−1, as well as remarkable durability and flexibility against adverse conditions like bending, compression and hammering at −20°C. The Zn–MnO2 battery based on AF-gel electrolyte presented a high discharge capacity of 226 mAh g−1 at −20°C, whereas the counterpart pure PAM battery failed nearly (Figure (h)).
To further decrease the freezing temperature of hydrogel electrolytes to satisfy all requirements while operating under all the extreme conditions, Chen et al. [Citation94] designed all-around hydrogel electrolytes for Zn–MnO2 batteries, which could adapt all extreme conditions. In this work, cellulose was applied as a hydrogel substrate and glycerol was added as an anti-freezing agent (Figure (i)). In the hydrogel matrix, the crosslinker tetraethyl orthosilicate (TEOS) was able to be covalently bound with glycerol and cellulose to construct a stable 3D structure. Furthermore, the hydroxyl groups on cellulose chains formed sufficient reversible hydrogen bonding, which could interact with water molecules intimately, and thus, the water activity was reduced. The resultant hydrogel (CT3G30) exhibited an extremely low freezing temperature of −64.6°C and ultrahigh stretchy capability of 846.5% elongation (Figure (j)). The CT3G30 hydrogel electrolyte exhibited a high ionic conductivity of 19.4 mS cm−1 at −40°C, and the assembled Zn–MnO2 battery delivered a high specific capacity of 211.8 mAh g−1 and stable electrochemical performances towards extreme environments. Besides, the CT3G30 hydrogel also contributed to suppression of Zn dendrites and side reactions, showing great potential for flexible batteries.
4.3. Dehydration tolerant Zn-based batteries
In general, water inevitably evaporates from the hydrogel matrix under ambient conditions, and higher temperature will further accelerate this process, which seriously hinders the long-term usability of hydrogel electrolytes. For the zinc-based batteries, especially ZABs that are more susceptible to dehydration due to its half-open configuration, it is highly desired to alleviate water evaporation for maintaining the long-term stability of battery performance. Adding polyol-based agents can effectively improve the water retention ability of hydrogel electrolytes and have been already applied in flexible ZABs. For instance, Chen et al. [Citation21] introduced various concentrations of glycerol into PAM/PAA hydrogel and thus successfully suppressed water loss from hydrogel matrix (Figure (a)). Even after baking at 70°C for 12 h, the hydrogel could keep its volume almost unchanged and still tightly adhere to both the air electrode and the Zn anode with good interfacial contact (Figure (b)). Benefitting from the admirable anti-dehydration property of the glycerol-based hydrogel electrolyte, the assembled ZAB delivered high capacity retention of 96.63% even at 70°C on comparing with the value tested at room temperature of 640.92 mAh g−1 (Figure (c)).
Figure 7. (a) Schematic illustration of the glycerol-based PAM/PAA hydrogel with abundant hydrogen bonding interactions within the polymer chains. (b) Adhesion of the glycerol-based PAM/PAA hydrogel after baking at 70°C for 12 h. (c) A photograph of the battery continuously powering a LED light at 70°C. Reproduced with permission from reference [Citation21]. (d) Synthesis route of the PANa hydrogel electrolyte. (e) Comparison of the real-time water retention of various hydrogels. (f) Long-term cycling performances of the Zn//NiCo battery equipped with the PANa hydrogel electrolyte. Reproduced with permission from reference [Citation23]. (g) Schematic synthesis of porous PVA-based nanocomposite GPE and assembly of the flexible ZAB. (h) Water retention of the nanocomposite PVA hydrogel. (i) GCD curves of various GPEs with a duration of 20 min per cycle. Reproduced with permission from reference [Citation32]. (j) Schematic synthesis of the KI–PVAA–GO GPE and configuration of the assembled flexible batteries. Reproduced with permission from reference [Citation31]. (k) Schematic illustration of the fabrication strategy of the environmental adaptive battery. Reproduced with permission from reference [Citation97].
![Figure 7. (a) Schematic illustration of the glycerol-based PAM/PAA hydrogel with abundant hydrogen bonding interactions within the polymer chains. (b) Adhesion of the glycerol-based PAM/PAA hydrogel after baking at 70°C for 12 h. (c) A photograph of the battery continuously powering a LED light at 70°C. Reproduced with permission from reference [Citation21]. (d) Synthesis route of the PANa hydrogel electrolyte. (e) Comparison of the real-time water retention of various hydrogels. (f) Long-term cycling performances of the Zn//NiCo battery equipped with the PANa hydrogel electrolyte. Reproduced with permission from reference [Citation23]. (g) Schematic synthesis of porous PVA-based nanocomposite GPE and assembly of the flexible ZAB. (h) Water retention of the nanocomposite PVA hydrogel. (i) GCD curves of various GPEs with a duration of 20 min per cycle. Reproduced with permission from reference [Citation32]. (j) Schematic synthesis of the KI–PVAA–GO GPE and configuration of the assembled flexible batteries. Reproduced with permission from reference [Citation31]. (k) Schematic illustration of the fabrication strategy of the environmental adaptive battery. Reproduced with permission from reference [Citation97].](/cms/asset/4d7b6441-91f2-45dc-bc06-d3407b014ae5/tmrl_a_2059412_f0007_oc.jpg)
Hydrogel network modification of introducing various hydrophilic groups into polymer chains is also an effective strategy of dehydration prevention for hydrogel electrolyte. For example, Huang et al. [Citation23] developed a PANa hydrogel, which featured a strong water retention capacity, to alleviate water evaporation from hydrogels (Figure (d)). Due to the higher interaction energy (Eint) between the carboxyl groups on the PANa chains and water molecules, the PANa hydrogel exhibited 97.3% water retention even after being exposed to the ambient condition for 185 h without salt precipitation, outperforming the counterpart PVA hydrogel with only 77.6% retention. As a result, the resultant Zn//NiCo battery and ZAB equipped with PANa hydrogel electrolyte displayed an elongated lifespan of unprecedented 16,000 h and 800 cycles, respectively (Figure (e,f)).
