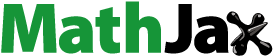
Abstract
Through composition design and structural regulation, low electronegativity selenium and phosphorus were doped into NiCo LDH nanosheets to prepare phosphorus and selenium co-doped nickel–cobalt layered double hydroxide electrodes (P, Se-NiCo LDH), which can enhance the availability of active sites, significantly improve the conductivity and promote the reaction kinetics. Prepared it as a positive cathode of supercapacitor ultrahigh energy density (61.23 Wh kg−1) and power density (16496.5 W kg−1) can be obtained. Rate performance of the hybrid supercapacitor can be maintained 50.9% at 20 A g−1. Both excellent rate performance and energy density may promote the wider application of supercapacitors.
GRAPHICAL ABSTRACT
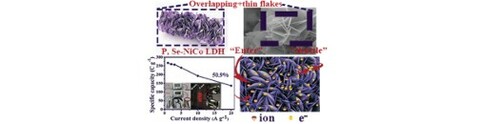
IMPACT STATEMENT
P and Se have influence on the morphology and pore size of NiCo LDH, which make P, Se-NiCo LDH have more active sites, and thus promoting the reaction kinetics.
KEYWORDS:
1. Introduction
Supercapacitors (SCs) have attracted much attention due to the ultra-high power density and faster charging and discharging capability [Citation1–4]. However, the energy density of SCs cannot meet the application in actual equipment [Citation5–8]. In order to break through this bottleneck, a lot of exploration and research have been carried out. Layered double hydroxide has a unique sandwich structure, which can make it have excellent supercapacitor performance by adjusting metal cations and exchanging interlayer anions [Citation9–11]. In addition, LDH materials have battery-like characteristics [Citation12]. Therefore, it has a higher charge storage capacity. In particular, nickel–cobalt layered double hydroxide (NiCo LDH) has received widespread attention on account of its high theoretical ability and excellent redox activity [Citation13–15]. Such as NiCo-LDH hollow spheres [Citation16], PNT@NiCo-LDH [Citation17], CNT/Co3S4@NiCo LDH [Citation18] and 3D flower-like hierarchical NiCo-LDH microspheres [Citation19]. However, the adjustment of the structure cannot meet the requirements of the conductivity and cycle stability of the supercapacitor. Rao et al designed and fabricated a phosphorus-doped 3D porous graphene electrode. It can achieve a high energy density of 6.24 μWh cm−2 and maintain more than 85% cycle stability after 10,000 cycles [Citation20]. Ramu Manikandan et al. prepared selenium-rich hybrid NiSe2@Fe3Se4 (NFS) nanocomposites on nickel foam. It shows a superior energy density of 52 W h kg−1 at 398 W kg−1 and the capacitance retention about 92% after 10,000 cycles at 5 A g−1 [Citation21]. Therefore, doping with heteroatoms such as N, P and Se can have synergistic effects with other elements and change their electronic structures, thus providing more electrochemical active sites and greater reaction kinetics [Citation22]. The electronegativity of phosphorus is lower than oxygen and selenium, so it can improve the conductivity, but pure transition metal phosphide has poor rate performance and cycle life, and selenization can just make up for these defects. Therefore, the co-doping of phosphorus and selenium can effectively improve the electrochemical property of NiCo LDH [Citation23].
Here, we use a simple hydrothermal method to in-situ grow P, Se-NiCo LDH with an overlapping sheet-like structure on the nickel foam. This composite material as a positive electrode, and the energy density of the hybrid supercapacitor P, Se-NiCo LDH//AC (active carbon) reaches 61.23 W h kg−1 and the power density of 825 W kg−1, even achieves a high power density of 16496.5 W kg−1 and energy density still can reach 31.16 W h kg−1. The specific capacitance can remain over 50.9% at 20 A/g−1, and it still has an excellent capacity retention rate of 92.1% at 5 A g−1 after 10,000 cycles. Our work successfully demonstrated the tremendous improvement of the electrochemical performance of NiCo LDH by co-doped selenium and phosphorus, which may the unlimited potential of this composite material in the future research of supercapacitors.
