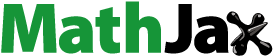
Abstract
Due to the attraction of high specific capacity and abundant raw materials, scientists have extensively researched room-temperature sodium-sulfur (RT-Na/S) batteries in recent years. However, unwanted dendrite growth, huge volume change, lower electrical conductivity and polysulfide shuttle effect make the RT-Na/S batteries performance unsatisfactory. This review focuses on the recent research results of RT-Na/S batteries in cathode material improvement, electrolyte optimization, separator modification and anode protection. The methods and innovations produced by previous researchers are also discussed, and possible solutions are proposed. It is expected that this review may spark fresh ideas for RT-Na/S batteries research.
GRAPHICAL ABSTRACT
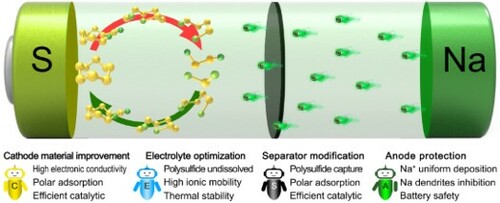
1. Introduction
Globalization has accelerated economic development and technological progress, and the demand for energy is growing. In the context of sustainable development, more and more renewable energy sources such as solar energy and wind energy are gradually replacing the existing fossil energy. [Citation1–3]. However, these renewable energy sources are unevenly distributed in time and space, resulting in large instability in continuous supply of electricity. Therefore, energy storage equipment is required to store electricity and then supply it to end users continuously and stably. Secondary batteries such as lead-acid batteries and lithium-ion batteries have been developed to solve the above problems. The lead-acid battery is currently used on the largest scale as secondary energy storage battery. It has the advantages of high stability and mature technology, but it also has shortcomings such as serious pollution and low energy density. Compared with lead-acid battery, Li-ion batteries create less pollution, possess a higher energy density and better stability. But in the same way, Li-ion batteries are suffering from capacity bottlenecks and expensive materials. Based on these many problems of existing batteries, it is necessary to develop next-generation low-cost and high-energy-density batteries [Citation4–7]. In recent years, more and more researchers have devoted themselves to the research of Na-S batteries, whose cathode and anode materials are S and Na respectively. Furthermore, these two elements are widely distributed on the earth, and the extraction process is mature, so the cost is lower. At the same time, the theoretical energy densities of Na and S are relatively high, which can meet the needs of large-scale energy storage. To summarize conclusion, Na-S batteries are very promising for application [Citation8–10].
1.1. History of Na-S batteries
Research on Na-S batteries originated in the 1960s, with the first research focused on High-Temperature Sodium-Sulfur (HT-Na/S) batteries, which operate around 300–350 °C. A molten Na anode (melting point=98 °C), a molten sulfur cathode (melting point = 118 °C) and ceramic β'-Al2O3 as solid electrolyte are assembled into the HT-Na/S batteries [Citation11]. HT-Na/S batteries avoid the dendrite problem and have high electrical conductivity. However, it also has the defects of high working temperature, high risk, low energy density and high operation cost. And then, the Intermediate-Temperature Sodium-Sulfur (IMT-Na/S) batteries were innovated in the 1970s and operate between 120–300 °C. The IMT-Na/S batteries also eliminated the dendrite problem, but the electronic conductivity and the utilization of sulfur also decreased. Researchers have been intensively investigating Room-Temperature Sodium-Sulfur (RT-Na/S) batteries, which operate around 25 °C-35 °C. RT-Na/S batteries can completely convert S8 to Na2S, so they have a high theoretical energy density (1274 Wh kg−1) [Citation12–15]. However, RT-Na/S batteries also suffer from anode dendrite growth, sulfur cathode volume expansion, low electronic conductivity, and polysulfide shuttle effect. Therefore, most of the current research is carried out around the problems above and is committed to promoting the commercialization of RT-Na/S batteries (Figure ). Since the work in this paper is all researched and discussed around RT-Na/S batteries, they will be referred to as Na-S batteries in the following [Citation16–20].
Figure 1. Historical map of Na-S batteries research and development. Copyright 2022, Wiley-VCH GmbH [Citation11].
![Figure 1. Historical map of Na-S batteries research and development. Copyright 2022, Wiley-VCH GmbH [Citation11].](/cms/asset/929091e7-7215-43db-ad9d-14d3d12d3c05/tmrl_a_2092428_f0001_oc.jpg)
1.2. Comparison with Li-S batteries
The technology of Li-ion batteries has gradually matured and commercialized, while the research on Na-ion batteries has just started. There are many similarities between Li and Na, and the related research ideas of Li-ion batteries can be applied to Na-ion batteries. Li EΘ(Li+/Li0) = −3.04 V vs. standard hydrogen electrode, with a theoretical specific capacity of 3860 mAh g−1 [Citation21]. Similarly, Na, which belongs to the same main group as Li, is an abundant and low-cost element with physical and chemical properties similar to those of Li. EΘ(Na+/Na0) = −2.71 V vs. standard hydrogen electrode, only 0.33 V lower than Li [Citation22]. However, this does not mean that the voltage of Na-ion batteries must be 0.33 V lower than that of Li-ion batteries. Because the voltage of the batteries depends on the potential difference between the positive and negative materials at the electrodes.
The theoretical energy density of the Li-S batteries is 2600 Wh kg−1, and the overall reaction can be written as:
(1)
(1)
The theoretical energy density of the Na-S batteries is 1245 Wh kg−1, and the reaction can be written [Citation23,Citation24]:
(2)
(2)
The working mechanism of Na-S battery is similar to Li-S battery, which realizes the transfer of electrons through the conversion reaction of S8 and S2-. Additionally, Na does not react electrochemically with aluminum (Al), so Na-S batteries can use Al foil instead of copper (Cu) foil as the anode collector. Therefore, despite their low energy density, Na-S batteries have a low-cost superiority, which makes Na-S batteries one of the candidates for future energy storage systems. Although the research on Na-S batteries has achieved many insightful results, some problems still have to be solved. Therefore, in order to better promote the research of Na-S battery, the author starts with the mechanism of Na-S battery, and analyzes its advantages as well as various challenges [Citation25–28]. In addition, through the analysis and interpretation of the existing research results, readers can more intuitively understand the research ideas of various technical routes and the advantages. And we hope that readers can obtain better research ideas for Na-S batteries through intensive reading of this review, and can further promote the commercialization of Na-S batteries.
2. Intrinsic challenges for Na-S batteries
To achieve efficient and smooth charge–discharge cycling of Na-S batteries, several challenges need to be overcome (Figure ). In this chapter, the problems of Na-S batteries will be discussed, and the root causes of their occurrence will be analyzed.
Firstly, the Na metal is relatively active. It will be instantly oxidized when exposed to the air, so the storage and use conditions are relatively harsh [Citation29].
Secondly, most Na-ion batteries use organic electrolytes. During the first few cycles of charge and discharge, the organic electrolyte is decomposed and then forms an ‘unstable solid electrolyte interphase’ (SEI) on the surface of the Na anode [Citation30]. The formation of the SEI film can protect the Na anode to a certain extent and further block its subsequent reaction with the electrolyte. However, the uneven deposition of Na brings about dendrite problems. The growth of dendrites will continue to consume electrolyte and form SEI films on the dendrite surfaces, resulting in low coulombic efficiency and low capacity. In addition, at high current and long cycles, dendrites are more severe and may puncture the separator and cause short circuits [Citation31].
Thirdly, the radius of the Li+ is 0.76 Å, while the Na+ is 1.02 Å. Therefore, researchers need to find a suitable Na+ storage material to facilitate the reversible insertion and de-insertion of Na+ [Citation32]. At the same time, the sodiation and desodiation will cause a huge change in the electrode volume, which may lead to the collapse of the electrode structure. More seriously, the collapse of the electrode structure will cause damage to the existing SEI film on the surface, resulting in the need to consume additional electrolytes to form a new SEI film on the electrode surface. This effect results in unsatisfactory long-cycle performance for Na-ion batteries. Meanwhile, in the reaction on the sulfur cathode side, the volume change from S to S2- expands by 80%, leading to more serious instability of the cathode [Citation23].