Uniformly doping nanoparticles, such as inorganic particles [Citation32] and metallic ceramic oxides [Citation95,Citation96] within polymeric network, is a common approach to modify inner structural network of hydrogel electrolyte, which can significantly enhance the water retention capacity of hydrogel matrix. As an example, Fan et al. [Citation32] researched and found that doping silica (SiO2) with suitable weight ratio into PVA hydrogels could effectively alleviate the water evaporation (Figure (g)). That was because that with existence of SiO2 (5 wt%), the crystallinity of the PVA matrix would reduce, and more PVA chains interacted with free water molecules, thus enhancing water retention ability (Figure (h)). The flexible ZAB equipped with nanocomposite PVA hydrogel displayed a two times longer discharging lifespan than that with pure PVA hydrogel (Figure (i)). In addition, graphene oxide (GO) could also be introduced and dispersed uniformly into PAA/PVA hydrogel matrix to form a composite system PVAA-GO [Citation31]. The incorporated GO could interact with the surrounding hydrophilic groups on PAA/PVA chains and thus constructed a more intact spongy-shaped hydrogel network (Figure (j)). The sufficient interspace within the porous matrix highly facilitated the water holding of the PVAA-GO hydrogel that it could maintain 90% water content after 12 h, outperforming the PVA hydrogel with only 64.7 wt% water retention. By these improvements, the assembled ZAB could work steadily for 200 h benefitting from the water retarding property of PVAA-GO hydrogel electrolyte.
The fundamental reason of hydrogel dehydration is the exposure of hydrogel surface to the outside ambient environment, where water continuously evaporates from inner hydrogel matrix. To this end, the use of encapsulation layer coated on the hydrogel surface can avoid the water loss for a physical perspective. For instance, a hydrophobic polydimethylsiloxan (PDMS) elastomer was coated onto the surface of a PAM hydrogel by using silane coupling agents, which enabled the hydrogel with excellent water retention property. By integrating electrodes inside hydrogel electrolyte, an environmental adaptive Zn–MnO2 battery was fabricated, in which the embedded electrode intimately contacted with hydrogel matrix, and meanwhile, the outer PDMS elastomeric layer could effectively prevent water evaporation at the same time (Figure (k)) [Citation97]. As a result, the environmental adaptive battery exhibited stable battery performances that it could continuously power an electronic watch even being placed in boiling water (100°C).
4.4. Thermoresponsive Zn-based batteries
Besides temperature tolerance ability, some smart Zn-based batteries with interesting environmental adaptive functions have also been realised, which show an interesting thermoresponsive sol–gel transition behaviour. In these polyelectrolytes, there are normally existing both hydrophilic and hydrophobic molecular chains inside the hydrogel matrix. At low temperatures, the copolymer shows high water solubility due to the stronger hydrogen bonding with surrounding water molecules than the hydrophobic part, thus the copolymer display a quasi-liquid state that can flow freely. Upon increasing the temperature, the hydrophobic part gradually plays the main role that the molecular chains would be gradually separated from water phase, leading to the destruction of hydrogen bonds and the precipitation of gels. In general, this sol–gel transition phenomenon is reversible. For instance, Zhao et al. [Citation98] proposed a smart flexible ZIB with cooling recovery ability originated from the thermo-reversible sol–gel transition behaviour of the temperature-sensitive pluronic hydrogel electrolyte (PHE), as shown in Figure (a). At normal temperature such as 25°C, the PHE exhibited a hydrophobic gel state, whereas upon cooling to below −5°C, PHE would transfer into the hydrated sol state. This special sol–gel transition characteristic was reversible and thus employed to repair the fractured interface between electrodes and electrolyte induced by extreme deformation via a simple cooling process (Figure (b,c)). The assembled flexible Zn ion batteries consisted of Zn/LiFePO4 (Zn/LFP) or Zn/LiMn2O4 (Zn/LMO)-based electrodes and the PHE exhibited superior cyclic stability. Furthermore, when this thermoresponsive battery was subjected to extreme deformations, the broken area on electrode could be healed by a fast gel to sol transition of PHE upon cooling within 5 min observed by SEM images, realising a highly efficient restoration of electrochemical performance. This thermo-reversible sol–gel transition electrolyte is enlightening significance for facilitating the development of self-recovery flexible energy storage devices.
Figure 8. (a) Cooling recovery function of the thermoresponsive pluronic hydrogel electrolyte. (b) A photograph of the flexible thermo-reversible battery under strong folding. (c) Top-viewed SEM images of the cooling-recovery process of the broken Pluronic-coated electrode area cooling at −5°C. Reproduced with permission from reference [Citation98]. (d) Schematic illustration of the thermoresponsive behaviour of the Zn–MnO2 battery with PNA electrolyte based on sol–gel transition. (e) Thermal self-protection behaviour of the thermoresponsive Zn–MnO2 battery as displayed in charge–discharge cycles at different temperatures. Reproduced with permission from reference [Citation99]. (f) Working mechanism of the thermal self-protective ZIB equipped with hygroscopic hydrogel electrolyte. (g) Demonstration of the reversibility of the thermoresponsive ZIBs. Reproduced with permission from reference [Citation100].