2. Results and discussion
Figure shows a schematic illustration of the formation process of P, Se-NiCo LDH, the rapid transport of ions and electrons as well as rapid kinetics for charge separation. Detailed laboratory sections are listed in supporting information. The final structure of P, Se-NiCo LDH is composed of ultra-thin nanosheets overlapping each other. In the meantime, the ultra-thin nanosheets facilitate electron migration, and the stacked channels by nanosheets help to transport the electrolyte. Therefore, P, Se-NiCo LDH may be a potential high-performance electrode of supercapacitor.
Figure 1. Preparation process of P, Se-NiCo LDH and the rapid transfer of electrons and ions as well as rapid kinetics for charge separation.
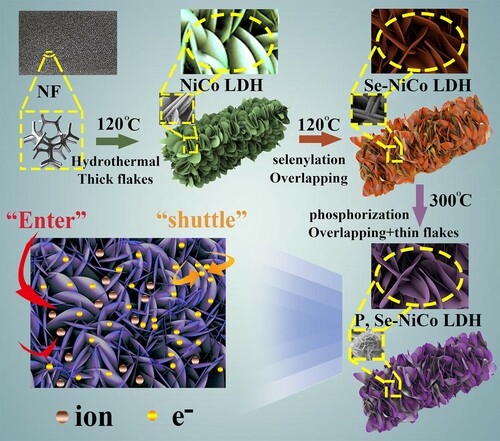
The chemical composition and phase analysis were deeply explored by XRD, as shown in Figure (a). The peaks of NiCo LDH are consistent with those of the standard PDF card β-Ni(OH)2 (No. 74-2057) and Co(OH)2 (No. 30-0443), which are expressed as NiCo LDH [Citation24,Citation25]. Furthermore, the peaks of the intermediate product Se-NiCo LDH are consistent with NiCoSe2 (JCPDS No.70-2851) [Citation26,Citation27]. The diffraction peaks of NiCo LDH are still maintained at the NiCoSe2, proving the successful synthesis of Se-NiCo LDH. The XRD peaks of P, Se-NiCo LDH are consistent with NiCoP (JCPDS No.71-2336) [Citation28], which can prove the successful preparation of P, Se-NiCo LDH [Citation29,Citation30]. XPS tests investigate the chemical state and composition of P, Se-NiCo LDH, in Figure (b), the image corresponds to the peaks of P 2p3/2 and 2p1/2 at 132.7 and 137.3 eV [Citation31]. In Figure (c), the peaks of binding energies at 53.2, 54.9 and 58.1 eV correspond to 3d5/2, 3d3/2 and SeOx of selenium [Citation32]. The characteristic peaks of O 1s which centered at 531.5, 531.2 and 530.8 in Figure (d) are assigned to surface adsorbed H2O, –OH and O–M [Citation33]. The existence of these characteristic peaks fully confirms the formation of Ni-Co hydroxide. Therefore, XPS results confirmed that P, Se-NiCo LDH was synthesized.
Figure 2. (a) XRD patterns of NiCo LDH, Se-NiCo LDH and P, Se-NiCo LDH; High-resolution XPS of (b) P 2p; (c) Se 3d and (d) O 1s of P, Se-NiCo LDH. (e) N2 adsorption–desorption isotherms and (f) BJH pore size distribution curve of NiCo LDH, Se-NiCo LDH, P-NiCo LDH and P, Se-NiCo LDH composites.
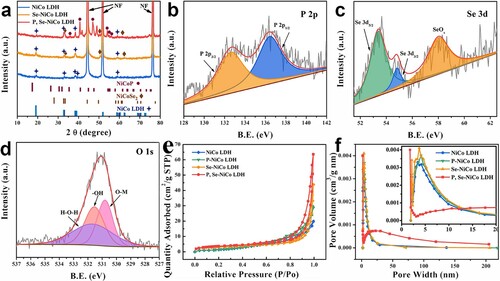
Barret–Joyner–Halenda (BJH) pore size distribution and Brunauer–Emmett–Teller (BET) specific surface area of NiCo LDH, P-NiCo LDH, Se-NiCo LDH and P, Se-NiCo LDH were conducted. In Figure (e), the BET specific surface area of P, Se-NiCo LDH is 12.75 m2 g−1, and the adsorption belongs to type III isotherm. Figure (f) shows the pore size distribution diagram of NiCo LDH, P-NiCo LDH, Se-NiCo LDH and P, Se-NiCo LDH. The P, Se-NiCo LDH has a wider pore size distribution, which owns mesopore and macropore. A large amount of mesopore and high specific surface area are conducive to providing more electrochemical active sites and increasing electron/ion diffusion rate, which could improve the electrochemical performances of P, Se-NiCo LDH.