Fourthly, the larger radii of Na+ and S2- reduce the ion mobility and the electrochemical reaction rate. This will lead to increased polarization during the reaction, slow reaction kinetics, and insufficient release of capacity [Citation28].
Fifthly, polysulfide intermediates (sodium polysulfide, Na2Sx, 4 ≤ x ≤ 8, NaPSs) produced during charging/discharging of the battery are readily dissolved in organic solvents. In the Na-S battery system based on ether electrolyte, NaPSs is easily dissolved in the electrolyte, which is beneficial to accelerating the reaction kinetics. However, driven by the concentration gradient and electric field, the NaPSs will shuttle through the pores of the separator to the anode side, triggering the polysulfide shuttle effect. More seriously, the NaPSs in the electrolyte on the anode side will continue to react with the Na to generate insoluble Na2S2/Na2S on the negative electrode surface. Moreover, the continuous deposition of Na2S2/Na2S on the anode will destroy the SEI film on the surface of the Na anode, which further leads to the instability of the Na anode. Further, the above process is irreversible. Therefore, as the reaction progresses, the active material on the cathode gradually decreases, resulting in poor long-term cycle performance of the Na-S batteries [Citation33].
Sixthly, due to the potential difference between the anode and cathode of the battery, even when the battery is in a static state, a slow redox reaction occurs inside the battery, resulting in a loss of capacity [Citation34]. However, the insoluble S8 will react with the ether electrolyte to form soluble NaPSs. Further, NaPSs undergo a shuttle effect to react with the Na anode, leading to self-discharge. Therefore, the self-discharge of Na-S batteries also needs to be solved urgently.
In order to better solve the problems existing in the Na-S batteries, it is necessary to fully research its reaction mechanism. Only by understanding the working mechanism of Na-S batteries can subsequent researchers better and more effectively solve the problems faced by Na-S batteries.
3. Storage mechanisms of Na-S batteries
Similar to the Li-S batteries, the reaction of the Na-S batteries is greatly affected by the electrolyte. In the systems of different electrolyte, the reaction mechanism of Na-S batteries is also very different.
For example, NaPSs have a higher solubility in ether electrolytes, while S8 and Na2S2/Na2S show lower solubility in ether electrolytes. The characteristics above make the reaction of the Na-S battery based on the ether electrolyte involve the transformation of solid–liquid-solid phase, and the reaction is relatively complicated. While, in ester electrolytes, S8, NaPSs and Na2S2/Na2S all have low solubility, so the whole reaction involves solid–solid transformation. Besides, in solid electrolytes, the reactions involved are relatively simple and are therefore discussed in the chapter on electrolytes. Therefore, this chapter will further analyze the reaction mechanism of Na-S batteries from the ether and ester electrolyte systems. [Citation6,Citation11].
3.1. Storage mechanisms in ether-based electrolytes
The CV and discharge curve of Na-S batteries in tetraethylene glycol dimethyl ether (TEGDME) electrolyte is shown in Figure a-b. It can be seen from the CV that the Na-S batteries based on ether electrolyte have two obvious reduction peaks at 2.20 and 1.65 V, which corresponds to the two-stage discharge plateau in the discharge curve. Therefore, the entire discharge curve can be simply divided into four regions [Citation35,Citation36]:
Figure 3. (a) CV, (b) discharge curve of Na-S batteries with 1.5 M NaClO4 + 0.3 M NaNO3 in TEGDME electrolyte. Copyright 2014, Wiley-VCH [Citation35]. (c) CV, (d) A practical discharge profile of Na-S batteries with 1 M NaClO4 in 1:1 ethylene carbonate (EC) /propylene carbonate (PC) and 3 wt% fluoroethylene carbonates (FEC). Copyright 2021, Cell Reports Physical Science [Citation37].
![Figure 3. (a) CV, (b) discharge curve of Na-S batteries with 1.5 M NaClO4 + 0.3 M NaNO3 in TEGDME electrolyte. Copyright 2014, Wiley-VCH [Citation35]. (c) CV, (d) A practical discharge profile of Na-S batteries with 1 M NaClO4 in 1:1 ethylene carbonate (EC) /propylene carbonate (PC) and 3 wt% fluoroethylene carbonates (FEC). Copyright 2021, Cell Reports Physical Science [Citation37].](/cms/asset/19367b08-8035-4b89-92a9-494640782c4f/tmrl_a_2092428_f0003_oc.jpg)
Region I is a relatively high voltage plateau with Na/Na+ of approximately 2.2 V, corresponding to the conversion of insoluble S8 to soluble Na2S8:
(3)
(3)
Region II is the first inclined region with a voltage range of 2.20 V-1.65 V, corresponding to the conversion of soluble long-chain NaPSs Na2S8 to medium-chain NaPSs Na2S4:
(4)
(4)
Region III refers to the lower voltage plateau at about 1.65 V, which corresponds to the conversion of dissolved Na2S4 into Na2S3, Na2S2 and Na2S:
(5)
(5)
(6)
(6)
(7)
(7)
Region IV is the second inclined region with a voltage range of 1.65 V-1.2 V, which corresponds to the solid–solid reaction of Na2S2 and Na2S:
(8)
(8)
Among the four reaction zones, zone II is the most complicated zone, and is affected by the chemical balance between each type of NaPSs species in the solution. The following side reactions may also occur:
(9)
(9)
(10)
(10)
(11)
(11)
Generally, NaPSs have high solubility in ether electrolytes, which is conducive to the forward progress of the reaction and can improve the reaction rate. However, this also exacerbates the shuttle effect of polysulfides, making the cycling stability of Na-S batteries unsatisfactory [Citation38–40].
3.2. Storage mechanisms in ester-based electrolytes
The most significant difference between ester solvents and ether solvents is the violent nucleophilic reaction between the nucleophilic Sx2- and the ester solvents. However, the solubility of NaPSs in ester electrolytes is lower than that in ether electrolytes. Therefore, the shuttle effect of polysulfides in the Na-S batteries based on the ester electrolyte is effectively suppressed, and the utilization rate of sulfur is improved. Many researches on Na-S batteries reported in recent years have used ester electrolytes [Citation40,Citation41]. For example, Yan et al. used nano-silver-modified core–shell carbon spheres to unload sulfur as the cathode, and 1 M NaClO4-EC/PC/FEC as the electrolyte, obtaining satisfactory performance and cycle stability [Citation37]. From Figure c, there are also two obvious peaks in the Na-S batteries based on the ester electrolyte. But except for these two peaks, the areas of other parts are basically negligible. Moreover, only two discharge platforms in the ester electrolyte contribute to the capacity, compared with Figure d. Besides, other parts have little contribution to the capacity, which is basically consistent with the CV results. This effectively illustrates that the reaction of the ester electrolyte-based Na-S battery is relatively simple, involving only the transformation of the solid–solid phase. And it is not difficult to find that there is a high irreversible capacity below 1.4 V in the first cycle discharge curve, which is related to the formation of SEI film. In addition, the decomposition of the electrolyte will also result in the formation of cathode electrolyte interphase (CEI) on the sulfur cathode. And then, in the subsequent cycles, the generated CEI layer promotes the sulfur particles in the cathode to undergo a quasi-solid phase transition, reducing the generation of soluble Sx2- intermediates. Thus, this simple reaction process is more conducive to the specific analysis of the problems existing in the Na-S batteries, and the problems can be solved in a more targeted manner [Citation28,Citation42–44].
4. Strategies for the effective use of sulfur cathode materials
Since the working mechanism of Li-S batteries is similar to that of Na-S batteries, researchers can learn from the research results of Li-S batteries to design a more effective solution and solve the problem of Na-S batteries [Citation45].