![Figure 8. (a) Cooling recovery function of the thermoresponsive pluronic hydrogel electrolyte. (b) A photograph of the flexible thermo-reversible battery under strong folding. (c) Top-viewed SEM images of the cooling-recovery process of the broken Pluronic-coated electrode area cooling at −5°C. Reproduced with permission from reference [Citation98]. (d) Schematic illustration of the thermoresponsive behaviour of the Zn–MnO2 battery with PNA electrolyte based on sol–gel transition. (e) Thermal self-protection behaviour of the thermoresponsive Zn–MnO2 battery as displayed in charge–discharge cycles at different temperatures. Reproduced with permission from reference [Citation99]. (f) Working mechanism of the thermal self-protective ZIB equipped with hygroscopic hydrogel electrolyte. (g) Demonstration of the reversibility of the thermoresponsive ZIBs. Reproduced with permission from reference [Citation100].](/cms/asset/634878e2-9ae2-4611-ae8d-baf37b6bd090/tmrl_a_2059412_f0008_oc.jpg)
As illustrated in Figure (d), another thermoresponsive polyelectrolyte of poly (Nisopropylacrylamide-co-Acrylic acid) (PNA) with reversible sol–gel transition function was also reported, which had been applied for thermal runaway prevention [Citation99]. Similarly, this PNA sol–gel electrolyte was normally in the flowing liquid state at room temperature and transformed into stationary gel state at high temperature, displaying an interesting temperature-dependent behaviour. The assembled thermoresponsive Zn–MnO2 battery could work well at low temperature due to the high ionic conductivity of the PNA polyelectrolyte in the sol state. While heating up to a critical temperature, the PNA polyelectrolyte transformed into stationary hydrogel state and thus inhibited the Zn ion transmission, resulting in the shutting down of the battery (Figure (e)). This kind of thermoresponsive electrolyte provides a new perspective for developing dynamically thermal self-protection battery systems.
With the use of thermoresponsive hydrogel, a new self-protection strategy imitating sweating has been proposed and applied for flexible Zn–MnO2 batteries (Figure (f)) [Citation100]. In this research, the inner moisture of PAAm hydrogel matrix has been balanced to the surrounding environment by adjusting the ZnCl2 concentration, which could keep the mass unchanged for over 10 days. While the temperature increased, the moisture equilibrium was disturbed that over 40 wt% water quickly evaporated from the inside hydrogel similar as sweating. Therefore, the overheated battery could be cooled down as the water evaporated and even shut down automatically as a result of the inhibition of ion transmission. As cooling back to room temperature, the hydrogel electrolyte could reabsorb moisture to its initial state and thus recovered the high electrochemical performances of the degraded batteries, which showed the high reversibility for long-term usage (Figure (g)). However, the relatively low reabsorption efficiency and time consumption in the environment of low RH need further improvement for facilitating the development of this fancy strategy.
4.5. Self-healing Zn-based batteries
In practical use, wearable batteries are inevitable to be damaged especially after repeated deformations. Therefore, the endowment of self-healing to the impaired batteries to realise restoration of both mechanical and electrical performance is attracting great research interest. Abundant efforts have been devoted to the exploration of self-healable polyelectrolyte for developing self-healing battery. For example, Huang et al. [Citation44] proposed a self-healing all-in-one integrated ZIB based on a self-healable Zn(CF3SO3)2/PVA hydrogel electrolyte prepared by a freeze/thaw strategy (Figure (a)). In this research, a Zn foil and polyaniline (PANI) nanorod were used as anode and cathode, respectively, and subsequently incorporated into hydrogel matrix to assemble the all-in-one integrated ZIB. The hydrogel electrolyte showed excellent self-healing property with a strong interfacial strength attaching the electrodes. The resultant flexible battery delivered a specific capacity of 77.2 mAh g−1 at 1 A g−1 and long-term stability up to 1000 cycles. As illustrated in Figure (b), when two pieces of the mechanically damaged parts of the battery were rejoined together, abundant hydrogen bonds of hydroxyl groups could efficiently re-establish on the fracture interface and thus realised the self-healing behaviour. In addition, the electron transportation of the broken electrodes could also restore after self-healing process owing to the electrodes alignment.
Figure 9. (a) Schematic self-healing mechanism of the Zn(CF3SO3)2/PVA hydrogel electrolyte. (b) Schematic structure of the self-healing ZIBs. Reproduced with permission from reference [Citation44]. (c) Schematic illustration of the synthesis process of the self-healing PANa-Fe3+ hydrogel electrolyte. (d) Fabrication of the intrinsically self-healable NiCo/Zn battery with PANa-Fe3+ hydrogel electrolyte. (e) Self-healing demonstration of the NiCo/Zn battery. Reproduced with permission from reference [Citation19]. (f) Schematic illustration of the self-healing flexible ZIB with PVA–Zn/Mn hydrogel electrolyte. (g) Self-healing demonstration of PVA–Zn/Mn hydrogel electrolyte. (h) Photographs of the self-healing flexible ZIB during four times of cutting and self-healing. Reproduced with permission from reference [Citation101].