Observed the internal structure of the materials through SEM images. Figure (a) shows the SEM image of NiCo LDH with sheet-like structure, which has an average thickness of 30 nm. By phosphating (Figure (b)), the thickness of P-NiCo LDH is about 10 nm, but the sheets have obvious superposition. After selenizing, the thickness of sheet-like Se-NiCo LDH is around 30 nm (Figure (c)), while a small number of pieces have been overlapped. The SEM image of P, Se-NiCo LDH displays that the sheets become thinner, and one piece overlaps with another. Low-magnification SEM images of samples are shown in Figures S2–S5. TEM was used to analyze the further microscopic morphology information of the final product P, Se-NiCo LDH, as shown in Figure (e). The HRTEM image of Figure (f) shows that the interplanar spacings of 2.7 and 3.1 Å match the (101) and (110) lattice planes of Se-NiCo LDH. In addition, the lattice fringe with a crystal interplanar spacing of 2.2 Å matches the (111) plane of P-NiCo LDH, indicating the successful doping of selenium and phosphorus elements. The element mapping diagrams in Figure (g) show that Ni, Co, Se, P and O are uniformly dispersing in the material.
Figure 3. (a–d) SEM images of the NiCo LDH, P-NiCo LDH, Se-NiCo LDH and P, Se-NiCo LDH. (e) TEM observation of P, Se-NiCo LDH sample. (f) HRTEM images of P, Se-NiCo LDH sample. (g) EDS elemental mapping of P, Se-NiCo LDH composite.
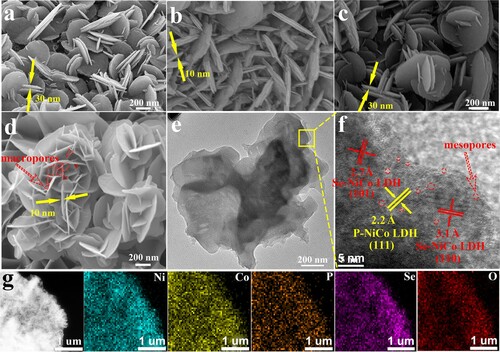
Due to the wider pore size distribution of P, Se-NiCo LDH electrode material, it is expected to show excellent electrochemical performances. Herein, NiCo LDH, P-NiCo LDH and Se-NiCo LDH were used as control groups of P, Se-NiCo LDH. A three-electrode configuration and 3 mol L−1 of KOH electrolyte were adopted (the potential window is –0.2–0.8 V). Figure (a) is the CV curves of four electrodes, there are obvious REDOX peaks in all samples, indicating that the energy storage is largely related to the reduction and oxidation reaction, and it is a capacitive type. Meanwhile, the integrated area of the cyclic voltammetry curve of the P, Se-NiCo LDH is significantly greater at the same sweep rate, indicating that its capacity is the largest under the same conditions. Figure (b) analyzes GCD curves at 1 Ag−1, which clearly shows that P, Se-NiCo LDH has the longest charge/discharge time among the four groups, meaning that it has the highest specific capacitance. Subsequently, the Nyquist curves and fitting circuit diagram of each sample are shown in Figure (c), firstly, the P, Se-NiCo LDH has the smallest intercept, proving that the interface resistance (Rs) between electrode and electrolyte is the smallest, because it has both the mesopore and macropore. In the high frequency region, the smallest semicircle of P, Se-NiCo LDH represents the lowest charge transfer resistance (Rct). The lowest Rct value displays that P, Se-NiCo LDH has the fastest electron transfer rate and excellent ion permeability [Citation34]. The slope of the low frequency region of the P, Se-NiCo LDH is also greater than those of NiCo LDH, P-NiCo LDH and Se-NiCo LDH, indicating that it has the smallest Warburg impedance. In general, the co-doping of phosphorus and selenium greatly decreases the impedance of NiCo LDH, thus the electrochemical performances were greatly improved. Figure S7(a,b) and Figure S9(a–f) are the CV curves (5–50 mV s−1) and galvanostatic charge–discharge (GCD) curves (0–0.4 V) of the P, Se-NiCo LDH, NiCo LDH, Se-NiCo LDH and P-NiCo LDH electrodes. By contrast, when the selenium and phosphorus were co-doped, the capacity can be increased and the polarization can be decreased. The GCD graph shows a hump/shoulder voltage platform, which is consistent with the pseudo-capacitance charge storage mechanism mentioned in CV analysis [Citation35,Citation36]. Their rate performances are shown in Figure S8, and the specific capacities are is listed in Table S1. Compared to just phosphating or selenization samples, the rate performance of P, Se-NiCo LDH has been greatly improved, and the capacity of P, Se-NiCo LDH is also significantly larger than those of other samples, proving the ultrathin nanosheet of 10 nm and lapped structure facilitate transfer of ions and electrons and diffusion of electrolyte.