In the existing research, Li-S batteries generally use three-dimensional (3D) carbon material composite sulfur as the cathode. Firstly, sulfur can transfer electrons faster with the help of the 3D carbon framework, increasing the reaction rate. Secondly, the 3D carbon framework can also effectively limit the dissolution of sulfur, and provide certain non-polar adsorption to limit the shuttle of polysulfides. Moreover, in order to better solve the shuttle effect of polysulfides, polar materials are also introduced to provide extra polar adsorption. The methods used in the cathode of Na-S batteries is basically the same as those mentioned above. Therefore, this review will describe the research on the cathode system of Na-S batteries from the aspects of carbon (porous carbon, doped carbon), metal sulfides/oxides, metals/mono-atoms, and other materials. And then, by analyzing the results obtained in the existing cases, the future research direction of the Na-S batteries cathode is speculated [Citation46–54].
4.1. Sulfur/ carbon composites
Initially, Na-S batteries used thermal diffusion (melt impregnation) technique to encapsulate sulfur into a 3D carbon framework, such as carbon nanotubes, hollow carbon nanospheres, carbon nanofibers, and porous nanocarbons, allowing better bonding between sulfur and carbon [Citation55–60]. And then, this chapter will analyze the existing research results of Na-S batteries step by step, starting from the use of pure carbon materials for cathode sulfur storage [Citation61,Citation62].
4.1.1. Sulfur/ pure carbon
Xin et al. experimentally produced carbon nanotubes (CNT) wrapped with a layer of sulfur-containing microporous carbon tubes (MPC) on the outside (S/(CNT@MPC)), forming a concentric circular structure similar to that of a cable (Figure a) [Citation63]. The composite material was then used as a cathode sulfur storage material for Na-S batteries, and the results showed that microporous carbon (pore size <0.5 nm) made sulfur only exist in the form of small molecules (S2–4) during the entire reaction process. The design of microporous carbon makes it difficult for NaPSs to escape from the carbon cage, and avoids the formation of soluble NaPSs intermediates. Furthermore, the microporous carbon effectively inhibits the shuttle of polysulfides. In the CV curves, the redox peak was shifted and had a sharp peak in the first lap due to the activation of the electrode and the formation of the SEI. This phenomenon gradually improved in the subsequent laps, indicating that the electrochemical reaction of the battery was relatively smooth. Figure b shows that at a current density of 0.1C (1 C = 1675 mAh g−1), S8 is gradually converted to Na2S and releases a specific capacity of 1610 mAh g−1. And the battery capacity reached 1148 mAh g−1 on the first charge and the initial efficiency of battery was 71.3%. The efficiency is relatively high considering the existence of electrode activation and SEI film formation in the first circle. Subsequently, the electrochemical performance gradually stabilizes, and the coulombic efficiency rapidly increases to 100%. After 2 ∼ 3 charge/discharge cycles, the capacity of the cell is maintained at around 900 mAh g−1 and 800 mAh g−1 (Figure c).
Figure 4. (a) SEM and TEM images of S/(CNT@MPC). (b) Diagram of the mechanism during discharge. (c) Cycling Performance at different currents. Copyright 2013, WILEY-VCH [Citation63]. (d) TEM images of S@iMCHS. (e) Diagram of fixed elemental sulfur. (f) Rate capability. Copyright 2016, American Chemical Society [Citation64]. (g) HRTEM images of the microporous carbon. (h) Diagram of material limitation of sulfur. (i) Schematic diagram of current capacity at different currents. Copyright 2020, WILEY-VCH [Citation65].
![Figure 4. (a) SEM and TEM images of S/(CNT@MPC). (b) Diagram of the mechanism during discharge. (c) Cycling Performance at different currents. Copyright 2013, WILEY-VCH [Citation63]. (d) TEM images of S@iMCHS. (e) Diagram of fixed elemental sulfur. (f) Rate capability. Copyright 2016, American Chemical Society [Citation64]. (g) HRTEM images of the microporous carbon. (h) Diagram of material limitation of sulfur. (i) Schematic diagram of current capacity at different currents. Copyright 2020, WILEY-VCH [Citation65].](/cms/asset/8dd0566f-6316-4087-939d-7ff4719f92da/tmrl_a_2092428_f0004_oc.jpg)
Figure 5. (a) TEM images of N, S-HPC and its corresponding EDAX elemental mapping. (b) Schematic diagram of the synthesis of N, S-HPC. (c) Long cycling Performance. Copyright 2016, Elsevier [Citation74]. (d) The typical TEM images of covalent-SC. (e) Diagram of the covalent-SC reaction mechanism. (f) Rate performance. Copyright 2020, American Chemical Society [Citation75]. (g) HAADF-STEM and the corresponding EDS elemental mapping of the S@MCCBs. (h) Schematic Illustration of the Features of the MCCBs as a sulfur host. (i) Long cycling Performance. Copyright 2021, American Chemical Society [Citation54].
![Figure 5. (a) TEM images of N, S-HPC and its corresponding EDAX elemental mapping. (b) Schematic diagram of the synthesis of N, S-HPC. (c) Long cycling Performance. Copyright 2016, Elsevier [Citation74]. (d) The typical TEM images of covalent-SC. (e) Diagram of the covalent-SC reaction mechanism. (f) Rate performance. Copyright 2020, American Chemical Society [Citation75]. (g) HAADF-STEM and the corresponding EDS elemental mapping of the S@MCCBs. (h) Schematic Illustration of the Features of the MCCBs as a sulfur host. (i) Long cycling Performance. Copyright 2021, American Chemical Society [Citation54].](/cms/asset/4f3782ee-2096-4c6a-85a7-839ffaf43e7a/tmrl_a_2092428_f0005_oc.jpg)
Wang et al. used interconnected mesoporous carbon hollow nanospheres (iMCHS) as an effective sulfur host through melt diffusion method. Sulfur can be observed encapsulated inside the hollow carbon nanospheres (Figure d) [Citation64]. At high temperatures, the carbon pores on the surface of hollow nanospheres are opened, which makes sulfur can easily enter and diffuse. And this method promotes the close contact between the 3D carbon spherical framework and the nano-sulfur, so that the nano-sulfur exhibits high electrochemical activity [Citation66]. Furthermore, the sulfur is attached to the 3D carbon skeleton and can migrate electrons through the carbon skeleton. Also, the hollow structure not only allows full penetration of the electrolyte and migration of small molecules, but also limits the escape of NaPSs, thus suppressing the shuttle effect (Figure e). It was shown by X-ray diffraction (XRD) data that the Na2S formed by discharge was not converted into long-chain NaPSs when the cell was recharged to 2.8 V, indicating that the conversion of Na2S is restricted. The S@ iMCHS -based Na-S battery also exhibits excellent performance at different current densities, 390 mAh g−1 at 0.1 A g−1, and 127 mAh g−1 at 5 A g−1 (Figure f).
Guo et al. produced ultra-microporous carbon with pores of about 0.5 nm (Figure g), using coffee grounds as raw material [Citation65]. Subsequently, by the melt diffusion method, sulfur is first diffused into larger pores, and then the high temperature is used to crack S8 into small molecules of S2–4, so that they are distributed into the ultra-microscopic pores. Theoretical calculations have determined that ultra-microporous pores (< 0.5 nm) can effectively inhibit the formation of NaPSs. In this structure, sulfur can only exist as a small molecule, and its reaction product in the cell is Na2S (Figure h). The material was also found to contain Na-C bonds, and this structure facilitates the oxidation of Na2S during the discharge process. Since the carbon pores are very small, there is a strong interaction between the product Na2S and the carbon skeleton. This action leads to an increase in the current-carrying capacity of the carbon skeleton and results in a significant improvement in electronic conductivity [Citation67]. Furthermore, as the reactants and products are mostly small molecules, the reaction kinetics are enhanced compared to the larger NaPSs molecules, resulting in faster reaction rates and increased capacity. The material has a good multiplicative property, low self-discharge, good cycling stability and negligible capacity loss after 2000 charge/discharge cycles at 1 C (Figure i). Moreover, Wu et al. also demonstrated that the structure and porosity of the 3D carbon framework played a crucial role in NaPSs [Citation68].