![Figure 9. (a) Schematic self-healing mechanism of the Zn(CF3SO3)2/PVA hydrogel electrolyte. (b) Schematic structure of the self-healing ZIBs. Reproduced with permission from reference [Citation44]. (c) Schematic illustration of the synthesis process of the self-healing PANa-Fe3+ hydrogel electrolyte. (d) Fabrication of the intrinsically self-healable NiCo/Zn battery with PANa-Fe3+ hydrogel electrolyte. (e) Self-healing demonstration of the NiCo/Zn battery. Reproduced with permission from reference [Citation19]. (f) Schematic illustration of the self-healing flexible ZIB with PVA–Zn/Mn hydrogel electrolyte. (g) Self-healing demonstration of PVA–Zn/Mn hydrogel electrolyte. (h) Photographs of the self-healing flexible ZIB during four times of cutting and self-healing. Reproduced with permission from reference [Citation101].](/cms/asset/4aa6b725-93b0-430b-94db-3c2f6bb75cc7/tmrl_a_2059412_f0009_oc.jpg)
In addition, a self-healing hydrogel electrolyte triggered by ferric ions-crosslinking has been developed, which is constructed with an integral dynamic network (Figure (c)) [Citation19]. In this work, the ferric ion crosslinkers formed abundant ionic bond among PANa chains in the polymer network to realise the self-healing ability, and the electrolyte ions were transported through the PANa-Fe3+ hydrogel matrix to support the electrochemical chemistry. Equipped with the as-synthesised PANa-Fe3+ hydrogel electrolyte, the resultant NiCo/Zn battery exhibited typical charge–discharge plateaus and exceptional autonomic self-healing ability that could retain 87% capacity after four cutting–healing cycles (Figure (d,e)). Recently, Liu et al. [Citation101] also proposed a self-healing flexible ZIB composing all-in-one electrodes of the Zn-nanowire/CC anode and VS2/CC cathode, accompanied with the PVA/Zn(CH3COO)2/Mn(CH3COO)2 hydrogel electrolyte (Figure (f)). This hydrogel electrolyte possessed abundant –OH group on PVA polymer chains, which could form strong hydrogen bonding to realise a self-healing function (Figure (g)). The resultant ZIB could retain over 88.9% potential after each cut/self-healing process (Figure (h)). In addition, substantial researchers have been devoted to develop intrinsic healable hydrogel electrolytes based on various self-healing mechanisms such as hydrogen bonding [Citation102], ester bonding [Citation103], conjugation [Citation104], and metal ionic bonding [Citation105]. In sum, the flexible self-healing Zn-based batteries exhibit great potential for the highly efficient wearable electronics with long-term usability.
5. Summary and perspective
In this review, recent advances of flexible Zn-based batteries using hydrogel electrolytes have been reviewed and discussed. In general, hydrogel electrolytes serve as both electrolyte and separator that supporting the ions transportation in a flexible battery without using the traditional porous separators. Therefore, the mechanical softness and the ionic conductivity of the hydrogel electrolytes are the essential characteristics that require attentive consideration in the design of flexible batteries. In addition to superior battery performance, advances in multifunctionality, such as super toughness, ultra-stretchability, extreme temperature tolerance, stimuli-responsive properties and self-healing capability, have been realised in flexible Zn-based batteries by the design of unique functional hydrogels through elaborate polymer chemistries, targeting on optimising mechanical durability, environmental adaptability and prolonged service life for practical applications. However, there are still some inadequacies that should be highlighted to raise more consideration in future research.
At subzero temperatures, the surface of hydrogel electrolyte will initially freeze before the hydrogel inner matrix, leading to a poor interfacial contact between the electrodes and the electrolyte [Citation106]. The interfacial resistance will immensely increase and thus deteriorate the battery performance. Apart from hydrogel electrolytes, low temperature will also decrease the performance of the electrodes to Zn-based batteries, especially in the ion diffusion rate and the electronic conductivity, which will further adversely affect the battery rate performance [Citation107]. Therefore, the electrochemical activity of the electrode materials is also crucial to flexible Zn-based batteries at low temperature, which should not be ignored.
Although introducing hydrated salt can be beneficial for anti-freezing resistance of hydrogel, the salts may precipitate at extremely low temperature. Similarly, water evaporation from hydrogel matrix can only be alleviated but cannot be avoided, as the loss of water will precipitate the salt, which adversely affects the ionic conductivity of hydrogel electrolyte and leads to the degradation of battery performance. The introduction of polyol solvents may also reduce the ionic conductivity. Moreover, too much modification on hydrogel network or polymer chains will make the manufacturing process more complex and raise costs. Therefore, more effective strategies for improving the extreme temperature tolerance of hydrogel electrolytes remain to be developed.
As flexible batteries are positioned as a potable power accessory for wearable electronics, which inevitably undergo frequent deformations in daily life, thus the long-term service life corresponding to both electrochemical and mechanical stabilities of the flexible batteries is of great significance. Unfortunately, the mechanical strength of most of the existing hydrogel electrolytes is still unsatisfactory to meet requirements in practical use. In addition, most reported self-healing hydrogel materials always take a long time to realise a well-repaired state after a fracture. New technologies for ultrafast self-healing capabilities should be further explored and assessed.
Due to the side reactions of water splitting that may occur in the discharging process, almost all aqueous Zn-based batteries only displayed open-circuit voltages from 1.4 to 1.6 V and a narrow voltage window below 2.0 V in mild aqueous electrolyte systems, which are far below than LIBs equipped with organic electrolytes. Therefore, it is of great significance to develop novel hydrogel electrolytes with wider electrochemical potential window so that more kinds of electrode materials can be sufficiently used with higher working voltage, with the hope to highly improve the energy and power densities of the flexible Zn-based batteries.
Besides the wearable applications, the scalability of the Zn-based batteries is also a big challenge. There are some persistent challenges that plaguing the scale-up implementation of the Zn-based batteries, especially located at the irreversible side reactions of Zn dendrites formation, HER, corrosion, and surface passivation, which always seriously affects the stability of Zn anode. The hydrogel electrolytes can effectively alleviate the HER in mild electrolyte systems benefited from its unique quasi-solid-state characteristics, but the effects for dendrite suppression of hydrogel electrolyte are normally unsatisfactory due to its relatively low mechanical strength.