Figure 4. (a) CV curves, (b) GCD profiles, (c) Nyquist plots and (d) b-values of four electrode materials. (e) Capacitance contribution and (f) Voltammetric response of P, Se-NiCo LDH electrode. (g) Cycle stability of four electrode materials at 10 A g−1.
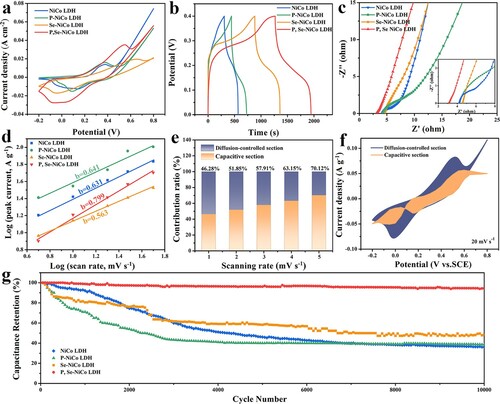
Furthermore, b-values of four cathodes are shown in Figure (d), which is an important indicator of the charge storage mechanism. The b curve can be obtained by fitting log(iP) and log(v) according to the following formula [Citation37,Citation38]:
(1)
(1) where I (mA cm−2) is the anode and cathode peak current, a and b are adjustable constants (b = 0.5 means that the charge storage is completely controlled by the battery-type energy storage diffusion, b = 1.0 means that the charge storage is only controlled by the surface), V (mV s−1) is the scanning rate. The b value of P, Se-NiCo LDH obtained by fitting and calculation is 0.799, between 0.5 and 1.0, indicating that capacitance control and diffusion control work together in the charging and discharging process [Citation39]. According to the following formula, the specific contributions of diffusion capacity (k2ν1/2) and surface capacity (k1ν) are quantitatively analyzed, and the current value under a specific potential (iv) is calculated [Citation40,Citation41]:
(2)
(2) Among them, i(V) is the peak current (A), k1v is the capacitance capacity contribution, and k2v1/2 is the diffusion capacity behavior. Figure (e) shows the capacitance contribution under various scan rates. Figure (f) shows the capacitance contribution at 20 mV s−1. The contribution of capacitance control occupies 46.28%, 51.85%, 57.91%, 63.15% and 70.12% in the whole process, respectively. Therefore, the efficient charge storage capability of P, Se-NiCo LDH is realized through the synergistic effect of capacitance control and diffusion control. In Figure S11, the Cdl values of NiCo LDH, P-NiCo LDH, Se-NiCo LDH and P, Se-NiCo LDH are 1.76, 1.57, 1.25 and 1.94 mF cm−2 by fitting and quantifying the electrochemically active area. P, Se-NiCo LDH has the largest Cdl value, showing that active sites are more exposed, so electrochemical performance can be significantly improved by morphology and pore regulation. Moreover, The cyclic stability of the four electrodes under 10 A g−1 was compared in Figure (g). The results showed that the P, Se-NiCo LDH cathode has the best cycle stability up to 94.46%, because its lapping structure of ultrathin nanosheets is the most stable structure.