According to the research results above, the use of 3D carbon skeleton as a sulfur host is a meaningful and promising research direction. Firstly, electrons can be transported through the framework of carbon materials, which speeds up the reaction. Secondly, the 3D carbon framework limits the dissolution of NaPSs through non-polar adsorption and smaller pores. Thirdly, the introduction of porous carbon can make the sulfur dispersed more uniformly, make it more wettable with the electrolyte, and contribute to the reaction. However, the non-polar adsorption capacity of carbon is limited, so that NaPSs cannot be effectively bound, resulting in poor cycling stability. Meanwhile, simply improving the conductivity cannot effectively speed up the conversion of NaPSs. Therefore, the introduction of heteroatom doping seems to be a feasible solution based on the carbon framework as the sulfur-loaded material [Citation69]. This approach increases the activation sites on the 3D carbon skeleton. Moreover, the introduction of multiple atoms can obtain faster ionic conductivity with larger molecular layer spacing, thus speeding up the reaction and maintaining the stability of the electrode. In addition, the introduction of heteroatoms and polar adsorption sites enhance the binding ability of NaPSs, which can effectively inhibit the escape of NaPSs. Besides, heteroatoms can also slightly enhance the defects of the carbon skeleton, which conduce to the catalytic conversion of NaPSs and improve the reaction kinetics [Citation70–73].
4.1.2. Sulfur/ heteroatom doped carbon
Qiang et al. reported that a hierarchical porous carbon (N, S-HPC) with high heteroatom content of N and S co-doped was prepared, using porous phenolic resin with melamine and benzyl disulfide carbonization [Citation74]. The heteroatoms such as N and S were effectively bound to the NaPSs in the nanocarbon network by being distributed in the 3D carbon skeleton through van der Waals forces (Figure a-b). N, S-HPC limits the reaction of NaPSs with electrolytes and enables excellent long-cycle capacity stability under high current (4.6 A g−1) (Figure c).
Yan et al. prepared carbon networks with S–C covalent bonds, using carbon disulfide and red phosphorus as raw materials [Citation75]. The introduction of heteroatoms increases the interlayer spacing of carbon, which is beneficial to the migration of Na+. In addition, the S–C in this material forms covalent bonds and is uniformly loaded inside and outside of the carbon skeleton (Figure d-e). This increases the active sites, promotes the adsorption of Na-ions, enhances the electrical conductivity, and possesses a higher sodium storage capacity (Figure f). Lim et al. demonstrated that the combination of S–C covalent bonds contributes to inhibiting the shuttle effect of polysulfides [Citation76]. And this research further shows that the morphology of the synthesized material has a great influence on the performance of Na-S batteries.
Li et al. obtained hollow and porous N/O co-doped nanoboxes as sulfur hosts by etching ZIF-8 followed by carbonization (MCCBs) [Citation54]. The porous and hollow carbon nanoboxes of MCCBs facilitate the dissolution of bound sulfur (Figure g). Stress analysis on the 3D structure of the carbon nanobox shows that it has strong structural stability and can withstand large volume changes and application of stress. Furthermore, the co-doping of N/O provides non-polar adsorption, which helps to further suppress anchored NaPSs (Figure h). With the help of DFT calculations, it is also verified that the porous hollow carbon structure and the doping of heteroatoms in MCCBs are beneficial to the immobilization of NaPSs. Moreover, the high stability of the MCCBs structure was verified by in situ transmission electron microscopy, which can effectively alleviate the problem of carbon skeleton damage caused by volume expansion. Therefore, the Na-S battery based on S@MCCBs as the cathode achieves satisfied long-cycle stability. At the current density of 5 A g−1, it still maintains a reversible capacity of 362 mAh g−1 after 800 cycles (Figure i).
The research results above show that the introduction of heteroatoms effectively improves the long-cycle stability of Na-S batteries. The heteroatoms can provide polar adsorption to inhibit the shuttle of polysulfides, and also catalyze the conversion of NaPSs. The heteroatoms-doped method has effectively solved the problems existing in Na-S batteries; it also opened up new ways for subsequent research, for example, doping nano-metal particles (metal sulfides, metal oxides, and metal atoms) on the 3D carbon skeleton seems to provide better polar adsorption and better catalytic performance than doping heteroatoms [Citation77–81].
4.2. Sulfur/ carbon composites
Wang et al. used an electrostatic spinning process to embed SnS nanoparticles into S and N co-doped conductive carbon fibers [Citation82]. The composite disperses the nanoparticles in a conductive fiber network, which can be effectively used to provide a large reaction area with the laminar structure of the network. It can also accommodate more Na+ to react and provide a buffer for volume changes (Figure a-b). The SnS@SNCF composite fabricated by this method has a capacity of about 300 mAh g−1 at 0.1 A g−1 (Figure c).
Figure 6. (a) SAED pattern and elemental mapping results of SnS@SNCF-55. (b) Diagram of sodium storage in SnS@SNCF-55. (c) Cycling performance. Copyright 2018, Elsevier [Citation82]. (d, e) Comparison of STEM-EDS mapping images with charge/discharge reaction. (f) Rate capability of NiS2@NPCTs/S (red) and NPCTs/S (blue). Copyright 2019, Springer Nature [Citation83]. (g) Schematic diagram of the electron transfer of Co-ions in the catalytic conversion of polysulfides. (h) Schematic diagram of the synthesis of S@Co1-CoS2/NC. (i) Electrochemical performance graph. Copyright 2022, WILEY-VCH [Citation84].
![Figure 6. (a) SAED pattern and elemental mapping results of SnS@SNCF-55. (b) Diagram of sodium storage in SnS@SNCF-55. (c) Cycling performance. Copyright 2018, Elsevier [Citation82]. (d, e) Comparison of STEM-EDS mapping images with charge/discharge reaction. (f) Rate capability of NiS2@NPCTs/S (red) and NPCTs/S (blue). Copyright 2019, Springer Nature [Citation83]. (g) Schematic diagram of the electron transfer of Co-ions in the catalytic conversion of polysulfides. (h) Schematic diagram of the synthesis of S@Co1-CoS2/NC. (i) Electrochemical performance graph. Copyright 2022, WILEY-VCH [Citation84].](/cms/asset/621ff2e7-505b-4203-94af-a0799221314c/tmrl_a_2092428_f0006_oc.jpg)
Yan et al. injected S powder along with NiS2 nanocrystals into N-doped porous carbon nanotubes to form NiS2@NPCTs composites [Citation83]. The material relies mainly on the mutual synergistic interaction between NiS2, N and C (Figure d). NiS2@NPCTs relies on the 3D structure and the physical limiting effect of polar and nonpolar forces, as well as the bonding interaction of internal chemical bonds. This material can effectively bind NaPSs and promote the rapid conversion of NaPSs to Na2S (Figure e). Meanwhile, the porous carbon nanotube structure can effectively accommodate S atoms and provide a buffer for the drastic volume change. And the structure of carbon nanotubes can provide a fast channel for electron transport, making the reaction more sufficient. The Na-S batteries based on NiS2@NPCTs can release a high specific capacity of 960 mA g−1 in the first cycle at 1 A g−1, and still maintain a respectable specific capacity of 401 mAh g−1 after 750 cycles. After tested at simultaneous multipliers with 0.1, 0.2, 0.5, 1, 2 and 5 A g−1 currents, it was found that the capacity recovered to 674 mA h g−1 when returned to 0.1 A g−1, which is consistent with the material's ability to maintain its capacity at 0.1 A g−1 current for 200 cycles at a capacity of 650 mAh g−1 (Figure f).
Lei et al. researched the mechanism of the catalytic conversion of Co-ions to NaPSs (Figure g). S@Co1/CoS2/NC was synthesized as sulfur cathode material by a three-step method (Figure h) [Citation84]. The escape of NaPSs was suppressed by rationally constructing adsorption and catalytic sites, and the conversion of NaPSs was accelerated. Moreover, this result was also validated by computation and characterization. Besides, the electrochemical performance map shows that the Na-S battery based on S@ Co1/CoS2/NC achieves ultra-stable long-cycle charge–discharge (Figure i).