Developing biocompatible hydrogel electrolytes is significantly beneficial to wearable applications. In practical use, direct contact of flexible and wearable batteries with the human skin is unavoidable; therefore, it is essential to ensure absolute safety of these batteries. Although the hydrogel electrolyte can effectively prevent the leakage of liquid electrolytes, the issues involving salt precipitation and corrosion should also be seriously concerned. Moreover, the use of heavy metal-based electrode materials like Co-containing catalysts, which are harmful to human body, require stringent restrictions in flexible batteries design.
Multifunctional hydrogel electrolytes are essential for broadening the application range of flexible Zn-based batteries in various conditions. The development of this prospective area requires further exploration on the combination of the fields of electrochemical and polymer chemistry, which highly desires a deep understanding of the scientific and technical views from interdisciplinary involving polymer synthetic chemistry, electrochemical mechanism, engineering mechanics and elaborate battery designs. Diverse multifunctionalities such as super toughness, extreme temperature tolerance, and self-healing ability have been developed and summarised in this article. However, there are still much potential functionalities (e.g. electrochromism, photodetection and shape memory) worthy for exploration and being integrated into the design of advanced battery systems, with a hope to pave the way for next-generation flexible and wearable battery technologies.
Acknowledgements
The authors would like to thank Dr Guojin Liang and Prof. Chunyi Zhi at City University of Hong Kong for helpful comments and assistance in manuscript preparation.
Disclosure statement
No potential conflict of interest was reported by the author(s).
Additional information
Funding
References
- Wang J, Li S, Yi F, et al. Sustainably powering wearable electronics solely by biomechanical energy. Nat Commun. 2016;7:1–8.
- Trung TQ, Ramasundaram S, Hwang BU, et al. An all-elastomeric transparent and stretchable temperature sensor for body-attachable wearable electronics. Adv Mater. 2016;28:502–509.
- Liu W, Song MS, Kong B, et al. Flexible and stretchable energy storage: recent advances and future perspectives. Adv Mater. 2017;29:1603436.
- Wang Q, Ping P, Zhao X, et al. Thermal runaway caused fire and explosion of lithium ion battery. J Power Sources. 2012;208:210–224.
- Chen Y, Kang Y, Zhao Y, et al. A review of lithium-ion battery safety concerns: the issues, strategies, and testing standards. J Energy Chem. 2021;59:83–99.
- Zhang H, Liu X, Li H, et al. Challenges and strategies for high-energy aqueous electrolyte rechargeable batteries. Angew Chem Int Ed. 2021;60:598–616.
- Wang F, Wu X, Li C, et al. Nanostructured positive electrode materials for post-lithium ion batteries. Energy Environ Sci. 2016;9:3570–3611.
- Xu Y, Xu C, An Q, et al. Robust LiTi2(PO4)3 microflowers as high-rate and long-life cathodes for Mg-based hybrid-ion batteries. J Mater Chem A. 2017;5:13950–13956.
- Li H, Ma L, Han C, et al. Advanced rechargeable zinc-based batteries: recent progress and future perspectives. Nano Energy. 2019;62:550–587.
- Fang G, Zhou J, Pan A, et al. Recent advances in aqueous zinc-ion batteries. ACS Energy Lett. 2018;3:2480–2501.
- Wang C, Pei Z, Meng Q, et al. Toward flexible zinc-ion hybrid capacitors with superhigh energy density and ultralong cycling life: the pivotal role of ZnCl2 salt-based electrolytes. Angew Chem Int Ed. 2021;60:990–997.
- Yang Q, Li Q, Liu Z, et al. Dendrites in Zn-based batteries. Adv Mater. 2020;32:2001854.
- Yi Z, Chen G, Hou F, et al. Strategies for the stabilization of Zn metal anodes for Zn-ion batteries. Adv Energy Mater. 2021;11:2003065.
- Zampardi G, La Mantia F. Prussian blue analogues as aqueous Zn-ion batteries electrodes: current challenges and future perspectives. Curr Opin Electroche. 2020;21:84–92.
- Guo X, Zhou J, Bai C, et al. Zn/MnO2 battery chemistry with dissolution-deposition mechanism. Mater Today Energy. 2020;16:100396.
- Selvakumaran D, Pan A, Liang S, et al. A review on recent developments and challenges of cathode materials for rechargeable aqueous Zn-ion batteries. J Mater Chem A. 2019;7:18209–18236.
- Wang Z, Li H, Tang Z, et al. Hydrogel electrolytes for flexible aqueous energy storage devices. Adv Funct Mater. 2018;28:1804560.
- Liu F, Urban MW. Recent advances and challenges in designing stimuli-responsive polymers. Prog Polym Sci. 2010;35:3–23.
- Huang Y, Liu J, Wang J, et al. An intrinsically self-healing NiCo||Zn rechargeable battery with a self-healable ferric-ion-crosslinking sodium polyacrylate hydrogel electrolyte. Angew Chem Int Ed. 2018;57:9810–9813.
- Wang H, Liu J, Wang J, et al. Concentrated hydrogel electrolyte-enabled aqueous rechargeable NiCo||Zn battery working from 20 to 50 °C. ACS Appl Mat Interf. 2018;11:49–55.
- Chen R, Xu X, Peng S, et al. A flexible and safe aqueous zinc–air battery with a wide operating temperature range from −20 to 70 °C. ACS Sustain Chem Eng. 2020;8:11501–11511.