Due to the best electrochemical performances of P, Se-NiCo LDH, We use it to assemble P, Se-NiCo LDH//AC hybrid supercapacitor, which can operate well under 0–1.65 V. Figure (b) shows the CV curves of P, Se-NiCo LDH//AC supercapacitor at 5–50 mV s−1. As the scan rate increases, the shape of the curve does not change significantly, displaying that P, Se-NiCo LDH//AC has an excellent capacitance behavior. Figure (c) is the GCD curves before and after 10000th cycles. Inset of Figure (d) is the GCD curves of P, Se-NiCo LDH//AC hybrid supercapacitor at 1–20 A g−1. With the increase of current density, all the curve shapes show good symmetry. Hence, P, Se-NiCo LDH//AC supercapacitor has excellent reversibility and good coulomb efficiency. Meanwhile, the calculated specific capacities are plotted in Figure (d). Its specific capacity at 1 A g−1 is 267.2 C g−1, and even at 20 A g−1, it also has a specific capacity of 136 C g−1, that is to say, the device can maintain 50.9% specific capacity rate, showing a good rate performance. The EIS curve of P, Se-NiCo LDH//AC after 10,000 cycles and before starting is shown in Figure (e). The Rct value only increases slightly after cycle. Therefore, the supercapacitor device still keeps fast electron transfer rate and ion permeability even after 10,000 cycles. Figure S12(a,b) indicates that the high-efficiency charge storage capability of P, Se-NiCo LDH is realized through both the capacitance control and diffusion control. In addition, the Ragone diagrams of comparison for P, Se-NiCo LDH//AC hybrid supercapacitor with other related NiCo LDH hybrid capacitors are as shown in Figure (f). According to the Ragone diagram, the electrochemical performances of P, Se-NiCo LDH//AC is superior to most other similar devices, such as CNT/Co3S4@NiCo LDH//AC [Citation18], NiCo LDH/IPC//IPC [Citation42], NiCo-LDH/rGO//AC [Citation43], NiCo-LDH//AC [Citation19], Co(OH)F@NiCo-LDH//rGO [Citation44], 4M-P@NiCo LDH//AC [Citation45], C-LDH/Co9S8//AC [Citation46], Ni1Co1-LDH@ACF//AC[Citation47], CoSx@NiCo-LDH//AC [Citation48] and CC/NiCoP@NiCo-LDH//AC [Citation49]. Table S2 shows in detail the electrochemical performances of P, Se-NiCo LDH//AC with other previously reported NiCo LDH devices. The cycle test of P, Se-NiCo LDH//AC is carried out (Figure (g)). At current density of 5 A g−1, the capacitance retention rate after 10,000 cycles is 92.1%, and the Coulombic efficiency is close to 100%. We also tested the practical application of P, Se-NiCo LDH//AC assembled devices. As shown in the inset of Figure (g), the two series-wound P, Se-NiCo LDH//AC devices can light up fifteen red bulbs over forty minutes. And it can power a digital meter for more than an hour. The SEM image of the P, Se-NiCo LDH electrode after 10,000 cycles is shown in the inset of Figure (g). The sheet structure remains good after the cycle, the macroporous structure formed by the overlap of the sheets still remains good and becomes thicker, so the device has excellent cycle stability. The P, Se-NiCo LDH cathode is composed of lapped ultrathin nanosheet with mesoporous and macroporous structures make the supercapacitor device with fairly high energy density, ultra-long cycle stability and excellent rate performance. The P, Se-NiCo LDH//AC device is with excellent comprehensive electrochemical performances, which make it with a broad application prospect.
Figure 5. (a) CV curves of P, Se-NiCo LDH and AC electrodes during 10 mV s−1. P, Se-NiCo LDH//AC device of (b) CV curves at 5–50 mV s−1, (c) GCD curves before and after 10,000th cycles, (d) Specific capacitances at 1–20 A g−1 (inset: GCD curves at 1–20 A g−1), (e) Nyquist plots before and after cycle, (f) Ragone plot for comparison, and (g) Long cycle stability test at 5 A g−1. Insets of (g) are the photographs of devices connected in series to trigger electronic meter and 15 red LEDs, the inset SEM image of (g) is P, Se-NiCo LDH cathode after 10,000 cycles.