4.3. Others
Ma et al. prepared CSB@TiO2 nanofibers by using BaTiO3 nanoparticles as doping atoms for porous carbon nanofibers and melt diffusing them into sulfur atoms [Citation85]. This material further enhanced the immobilization of NaPSs. The cathode material exhibited higher rate performance and excellent durability compared with pure C/S and C/S/BaTiO3 electrodes (Figure a-b). The CSB@TiO2 electrode maintained 524.8 mAh g−1 after 1400 cycles at 1 A g−1 and 382 mAh g−1 after 3000 cycles at 2 A g−1. The main confining effect of the molecular network formed by BaTiO3-C-TiO2 inhibits the shuttle effect of polysulfides (Figure c). Moreover, Liu et al. fabricated S-doped Nb2O5 hollow nanospheres, which were uniformly and homogeneously distributed in the S-reduced graphene oxide (rGO) lamellar structure [Citation53].
Figure 7. (a) SEM and the corresponding elemental mapping images of the CSB@TiO2 nanofiber. (b) Schematic illustration of the preparation process. (c) The long-term cycling performance. Copyright 2018, WILEY-VCH [Citation85]. (d) Characterization of the S@Ni-NCFs composite. (e) S@Ni-NCFs charging and discharging diagram. (f) Rate capabilities of S@Ni-NCFs (orange) and S@NCFs (blue). Copyright 2020, WILEY-VCH [Citation86]. (g) HRTEM, STEM images of FCNT@Co3C-Co/S. (h) Schematic Diagram. (i) long cycling performances. Copyright 2020, Elsevier [Citation87].
![Figure 7. (a) SEM and the corresponding elemental mapping images of the CSB@TiO2 nanofiber. (b) Schematic illustration of the preparation process. (c) The long-term cycling performance. Copyright 2018, WILEY-VCH [Citation85]. (d) Characterization of the S@Ni-NCFs composite. (e) S@Ni-NCFs charging and discharging diagram. (f) Rate capabilities of S@Ni-NCFs (orange) and S@NCFs (blue). Copyright 2020, WILEY-VCH [Citation86]. (g) HRTEM, STEM images of FCNT@Co3C-Co/S. (h) Schematic Diagram. (i) long cycling performances. Copyright 2020, Elsevier [Citation87].](/cms/asset/74c8167f-4dff-40a0-93e8-fb8e11fa13fc/tmrl_a_2092428_f0007_oc.jpg)
Guo et al. synthesized a 3D network body (S@Ni-NCFs) composed of Ni-doped carbon fibers and nickel hollow spheres, using an electrostatic spinning method [Citation86]. The composite material is designed with Ni hollow spheres connected in series with carbon fibers. In the redox reaction, each Ni hollow sphere can effectively accommodate S atoms and provide buffer space, while the electron conduction can be effectively enhanced with the help of a carbon fiber network. Ni atoms reduce the activation energy of NaPSs reduction and accelerate the reaction (Figure d-e). This enables the S@Ni-NCFs cathode to exhibit excellent rate capability and excellent cycling performance. A specific capacity of 738.7 mAh g−1 was guaranteed at a current of 0.2 C, and a specific capacity of 249.8 mAh g−1 was still maintained at a current of 2 C. At the same time, the S@Ni-NCFs electrodes maintained 738.7, 565.6, 481.1, 401.9, 311.1, 249.8 and 181.7 mAh g−1 at multiplier currents of 0.2, 0.3, 0.5, 1.0, 2.0, 3.0 and 5.0 C and to 578.5 mAh g−1, when the current returns to 0.2 C (Figure f).
Liu et al. distributed Co single atoms in the carbon skeleton while introducing ZnS nanoparticles [Citation88]. The composite exhibited remarkable cycling performance (640 mAh g−1) with high capacity retention even after 500 cycles at 0.1 A g−1. It mainly lies in the strong adsorption and catalytic force provided by the doped Co and ZnS nanoparticles, which greatly promote the conversion of NaPSs and the reduction of Na2S and prevent the dissolution of NaPSs.
Qin et al. designed a Co3C-Co composite F-doped carbon nanotube (FCNT@Co3C-Co) array for sulfur fixation in the cathode of Na-S batteries (Figure g) [Citation87]. On the basis of the previous use of heteroatom-doped carbon to achieve adsorption and catalysis of NaPSs, it also introduced a Co3C heterojunction structure. With the dual action of F atoms and Co3C, the anchoring effect of NaPSs is realized. Moreover, the research shows that the presence of Co3C effectively promotes the cleavage of long-chain NaPSs, while fluorine-doped carbon nanotubes accelerate the further conversion of short-chain NaPSs (Figure h). And then, this article proposes that the heterojunction structure of Co3C and Co can improve the performance of Na-S batteries. Therefore, a high specific capacity of 1364 mAh g−1 was obtained at a current density of 0.1 C, as well as high long-term cycling stability at a current density of 2 C (Figure i).
Based on the research results above, researchers have achieved remarkable results in the field of cathode materials for Na-S batteries. From the beginning, the S/C composite cathode solves the problem of low conductivity of Na-S batteries, and uses the 3D carbon framework to provide a buffer for the volume change of the redox of Na and S. The subsequent introduction of heteroatoms effectively makes up for the lack of carbon materials’ ability to bind NaPSs. The doping of heteroatoms not only provides polar adsorption to effectively limit the escape of NaPSs, but also provides a certain catalytic effect to accelerate the conversion of NaPSs. And then, in order to better solve the problems existing in Na-S batteries, the researchers combined metal oxides, sulfides, metal elements, etc. with carbon materials as the cathode sulfur-host. Existing findings suggest that metal compounds and metal atoms can provide stronger polar adsorption, which can further bind NaPSs. Furthermore, they can also catalyze NaPSs, speeding up the process of the reaction. Therefore, in order to suppress the shuttle of polysulfides, improve the utilization rate of sulfur and fully release the capacity of Na-S batteries, We can conduct in-depth research from the following aspects: (1) Materials with high electronic conductivity are used to facilitate electron transport; (2) Fabrication of 3D structures to physically confine sulfur and NaPSs; (3) Use polar and non-polar materials to adsorb NaPSs; (4) Introducing NaPSs catalyst to accelerate the reaction (Figure ) [Citation50,Citation87].
5. Electrolyte design for Na-S batteries
Generally, battery consists of positive and negative electrodes, electrolytes, separators, and current collectors. The reaction of a battery is not only related to its anode and cathode materials, but also closely related to the type of electrolyte. The electrolyte is an important carrier for ion transport, and also plays a role in isolating electron transport to avoid short circuits between positive and negative electrodes (Figure ). At present, the electrolytes of Na-S batteries can be roughly divided into three types: solid electrolytes, liquid electrolytes and hybrid electrolytes. The working principles of sodium-sulfur batteries based on different electrolytes are different, and each system has its advantages and disadvantages. Therefore, this chapter will discuss different electrolytes from multiple perspectives, so as to further analyze and speculate the direction of possible future research [Citation89–93].
5.1. Liquid electrolyte
In Na-S batteries, the liquid electrolyte has at least two components: the solvent and the sodium salt. The solvent can be esters, ethers, etc. In terms of solvent selection, three general points are looked at: dielectric constant, viscosity, and the electron-donating properties of solvent. In general, a high dielectric constant favors the ionization of sodium salts, a strong electron-donating capacity can favor the dissolution of electrolyte salts, and a low viscosity can increase the mobility of ions and contribute to the conductivity. The solute-sodium salt is mainly used to provide current-carrying capacity. In the selection of sodium salts, there are generally several considerations, such as the ability to exist stably in the battery system, low self-discharge rate, high conductivity, low ohmic pressure drop-in solution, safety, non-toxicity, non-pollution, etc. In Na-S batteries, the more used sodium salts are NaPF6, NaClO4, NaCF3SO3, etc. At present, Na-S batteries mainly use organic electrolytes, but they also bring safety risks, including the Na dendrite problem and the complex side reactions in the cathode, prompting the generation of NaPSs. The produced NaPSs will reach the anode with the help of the electrolyte, resulting in the loss of sulfur. Therefore, researchers have carried out a lot of research on electrolytes in order to promote the commercialization of Na-S batteries [Citation94–96].