- Ma L, Chen S, Li X, et al. Liquid-free all-solid-state zinc batteries and encapsulation-free flexible batteries enabled by in situ constructed polymer electrolyte. Angew Chem Int Ed. 2020;132:24044–24052.
- Huang Y, Li Z, Pei Z, et al. Solid-state rechargeable Zn//NiCo and Zn–air batteries with ultralong lifetime and high capacity: The role of a sodium polyacrylate hydrogel electrolyte. Adv Energy Mater. 2018;8:1802288.
- Liu Z, Liang G, Zhan Y, et al. A soft yet device-level dynamically super-tough supercapacitor enabled by an energy-dissipative dual-crosslinked hydrogel electrolyte. Nano Energy. 2019;58:732–742.
- Sun J-Y, Zhao X, Illeperuma WR, et al. Highly stretchable and tough hydrogels. Nature. 2012;489:133–136.
- Wang J, Huang Y, Liu B, et al. Flexible and anti-freezing zinc-ion batteries using a guar-gum/sodium-alginate/ethylene-glycol hydrogel electrolyte. Energy Storage Mater. 2021;41:599–605.
- Han L, Liu K, Wang M, et al. Mussel-inspired adhesive and conductive hydrogel with long-lasting moisture and extreme temperature tolerance. Adv Funct Mater. 2018;28:1704195.
- Taylor DL, Panhuis IHM. Self-healing hydrogels. Adv Mater. 2016;28:9060–9093.
- Peng H, Lv Y, Wei G, et al. A flexible and self-healing hydrogel electrolyte for smart supercapacitor. J Power Sources. 2019;431:210–219.
- Guo Y, Zheng K, Wan P. A flexible stretchable hydrogel electrolyte for healable all-in-one configured supercapacitors. Small. 2018;14:1704497.
- Song Z, Ding J, Liu B, et al. A rechargeable Zn–air battery with high energy efficiency and long life enabled by a highly water-retentive gel electrolyte with reaction modifier. Adv Mater. 2020;32:1908127.
- Fan X, Liu J, Song Z, et al. Porous nanocomposite gel polymer electrolyte with high ionic conductivity and superior electrolyte retention capability for long-cycle-life flexible zinc–air batteries. Nano Energy. 2019;56:454–462.
- Choudhury N, Sampath S, Shukla A. Hydrogel-polymer electrolytes for electrochemical capacitors: an overview. Energy Environ Sci. 2009;2:55–67.
- Zhao F, Bae J, Zhou X, et al. Nanostructured functional hydrogels as an emerging platform for advanced energy technologies. Adv Mater. 2018;30:1801796.
- Gong JP. Materials both tough and soft. Science. 2014;344:161–162.
- Kwon HJ, Osada Y, Gong JP. Polyelectrolyte gels-fundamentals and applications. Polym J. 2006;38:1211–1219.
- Wu K, Huang J, Yi J, et al. Recent advances in polymer electrolytes for zinc ion batteries: mechanisms, properties, and perspectives. Adv Energy Mater. 2020;10:1903977.
- Li P, Jin Z, Peng L, et al. Stretchable all-gel-state fiber-shaped supercapacitors enabled by macromolecularly interconnected 3D graphene/nanostructured conductive polymer hydrogels. Adv Mater. 2018;30:1800124.
- Wang K, Zhang X, Han J, et al. High-performance cable-type flexible rechargeable Zn battery based on MnO2@CNT fiber microelectrode. ACS Appl Mat Interf. 2018;10:24573–24582.
- Wang K, Zhang X, Li C, et al. Chemically crosslinked hydrogel film leads to integrated flexible supercapacitors with superior performance. Adv Mater. 2015;27:7451–7457.
- Jin X, Sun G, Zhang G, et al. A cross-linked polyacrylamide electrolyte with high ionic conductivity for compressible supercapacitors with wide temperature tolerance. Nano Res. 2019;12:1199–1206.
- Huang Y, Zhong M, Shi F, et al. An intrinsically stretchable and compressible supercapacitor containing a polyacrylamide hydrogel electrolyte. Angew Chem Int Ed. 2017;56:9141–9145.
- Liu J, Guan C, Zhou C, et al. A flexible quasi-solid-state nickel–zinc battery with high energy and power densities based on 3D electrode design. Adv Mater. 2016;28:8732–8739.
- Huang S, Wan F, Bi S, et al. A self-healing integrated all-in-one zinc-ion battery. Angew Chem. 2019;131:4357–4361.
- Karan S, Sahu TB, Sahu M, et al. Characterization of ion transport property in hot-press cast solid polymer electrolyte (SPE) films: [PEO: Zn(CF3SO3)2]. Ionics. 2017;23:2721–2726.
- Li H, Liu Z, Liang G, et al. Waterproof and tailorable elastic rechargeable yarn zinc ion batteries by a cross-linked polyacrylamide electrolyte. ACS Nano. 2018;12:3140–3148.
- Ma L, Chen S, Li H, et al. Initiating a mild aqueous electrolyte Co3O4/Zn battery with 2.2 V-high voltage and 5000-cycle lifespan by a Co(III) rich-electrode. Energy Environ Sci. 2018;11:2521–2530.
- Zhu X, Yang H, Cao Y, et al. Preparation and electrochemical characterization of the alkaline polymer gel electrolyte polymerized from acrylic acid and KOH solution. Electrochim Acta. 2004;49:2533–2539.
- Liu J, Hu M, Wang J, et al. An intrinsically 400% stretchable and 50% compressible NiCo//Zn battery. Nano Energy. 2019;58:338–346.