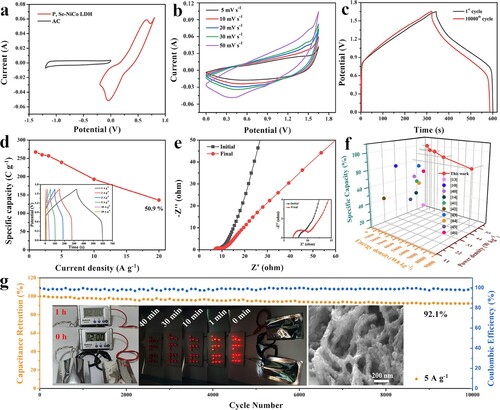
3. Conclusion
In closing, P, Se-NiCo LDH cathode is composed of lapped ultrathin with nanosheet and mesoporous and macroporous structures have been prepared through two-step hydrothermal and low-temperature phosphating treatment. Co-dope of phosphorus and selenium facilitate to improve the conductivity of electrode material. Furthermore, the presence of mesopore and macropore is conducive to providing more conductive active sites and speeding up electron/ion transport, which could improve the rate property and cycle ability of the anode material. The P, Se-NiCo LDH//AC device has a better energy density of 61.23 W h kg−1, and the power density at this time is 825 W kg−1. The energy density can still remain at 31.16 W h kg−1 when the power density reaches 16,496.5 W kg−1. Even at 20 A g−1, the capacitance retention is 50.9%. Moreover, at 5 A g−1, after 10,000 cycles capacity retention rate can maintain at 92.1%. The above-mentioned excellent electrochemical performances of supercapacitor fully prove that phosphorus and selenium element co-doping can significantly improve the electrochemical performances of NiCo LDH, which can meet the needs of modern society for electronic products with high-rate and high energy density.
Supplemental Material
Download MS Word (39.7 MB)Disclosure statement
No potential conflict of interest was reported by the author(s).
Additional information
Funding
References
- Yan J, Li SH, Lan BB, et al. Rational design of nanostructured electrode materials toward multifunctional supercapacitors. Adv Funct Mater. 2020 Jan;30(2):1902564.
- Wu NN, Bai X, Pan D, et al. Recent advances of asymmetric supercapacitors. Adv Mater Interfaces. 2021 Jan;8(1):2001710.
- Huang J, Yuan K, Chen YW. Wide voltage aqueous asymmetric supercapacitors: advances, strategies, and challenges. Adv Funct Mater. 2022 Jan;32(4):2108107.
- Peng M, Wang L, Li L, et al. Molecular crowding agents engineered to make bioinspired electrolytes for high-voltage aqueous supercapacitors. eScience. 2021 Nov 1;1(1):83–90.
- Shao Y, El-Kady MF, Sun J, et al. Design and mechanisms of asymmetric supercapacitors. Chem Rev. 2018 Sep 26;118(18):9233–9280.
- Liang K, Matsumoto RA, Zhao W, et al. Engineering the interlayer spacing by pre-intercalation for high performance supercapacitor MXene electrodes in room temperature ionic liquid. Adv Funct Mater. 2021 Aug;31(33):2104007.
- Zhang KY, Wei YH, Huang J, et al. A generalized one-step in situ formation of metal sulfide/reduced graphene oxide nanosheets toward high-performance supercapacitors. Sci China Mater. 2020 Oct;63(10):1898–1909.
- Garakani MA, Abouali S, Xu ZL, et al. Heterogeneous, mesoporous NiCo2O4-MnO2/graphene foam for asymmetric supercapacitors with ultrahigh specific energies. J Mater Chem A. 2017 Feb;5(7):3547–3557.
- Chodankar NR, Pham HD, Nanjundan AK, et al. True meaning of pseudocapacitors and their performance metrics: asymmetric versus hybrid supercapacitors. Small. 2020 Sep;16(37):2002806.
- Cao RG, Xu W, Lv DP, et al. Anodes for rechargeable lithium-sulfur batteries. Adv Energy Mater. 2015 Aug;5(16):1402273.
- Li JB, Ding ZB, Li JL, et al. Synergistic coupling of NiS1.03 nanoparticle with S-doped reduced graphene oxide for enhanced lithium and sodium storage. Chem Eng J. 2021 Mar;407:127199.
- Abouali S, Akbari Garakani M, Xu Z-L, et al. NiCO2O4/CNT nanocomposites as bi-functional electrodes for Li ion batteries and supercapacitors. Carbon. 2016 Jun 1;102:262–272.
- Zhang D, Guo X, Tong X, et al. High-performance battery-type supercapacitor based on porous biocarbon and biocarbon supported Ni-Co layered double hydroxide. J Alloys Compd. 2020 Oct 5;837:155529.
- Zhang KY, Xu YZ, Lin YC, et al. Enriching redox active sites by interconnected nanowalls-like nickel cobalt phospho-sulfide nanosheets for high performance supercapacitors. Chin Chem Lett. 2021 Nov;32(11):3553–3557.