5.1.1. Ester-based electrolytes
Conventional ester electrolyte solvents are EC, PC, diethyl carbonate (DEC) and dimethyl carbonate (DMC) to name a few (Figure ). Commonly used sodium salts are NaPF6, NaClO4, NaCF3SO3, trifluoromethylsulfoxide amine (NaTFSI) etc. And then, NaNO3 and FEC are used as electrolyte additives to effectively improve the cycle stability of Na-S batteries and build a more excellent SEI film.
Figure 10. Schematic summary of commonly used sodium salts, solvents and additives for carbonate electrolytes of Na-S batteries.
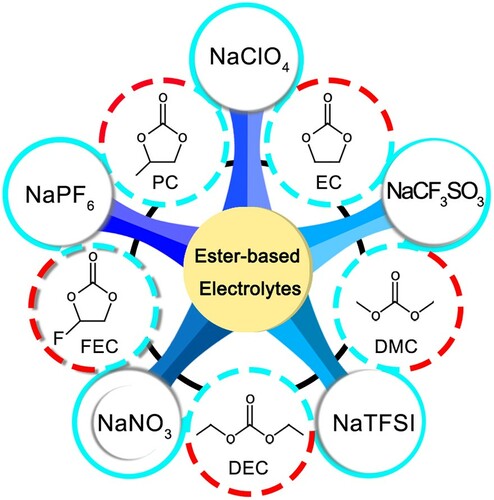
These ester electrolyte solvents, such as EC, PC and DEC, are also known as carbonate-based electrolytes as they are all carbonate-based compounds. These ester-based solvents have been demonstrated to have good cycling performance in Na-ion batteries and ability to work at high pressures. In addition, the solubility of NaPSs in ester electrolytes is lower than that in ether electrolytes, so the shuttle effect of polysulfides can be weakened to a certain extent, and excellent long-cycle stability can be obtained. However, different solvents have different characteristics and can be selected to meet the needs of batteries under different conditions [Citation97]. For example, cyclic carbonates (EC, PC) have high dielectric constants and relatively high ionic conductivity. And then, they can be partially decomposed during the discharge process and participate in building a stable SEI film on the surface of the Na anode. Nevertheless, due to the high melting point of EC and the problem of the low decomposition voltage of PC, EC and PC are usually prepared into a composite carbonate solvent according to a certain ratio. Besides, some chain carbonates are used as solvents, such as DEC and DMC. The viscosity of chain carbonate solvents is lower than that of cyclic solvents, and the electrochemical performance is relatively stable. And the melting point is low, making it suitable for use in batteries at low temperatures [Citation98–101]. Typically, different solvents are formulated for optimum performance. For example, Ma used 1 M of NaClO4 in a mixture of EC and DEC (1:1 v/v) when validating his cathode material for Na-S batteries, and achieved excellent performance [Citation85].
Researches have shown that using FEC as an electrolyte additive is helpful to construct more excellent SEI films. Zhao et al. also studied that the addition of 5 wt% FEC to an electrolyte of 1 M NaClO4-EC/PC produced a superior SEI film and led to a more complete conversion between S and Na2S [Citation102]. The results show that the partial decomposition of FEC mainly produces organic fluorocarbons and inorganic compounds, such as NaF and Na2O, promoting the conversion of S to Na2S. When a C/S complex with a higher specific surface area (YP50F/S) was used as the cathode material, the Na-S battery was found to have a specific capacity as high as 1651 mAh g−1 at 0.1C. Furthermore, the addition of FEC has led to the generation of a NaF-SEI layer, which provides effective inhibition of Na dendrites and exhibits excellent electrochemical and safety performance in long cycles (Figure a-b).
Figure 11. (a), (b) FEC additive working mechanism diagram. Copyright 2018, Elsevier [Citation102]. Combustion tests of (a) 2 M NaTFSI/EC/DEC (1:1, v/v) and (b) 2 M NaTFSI/TMP/FEC electrolytes. Copyright 2019, Elsevier [Citation103].
![Figure 11. (a), (b) FEC additive working mechanism diagram. Copyright 2018, Elsevier [Citation102]. Combustion tests of (a) 2 M NaTFSI/EC/DEC (1:1, v/v) and (b) 2 M NaTFSI/TMP/FEC electrolytes. Copyright 2019, Elsevier [Citation103].](/cms/asset/37276035-4ab8-455b-8278-70243556e71a/tmrl_a_2092428_f0011_oc.jpg)
Generally, ester electrolytes can effectively solve many problems of Na-S batteries and improve electrochemical performance. However, ester electrolytes are highly flammable and volatile, posing certain safety risks. If they are to be commercialized, their safety problem needs to be further addressed. Nowadays, researchers have found that adding certain additives can overcome their shortcomings. For example, flame retardants are added to ester electrolytes to suppress flammability problems. These flame retardants, which include organophosphates and fluorinated phosphates, can use chemical properties to achieve flame retardancy by scavenging reactive hydroxide radicals to delay the progress of combustion chain reactions. Among the flame-retardant additives, trimethyl phosphate (TMP) has received much attention due to its excellent properties, with a low boiling point, high dielectric constant, high phosphorus content and low viscosity. This improves the thermal stability, while at the same time shifting the position of the exothermic peak to the right. Compared to electrolytes without TMP, the capacity released by the cell remains essentially unchanged. It can be concluded that the moderate addition of TMP does not affect the chemical performance of the battery and provides a flame retardant effect to the electrolyte. Wu et al. prepared Na-S batteries electrolyte consisting of TMP, NaTFSI and FEC, combining the properties of the above materials to make the electrolyte non-flammable and safe [Citation103]. The formation of NaF-rich solid electrolyte interfacial films was also revealed by molecular dynamics and surface analysis. Besides, the conventional EC/PC electrolyte was more likely to catch fire, while the electrolyte with TMP did not show the corresponding situation. This intuitively indicates that the electrolyte with the addition of 15% TMP can reduce the safety risk of the Na-S batteries (Figure c-d). Murugan et al. prepared Sodium bis(perfluoropinacol) borate, NaB[O2C2(CF3)4]2 (Na-PPB), and prepared it as a 1M Na-PPB-PC/FEC electrolyte for Na-S batteries [Citation104]. With the high-loaded sulfur cathode and the above electrolyte, the Na-S batteries achieve extremely high electrochemical performance and long-cycle stability.
5.1.2. Ether-based electrolytes
The commonly used solvents for ether electrolytes are monoethylene glycol dimethyl ether (MGDE), diethylene glycol dimethyl ether (DGDE), triethylene glycol dimethyl ether (TREGDME) and TEGDME. Compared with the ester electrolyte, the ether electrolyte can fully dissolve the NaPSs, which helps the reaction to proceed fully, and the liquid phase reaction proceeds faster than the solid phase reaction, which contributes to the battery capacity release. This has been demonstrated in researches of Li-S batteries and is gradually being extended to Na-S batteries researches, where some results have been obtained [Citation105–108]. Initially, Ryu et al. dissolved 1 M NaCF3SO3 in TEGDME and measured an ionic conductivity of 3.9×10−3 S cm−1 [Citation109]. Also, in a Na-S battery with an unmodified S cathode, the first-cycle discharge capacity was 538 mAh g−1, lower than that of an HT-Na/S battery in the same situation. And its long cycle performance is also not satisfactory. The end products of the reaction were detected as Na2S3 and Na2S2. Moreover, it was also found that S and NaPSs were relatively more soluble in the ether electrode solution, and that during the charge/discharge cycle, more and more of the dissolved SX2- shuttled to the Na anode side, leading to a gradual reduction in the active mass of the S cathode, resulting in a gradual reduction in the subsequent charge/discharge capacity [Citation110]. Ryu et al. successfully verified the possibility of the application of ether electrolytes in Na-S batteries, and provided a reference for subsequent research, drawing more attention on using ether electrolytes as the research object of Na-S batteries. Seh et al. found that electrolytes made by dissolving NaPF6 in ether-based solvents (MGDE, DGDE, TEGDME) could form superior SEI films on the surface of the Na anode [Citation111]. It was found that the ether electrolyte facilitates inducing the production of Na2O and NaF, resulting in the production of a more uniform, dense, and thin SEI film. Wu et al. used 2,2,2-trifluoro-N, N-dimethylacetamide (FDMA) solvent, 1,1,2,2-tetrafluoroethyl methyl ether (MTFE) anti-solvent and FEC as Na-S batteries electrolyte [Citation112]. The electrolyte played a role in inhibiting the shuttling of polysulfides. In addition, the electrolyte reacts on the surface of the Na anode to generate an anode protection layer rich in NaF and Na3N to protect the anode, during the discharge process.