- Han Q, Chi X, Zhang S, et al. Durable, flexible self-standing hydrogel electrolytes enabling high-safety rechargeable solid-state zinc metal batteries. J Mater Chem A. 2018;6:23046–23054.
- Wang Z, Ruan Z, Liu Z, et al. A flexible rechargeable zinc-ion wire-shaped battery with shape memory function. J Mater Chem A. 2018;6:8549–8557.
- Zhang S, Yu N, Zeng S, et al. An adaptive and stable bio-electrolyte for rechargeable Zn-ion batteries. J Mater Chem A. 2018;6:12237–12243.
- Shi Y, Zhang J, Pan L, et al. Energy gels: a bio-inspired material platform for advanced energy applications. Nano Today. 2016;11:738–762.
- Zhao F, Shi Y, Pan L, et al. Multifunctional nanostructured conductive polymer gels: synthesis, properties, and applications. Acc Chem Res. 2017;50:1734–1743.
- Rong Q, Lei W, Chen L, et al. Anti-freezing, conductive self-healing organohydrogels with stable strain-sensitivity at subzero temperatures. Angew Chem Int Ed. 2017;56:14159–14163.
- Liu Q, Nian G, Yang C, et al. Bonding dissimilar polymer networks in various manufacturing processes. Nat Commun. 2018;9:1–11.
- Yang H, Liu Z, Chandran BK, et al. Self-protection of electrochemical storage devices via a thermal reversible sol–gel transition. Adv Mater. 2015;27:5593–5598.
- Liu H, Xiong C, Tao Z, et al. Zwitterionic copolymer-based and hydrogen bonding-strengthened self-healing hydrogel. RSC Adv. 2015;5:33083–33088.
- Kakuta T, Takashima Y, Nakahata M, et al. Preorganized hydrogel: self-healing properties of supramolecular hydrogels formed by polymerization of host–guest- monomers that contain cyclodextrins and hydrophobic guest groups. Adv Mater. 2013;25:2849–2853.
- Nakahata M, Takashima Y, Yamaguchi H, et al. Redox-responsive self-healing materials formed from host–guest polymers. Nat Commun. 2011;2:1–6.
- Hu M, Wang J, Liu J, et al. A flour-based one-stop supercapacitor with intrinsic self-healability and stretchability after self-healing and biodegradability. Energy Storage Mater. 2019;21:174–179.
- Luo F, Sun TL, Nakajima T, et al. Oppositely charged polyelectrolytes form tough, self-healing, and rebuildable hydrogels. Adv Mater. 2015;27:2722–2727.
- Wei Z, He J, Liang T, et al. Autonomous self-healing of poly (acrylic acid) hydrogels induced by the migration of ferric ions. Polym Chem. 2013;4:4601–4605.
- Huang Y, Zhong M, Huang Y, et al. A self-healable and highly stretchable supercapacitor based on a dual crosslinked polyelectrolyte. Nat Commun. 2015;6:1–8.
- Huang S, Zhu J, Tian J, et al. Recent progress in the electrolytes of aqueous zinc-ion batteries. Chem Eur J. 2019;25:14480–14494.
- Shi Y, Chen Y, Shi L, et al. An overview and future perspectives of rechargeable zinc batteries. Small. 2020;16:2000730.
- Naveed A, Yang H, Yang J, et al. Highly reversible and rechargeable safe Zn batteries based on a triethyl phosphate electrolyte. Angew Chem Int Ed. 2019;58:2760–2764.
- Naveed A, Yang H, Shao Y, et al. A highly reversible Zn anode with intrinsically safe organic electrolyte for long-cycle-life batteries. Adv Mater. 2019;31:1900668.
- Yi J, Guo S, He P, et al. Status and prospects of polymer electrolytes for solid-state Li–O2 (air) batteries. Energy Environ Sci. 2017;10:860–884.
- Xu C, Li B, Du H, et al. Energetic zinc ion chemistry: the rechargeable zinc ion battery. Angew Chem Int Ed. 2012;124:957–959.
- Zhang N, Cheng F, Liu J, et al. Rechargeable aqueous zinc-manganese dioxide batteries with high energy and power densities. Nat Commun. 2017;8:1–9.
- Wan F, Niu Z. Design strategies for vanadium-based aqueous zinc-ion batteries. Angew Chem Int Ed. 2019;131:16508–16517.
- Kundu D, Vajargah SH, Wan L, et al. Aqueous vs. nonaqueous Zn-ion batteries: consequences of the desolvation penalty at the interface. Energy Environ Sci. 2018;11:881–892.
- Zhang L, Chen L, Zhou X, et al. Towards high-voltage aqueous metal-ion batteries beyond 1.5V: the zinc/zinc hexacyanoferrate system. Adv Energy Mater. 2015;5:1400930.
- Shi B, Li Z, Fan Y. Implantable energy-harvesting devices. Adv Mater. 2018;30:1801511.
- Liu Y, Pharr M, Salvatore GA. Lab-on-skin: a review of flexible and stretchable electronics for wearable health monitoring. ACS Nano. 2017;11:9614–9635.
- Duan J, Xie W, Yang P, et al. Tough hydrogel diodes with tunable interfacial adhesion for safe and durable wearable batteries. Nano Energy. 2018;48:569–574.
- Fang G, Zhu C, Chen M, et al. Suppressing manganese dissolution in potassium manganate with rich oxygen defects engaged high-energy-density and durable aqueous zinc-ion battery. Adv Funct Mater. 2019;29:1808375.