- Huang J, Xiong YS, Peng ZY, et al. A general electrodeposition strategy for fabricating ultrathin nickel cobalt phosphate nanosheets with ultrahigh capacity and rate performance. ACS Nano. 2020 Oct;14(10):14201–14211.
- Zhang X, Lu W, Tian YH, et al. Nanosheet-assembled NiCo-LDH hollow spheres as high-performance electrodes for supercapacitors. J Colloid Interface Sci. 2022 Jan;606:1120–1127.
- Zang Y, Luo H, Zhang H, et al. Polypyrrole nanotube-interconnected NiCo-LDH nanocages derived by ZIF-67 for supercapacitors. ACS Appl Energy Mater. 2021 Feb;4(2):1189–1198.
- Wei DD, Zhang YL, Zhu XZ, et al. CNT/Co3S4@NiCo LDH ternary nanocomposites as battery-type electrode materials for hybrid supercapacitors. J Alloys Compd. 2020 May;824:153937.
- Jiang D, Wei CAY, Zhu ZY, et al. Synthesis of 3D flower-like hierarchical NiCo-LDH microspheres with boosted electrochemical performance for hybrid supercapacitors. Inorg Chem Front. 2021 Oct;8(19):4324–4333.
- Rao YF, Yuan M, Luo F, et al. One-step laser fabrication of phosphorus-doped porous graphene electrodes for high-performance flexible microsupercapacitor. Carbon. 2021 Aug;180:56–66.
- Manikandan R, Raj CJ, Nagaraju G, et al. Selenium enriched hybrid metal chalcogenides with enhanced redox kinetics for high-energy density supercapacitors. Chem Eng J. 2021 Jun;414:128924.
- Liu S, Yin Y, Ni DX, et al. Phosphorous-containing oxygen-deficient cobalt molybdate as an advanced electrode material for supercapacitors. Energy Storage Mater. 2019 May;19:186–196.
- Wang T, Chen HC, Yu F, et al. Boosting the cycling stability of transition metal compounds-based supercapacitors. Energy Storage Mater. 2019 Jan;16:545–573.
- Du YM, Zhao HM, Wang WS, et al. (Ni,Co)Se@Ni(OH)(2) heterojunction nanosheets as an efficient electrocatalyst for the hydrogen evolution reaction. Dalton Trans. 2021 Jan;50(1):391–397.
- Chen Y, Lian P, Feng J, et al. Tailoring defective vanadium pentoxide/reduced graphene oxide electrodes for all-vanadium-oxide asymmetric supercapacitors. Chem Eng J. 2022 Feb 1;429:132274.
- Qin ZX, Chen YB, Huang ZX, et al. A bifunctional NiCoP-based core/shell cocatalyst to promote separate photocatalytic hydrogen and oxygen generation over graphitic carbon nitride. J Mater Chem A. 2017 Sep;5(36):19025–19035.
- Du W, Zong Q, Zhan J, et al. Tailoring Mo-doped NiCoP grown on (Ni,Co)Se2 nanoarrays for asymmetric supercapacitor with enhanced electrochemical performance. ACS Appl Energy Mater. 2021 Jul 26;4(7):6667–6677.
- Ye B, Zhou J, Cao X, et al. Scalable CNTs/NiCoSe2 hybrid films for flexible all-solid-state asymmetric supercapacitors. ACS Appl Mater Interfaces. 2021 Nov 17;13(45):53868–53876.
- Zhu YL, Zong Q, Zhang QL, et al. Three-dimensional core-shell NiCoP@NiCoP array on carbon cloth for high performance flexible asymmetric supercapacitor. Electrochim Acta. 2019 Mar;299:441–450.
- Wang T, Zhang SL, Yan XB, et al. 2-Methylimidazole-derived Ni-Co layered double hydroxide nanosheets as high rate capability and high energy density storage material in hybrid supercapacitors. ACS Appl Mater Interfaces. 2017 May;9(18):15510–15524.
- Jia H, Li Q, Li C, et al. A novel three-dimensional hierarchical NiCo2O4/Ni2P electrode for high energy asymmetric supercapacitor. Chem Eng J. 2018 Dec;354:254–260.