Since most electrolyte modifications are aimed at solving the shuttle problem of polysulfides or solving the problem of Na anode dendrites, they are less discussed in this chapter. The research data on Na-S batteries in recent years are integrated and prepared into Table , which is convenient to understand the influence of various electrolytes on Na-S battery performance [Citation29,Citation37,Citation54,Citation65,Citation86,Citation87,Citation112–118].
Table 1. A summary of cathode, liquid electrolytes, and performance for RT-Na/S batteries.
5.2. Solid-based electrolytes
The earliest HT-Na/S batteries used a solid electrolyte. By being heated to a certain temperature, the electrode material and the electrolyte are in a molten state, and the redox reaction can be carried out. Moreover, a major advantage of using solid-state electrolytes is that dendrite growth can be avoided and the problem of polysulfide shuttle can be solved [Citation119,Citation120]. Therefore, with this huge advantage, researchers tried to introduce solid electrolytes into the RT-Na/S batteries system. RT-Na/S batteries based on solid electrolytes have high safety and can avoid the dendrite and polysulfide shuttle problems in organic electrolyte systems. However, compared to the liquid system for RT-Na/S batteries and the molten solid electrolyte system for HT-Na/S batteries, the problem with the solid electrolyte for RT-Na/S batteries is that it has a poorer affinity with the electrode material. Further, the poor contact between the electrode and the electrolyte will lead to an increase in the internal resistance, resulting in a decrease in the capacity of the Na-S batteries [Citation121–125]. A NASICON-type Na+ conductive solid electrolyte (Na3Zr2Si2PO12) was developed and a polymer of intrinsic nanoporosity (PIN) was coated on its surface for application in Na-S batteries. The solid electrolyte Na3Zr2Si2PO12 was detected by XRD and scanning electron microscopy and showed a symbolic NASICON dense structure. It has a Na ionic conductivity of 1.0×10−3 S cm−1 at room temperature and stable cycling and good capacity retention over 100 cycles [Citation126,Citation127].
In the electrolyte section, both solid and liquid electrolytes are inherently designed to provide ion transport pathways for the electrochemical reactions within the battery. The advantage of solid electrolyte is that it can suppress the growth of dendrites and the shuttle of polysulfides. However, the low conductivity of the Na-S batteries and the poor contact between the electrodes and the electrolyte are also disadvantages, which led to the development of quasi-solid gel electrolytes to solve this problem. Similarly, liquid electrolytes have the advantage of good ion transport paths and good intermaterial wettability. However, Na dendrite and polysulfide shuttle effect are inherent problems in liquid electrolyte-based Na-S batteries Figure .
In summary, the electrolyte acts as a medium for ion transport inside the battery, avoiding the transport of electrons. And based on the electrolytes of different systems, the working principles of the batteries are different, and they have their advantages and disadvantages. Firstly, the Na-S batteries based on solid electrolytes can effectively avoid the problem of dendrite growth through physical methods, which improves the safety of the battery. At the same time, due to the pure solid-phase reaction, the dissolution of NaPSs is avoided, and the performance of long-cycle stability is improved. However, the wettability between the electrode and the electrolyte is poor, there is a risk of poor contact. The internal resistance is easily increased, and the electrical conductivity is also reduced. Secondly, in order to release the performance of the Na-S batteries, researchers introduced a liquid-phase electrolyte, so that the Na and S were first converted into the intermediate product NaPSs. And then, the NaPSs is converted to Na2S for better capacity release. Moreover, the electrolyte can fully infiltrate the electrodes, so that the contact resistance between the two is reduced, and the performance of the battery is improved. Among the liquid-phase electrolytes, ether electrolyte can fully dissolve the NaPSs, so that the reaction of the battery is transformed from solid–solid to solid–liquid-solid phase reaction. In the liquid phase system, the conversion rate of NaPSs is improved, but the shuttle effect of polysulfide is inevitable. While in ester electrolytes, the solubility of NaPSs is relatively low. Although the conversion rate of NaPSs is reduced, the shuttle effect of polysulfide is effectively suppressed. And based on the slow conversion of NaPSs in ester electrolytes, the conversion of NaPSs can be accelerated by introducing a catalyst into the electrode material. In addition, the organic electrolyte also has the characteristics of low flash point and flammability. The existing solution is to add appropriate additives to improve its safety and stability.
6. Design of modified separator
If the electrolyte is the bridge that communicates the positive and negative electrodes, then the separator can be used as a checkpoint on this bridge. Besides, the function of the separator is to prevent the positive and negative electrodes from being in direct contact, and only allow the ions in the electrolyte to pass through. At the same time, there are abundant pores on the separator, and the existence of these pores itself provides channels for ion transport. However, these channels also provide convenience for the shuttle of polysulfides, so that NaPSs can have a shuttle effect through pores. Therefore, researchers tried to modify the separator to block the shuttle of polysulfides and accelerate the conversion of NaPSs (Figure ) [Citation128–130].
Figure 13. Schematic diagram of the modified separator to inhibit polysulfide shuttle effect in Na-S batteries.
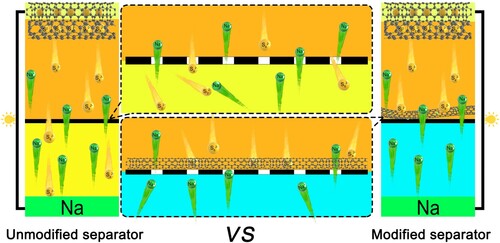
Due to the higher reactivity of Na, the dendrite problem is exacerbated. Moreover, the diameter of Na+ is larger, so larger channels are required to facilitate the migration of Na+. Therefore, glass fibers are generally used as separators in Na+ batteries to alleviate the above problems. Compared with the conventional polypropylene (PP) separator, the glass fiber separator has a larger porosity and higher wettability of the electrolyte. Larger pores facilitate ion migration, but they also induce more severe polysulfide shuttle effects. Therefore, modifying the glass fiber separator to inhibit the shuttle effect of free NaPSs is also one of the strategies to improve the cycle life of Na-S batteries.
Yang et al. wrapped polar MoS2 on hollow carbon spheres (HCS/MoS2) [Citation131]. The HCS/MoS2 were used as a cathode sulfur storage material for Na-S batteries and coated on glass fiber separators. And then, the improvement of electronic conductivity is achieved with the HCS at the positive electrode. The MoS2 on the outer layer of the HCS inhibits the escape of NaPSs at the positive electrode and catalyzes the conversion of NaPSs (Figure a). More importantly, the second line of defense was set up at the separator. Molybdenum disulfide-coated carbon spheres were used to capture and catalytically convert free polysulfides. Therefore, the versatile design improves the utilization of sulfur, improves the cycle stability, and obtains relatively excellent electrochemical performance. At a current of 1 C, a high initial specific capacity (1090 mAh g−1) was obtained for the Na-S batteries with the HCS/MoS2 modified cathode and separator.
Figure 14. (a) Schematic diagram of HSC/MoS2 modified separator. Copyright 2019, WILEY-VCH [Citation131]. (b) Schematic diagram of the synthesis of the FPNs-G/separator. Copyright 2020, WILEY-VCH [Citation132]. (c) Working mechanism diagram of the modified separator. (d) Electrochemical performance graph. Copyright 2021, Elsevier [Citation133]. (e) Schematic diagram of the synthesis of MXene@C/PP/MXene@C separator. Copyright 2022, Wiley-VCH [Citation134].