- Zeng Y, Zhang X, Meng Y, et al. Achieving ultrahigh energy density and long durability in a flexible rechargeable quasi-solid-state Zn–MnO2 battery. Adv Mater. 2017;29:1700274.
- Qiu W, Li Y, You A, et al. High-performance flexible quasi-solid-state Zn–MnO2 battery based on MnO2 nanorod arrays coated 3D porous nitrogen-doped carbon cloth. J Mater Chem A. 2017;5:14838–14846.
- Li H, Han C, Huang Y, et al. An extremely safe and wearable solid-state zinc ion battery based on a hierarchical structured polymer electrolyte. Energy Environ Sci. 2018;11:941–951.
- Chen X, Zhong C, Liu B, et al. Atomic layer Co3O4 nanosheets: the key to knittable Zn–air batteries. Small. 2018;14:1702987.
- Li Y, Zhong C, Liu J, et al. Atomically thin mesoporous Co3O4 layers strongly coupled with n-rGO nanosheets as high-performance bifunctional catalysts for 1D knittable zinc–air batteries. Adv Mater. 2018;30:1703657.
- Huang Y, Ip WS, Lau YY, et al. Weavable, conductive yarn-based NiCo//Zn textile battery with high energy density and rate capability. ACS Nano. 2017;11:8953–8961.
- Zamarayeva AM, Gaikwad AM, Deckman I, et al. Fabrication of a high-performance flexible silver–zinc wire battery. Adv Electron Mater. 2016;2:1500296.
- Zeng S, Tong X, Zhou S, et al. All-in-one bifunctional oxygen electrode films for flexible Zn-air batteries. Small. 2018;14:1803409.
- Li L, Lou Z, Chen D, et al. Recent advances in flexible/stretchable supercapacitors for wearable electronics. Small. 2018;14:1702829.
- Liu Z, Wang D, Tang Z, et al. A mechanically durable and device-level tough Zn-MnO2 battery with high flexibility. Energy Storage Mater. 2019;23:636–645.
- Pei Z, Ding L, Wang C, et al. Make it stereoscopic: interfacial design for full-temperature adaptive flexible zinc-air batteries. Energy Environ Sci. 2021;14:4926–4935.
- Pei Z, Yuan Z, Wang C, et al. A flexible rechargeable zinc–air battery with excellent low-temperature adaptability. Angew Chem Int Ed. 2020;132:4823–4829.
- Liu Y, Zhou X, Bai Y, et al. Engineering integrated structure for high-performance flexible zinc-ion batteries. Chem Eng J. 2021;417:127955.
- Chen M, Zhou W, Wang A, et al. Anti-freezing flexible aqueous Zn-MnO2 batteries working at −35°C enabled by a borax-crosslinked polyvinyl alcohol/glycerol gel electrolyte. J Mater Chem A. 2020;8:6828–6841.
- Mo F, Liang G, Meng Q, et al. A flexible rechargeable aqueous zinc manganese-dioxide battery working at −20 °C. Energy Environ Sci. 2019;12:706–715.
- Chen M, Chen J, Zhou W, et al. Realizing an all-round hydrogel electrolyte toward environmentally adaptive dendrite-free aqueous Zn–MnO2 batteries. Adv Mater. 2021;33:2007559.
- Adebahr J, Byrne N, Forsyth M, et al. Enhancement of ion dynamics in PMMA-based gels with addition of TiO2 nano-particles. Electrochim Acta. 2003;48:2099–2103.
- Yang C-C. Study of alkaline nanocomposite polymer electrolytes based on PVA–ZrO2–KOH. Mater Sci Eng B-Adv. 2006;131:256–262.
- Mo F, Liang G, Wang D, et al. Biomimetic organohydrogel electrolytes for high-environmental adaptive energy storage devices. EcoMat. 2019;1:e12008.
- Zhao J, Sonigara KK, Li J, et al. A smart flexible zinc battery with cooling recovery ability. Angew Chem Int Ed. 2017;129:7979–7983.
- Mo F, Li H, Pei Z, et al. A smart safe rechargeable zinc ion battery based on sol-gel transition electrolytes. Sci Bull. 2018;63:1077–1086.
- Yang P, Feng C, Liu Y, et al. Thermal self-protection of zinc-ion batteries enabled by smart hygroscopic hydrogel electrolytes. Adv Energy Mater. 2020;10:2002898.
- Liu J, Long J, Shen Z, et al. A self-healing flexible quasi-solid zinc-ion battery using all-in-one electrodes. Adv Sci. 2021;8:2004689.
- Wang S, Liu N, Su J, et al. Highly stretchable and self-healable supercapacitor with reduced graphene oxide based fiber springs. ACS Nano. 2017;11:2066–2074.
- Wang Z, Tao F, Pan Q. A self-healable polyvinyl alcohol-based hydrogel electrolyte for smart electrochemical capacitors. J Mater Chem A. 2016;4:17732–17739.
- Tao F, Qin L, Wang Z, et al. Self-healable and cold-resistant supercapacitor based on a multifunctional hydrogel electrolyte. ACS Appl Mat Interfaces. 2017;9:15541–15548.
- Guo Y, Zhou X, Tang Q, et al. A self-healable and easily recyclable supramolecular hydrogel electrolyte for flexible supercapacitors. J Mater Chem A. 2016;4:8769–8776.
- He Z, Wu C, Hua M, et al. Bioinspired multifunctional anti-icing hydrogel. Matter. 2020;2:723–734.
- An L, Huang B, Zhang Y, et al. Interfacial defect engineering for improved portable zinc–air batteries with a broad working temperature. Angew Chem Int Ed. 2019;131:9559–9563.