- Zhang H, Wang TT, Sumboja A, et al. Integrated hierarchical carbon flake arrays with hollow P-doped CoSe2 nanoclusters as an advanced bifunctional catalyst for Zn-air batteries. Adv Funct Mater. 2018 Oct;28(40):1804846.
- Chen XB, Ding JG, Li Y, et al. Size-controllable synthesis of NiCoSe2 microspheres as a counter electrode for dye-sensitized solar cells. RSC Adv. 2018;8(46):26047–26055.
- Yang XJ, Sun HM, Zan P, et al. Growth of vertically aligned Co3S4/CoMo2S4 ultrathin nanosheets on reduced graphene oxide as a high-performance supercapacitor electrode. J Mater Chem A. 2016;4(48):18857–18867.
- Wang HZ, Liu J, Zhang WG, et al. Preparation of binder-free three-dimensional N-doped carbon framework/nickel cobaltate composite for all-solid supercapacitor application. Appl Surf Sci. 2021 Sep;561:149893.
- Karuppaiah M, Benadict Joseph X, Wang S-F, et al. Engineering architecture of 3D-urchin-like structure and 2D-nanosheets of Bi2S3@g-C3N4 as the electrode material for a solid-state symmetric supercapacitor. Energy Fuels. 2021 Aug 5;35(15):12569–12580.
- Kim HS, Cook JB, Lin H, et al. Oxygen vacancies enhance pseudocapacitive charge storage properties of MoO3-x. Nat Mater. 2017 Apr;16(4):454.
- Wang XH, Fang Y, Shi B, et al. Three-dimensional NiCo2O4@NiCo2O4 core-shell nanocones arrays for high-performance supercapacitors. Chem Eng J. 2018 Jul;344:311–319.
- Wang KB, Wang SE, Liu JD, et al. Fe-based coordination polymers as battery-type electrodes in semi-solid-state battery-supercapacitor hybrid devices. ACS Appl Mater Interfaces. 2021 Apr;13(13):15315–15323.
- Zhang MC, Fan HQ, Ren XH, et al. Study of pseudocapacitive contribution to superior energy storage of 3D heterostructure CoWO4/Co3O4 nanocone arrays. J Power Sources. 2019 Apr;418:202–210.
- Shrestha KR, Kandula S, Rajeshkhanna G, et al. An advanced sandwich-type architecture of MnCo2O4@N-C@MnO2 as an efficient electrode material for a high-energy density hybrid asymmetric solid-state supercapacitor. J Mater Chem A. 2018 Dec;6(47):24509–24522.
- Zhang X, Lu Q, Guo E, et al. Nico layer double hydroxide/biomass-derived interconnected porous carbon for hybrid supercapacitors. J Energy Storage. 2021 Jun 1;38:102514.
- Zhang L, Cai P, Wei Z, et al. Synthesis of reduced graphene oxide supported nickel-cobalt-layered double hydroxide nanosheets for supercapacitors. J Colloid Interface Sci. 2021 Apr 15;588:637–645.
- He YZ, Zhang XL, Wang JM, et al. Constructing Co(OH)F nanorods@NiCo-LDH Nanocages Derived from ZIF-67 for high-performance supercapacitors. Adv Mater Interfaces. 2021 Sep;8(17):2100642.
- Wang GR, Jin ZL, Zhang WX. A phosphatized NiCo LDH 1D dendritic electrode for high energy asymmetric supercapacitors. Dalton Trans. 2019 Oct;48(39):14853–14863.
- Li ZY, Huang Y, Zhang Z, et al. Hollow C-LDH/Co9S8 nanocages derived from ZIF-67-C for high-performance asymmetric supercapacitors. J Colloid Interface Sci. 2021 Dec;604:340–349.
- Luo L, Zhou YL, Yan W, et al. Design and construction of hierarchical sea urchin-like NiCo-LDH@ACF composites for high-performance supercapacitors. Ind Crops Prod. 2021 Nov;171:113900.
- Liu X, Ye L, Du YQ, et al. Metal organic framework derived core-shell hollow CoSx@NiCo-LDH as advanced electrode for high-performance supercapacitor. Mater Lett. 2020 Jan;258:126812.
- Gao XY, Zhao YF, Dai KQ, et al. Nicop nanowire@NiCo-layered double hydroxides nanosheet heterostructure for flexible asymmetric supercapacitors. Chem Eng J. 2020 Mar;384:123373.