![Figure 14. (a) Schematic diagram of HSC/MoS2 modified separator. Copyright 2019, WILEY-VCH [Citation131]. (b) Schematic diagram of the synthesis of the FPNs-G/separator. Copyright 2020, WILEY-VCH [Citation132]. (c) Working mechanism diagram of the modified separator. (d) Electrochemical performance graph. Copyright 2021, Elsevier [Citation133]. (e) Schematic diagram of the synthesis of MXene@C/PP/MXene@C separator. Copyright 2022, Wiley-VCH [Citation134].](/cms/asset/993dfbfb-54a7-4c10-b21e-4ad03299e054/tmrl_a_2092428_f0014_oc.jpg)
Li et al. used the self-assembly of Fe-ion and polyacrylamide in a solvent to obtain highly uniformly distributed Fe-ion composite polyacrylamide nanospheres (FPNs), which were mixed with rGO and then coated on glass fiber separators (FPNs-G/separator) (Figure b) [Citation132]. With the help of iron-composite carbon microspheres, the adsorption and catalytic ability of NaPSs can be effectively improved. The overall mechanical strength of the separator is improved with the strong physical properties of rGO. Meanwhile, rGO can be used to adsorb NaPSs in the solvent more quickly and transfer them to Fe-ion for confinement and catalysis. Subsequently, the FPNs were carbonized at high temperature and etched to obtain mesoporous polyacrylamide nano-microspheres (PNC-Ns), which were used for sulfur storage in the positive electrode of Na-S batteries. The introduction of PNC-Ns enables sulfur to be trapped and fast electron to transport through the carbon shell. In general, through the design of the modified cathode and the modified separator, the electrochemical reaction of sulfur is effectively improved. Therefore, at a current density of 0.1 C, the Na-S battery still retains a high specific capacity of 639 mAh g−1 after 400 cycles.
In addition to the direct coating on glass fiber separators for Na-S batteries, the performance of Na-S batteries can be improved by designing a self-supporting material to act as an interlayer. Saroha et al. obtained a self-supporting carbon fiber substrate (CNFs) by electrospinning first and then high-temperature carbonization [Citation133]. And then the V2O3 composite CNFs self-supporting interlayer was synthesized by hydrothermal method (V2O3@CNFs) (Figure c). It was then placed as an interlayer between the battery's separator and the sulfur cathode. Among them, the CNFs obtained by electrospinning provides a physical barrier to inhibit the shuttle of polysulfides. Moreover, CNFs provide a substrate for the attachment of V2O3 nanoparticles, so that the two can work synergistically. And then, V2O3 can not only adsorb NaPSs but also realize the catalytic conversion of NaPSs. Therefore, the V2O3@CNFs-based Na-S batteries can also obtain strong electrochemical stability without modifying the sulfur cathode or separator. At a current density of 1C, the V2O3@CNFs-based Na-S battery has good cycling stability and a reversible specific capacity of 406 mAh g−1 after 500 cycles. The V2O3@CNFs-based Na-S battery has a decay rate of only 0.076% after 1000 cycles at 2C (Figure d).
Considering the large porosity of glass fibers, researchers tried to modify the PP separator to improve the cycle stability of Na-S batteries. Wang et al. synthesized carbon-wrapped MXene nanosheets with a core–shell structure for modifying both sides of the PP separator (MXene@C/PP/MXene@C) (Figure e) [Citation134]. Moreover, the MXene-based separator solves the two major problems of Na-S batteries: (1) Suppressing the escape of NaPSs in the electrolyte on the S cathode side; (2) Inducing uniform deposition of Na+ on the Na anode. In addition, the nanosheet structure of MXene increases the escape difficulty of free NaPSs, so that the NaPSs dissolved in the electrolyte are effectively adsorbed on the nanosheets and undergo catalytic conversion. Therefore, the Na-S battery exhibits an initial specific capacity of1159 mAh g−1 at 0.2 C. And after 650 cycles at 0.5 C, the capacity of the Na-S battery remained at 95.8%.
Based on the above research results, modifying the separator or introducing an interlayer is one of the effective methods to improve the performance of Na-S batteries. Although the current research results are few, it is not difficult to find that the wettability of the electrolyte can be effectively improved by adding carbon materials. And carbon materials can also capture free NaPSs to suppress the shuttle effect of polysulfides. Meanwhile, adding metal compounds can provide more powerful polar adsorption and realize the catalytic conversion of NaPSs, so as to solve the problem of slow NaPSs conversion. In the existing design, there is also a scheme of using a material to modify the cathode and the separator at the same time, which also greatly improves the performance of the Na-S batteries. Therefore, if multiple lines of defense can be set up in metal-sulfur batteries, it can increase the difficulty of the shuttle of polysulfides, further effectively inhibit the shuttle of polysulfides and improve long-term cycle stability (Figure ). In addition, modification of the anode side of the separator can also induce uniform metal deposition to suppress dendrite growth. Moreover, the modified layer helps to enhance the mechanical properties of the separator, so that the separator is not easily pierced by dendrites. Therefore, modifying the separator is a relatively simple and effective way to solve the problems of Na-S batteries.
7. Strategies for safe sodium metal anodes
Due to the strong activity, Na metal is easy to spontaneously react with the electrolyte, and the electrolyte is consumed to form an SEI film and loses capacity. Besides, due to the soft texture of Na, metal cracking is more likely to occur, which exposes more fresh Na metal and aggravates the consumption of electrolytes. And this will also bring about uneven deposition of Na, leading to more serious dendrites. Moreover, the deposition of NaPSs on the surface of Na metal caused by the shuttle effect of polysulfides will lead to corrosion of Na anode in Na-S batteries, which further reduces the performance of the Na-S batteries. The problems above reduce cycling performance of Na-S batteries and even lead to serious safety problems. Therefore, the stability of the Na anode is the key to the practical application of Na-S batteries [Citation135–139]. Various approaches have been proposed to solve these problems: (1) Selecting appropriate electrolyte and related additives to form a stable SEI protective layer on the surface of the Na anode; (2) Through pretreatment, additional chemical or physical modification is performed on the surface of the Na anode to form a stable artificial SEI protective layer; (3) Constructing a 3D electrode structure that promotes the uniform deposition and conversion into Na metal of Na-ions to suppress the growth of Na dendrites (Figure ) [Citation140–143].
7.1. Electrolyte composition
7.1.1. Liquid electrolyte engineering
Na-based batteries have different performances in the ester and ether solvents. Recent researches have shown that Na-based batteries exhibit better performance in ether-based electrolytes. As mentioned above, Seh et al. found that NaPF6 can provide effective protection to the anode in ether electrolytes [Citation111].
The concentration of the electrolyte salt will also significantly affect the electrochemical performance of the liquid electrolyte and affect the growth of dendrites. The increase in the concentration of electrolyte salt will form a certain solvation structure in the electrolyte, which will reduce the amount of free solvent in the electrolyte and restrain the side reaction between the organic solvent and Na [Citation144–149]. Lee et al. proposed a high-concentration electrolyte (HCE), composing of 5 M sodium bis (fluorosulfonyl) imide in 1,2-dimethoxyethane [Citation150]. With this electrolyte in the Na/stainless steel (SS) battery, when Na plating/stripping in the 120th cycle, a high coulomb efficiency of 99.3% can still be achieved, and the corrosion of Na metal is also greatly reduced.
Although HCE has the advantage of improving Na anode to a certain extent, it also has disadvantages such as high viscosity and poor wettability. In order to make up for the shortcomings above, a method of adding ‘inert’ solvents to HCE to form a localized high concentration electrolyte (LHCE) has recently been developed. ‘Inert’ solvents with low donor number and low dielectric constant have little effect on the original solvent structure of HCE. For example, Zheng et al. maintained the solvation structure of HCE by using hydrofluoroether as an ‘inert’ diluent, thereby forming a LHCE [2.1 M sodium bis (fluorosulfonyl) imide (NaFSI)/1,2-dimethoxyethane (DME)-bis (2,2,2-trifluoroethyl) ether (BTFE) (solvent molar ratio 1:2)] [Citation151]. The LHCE can achieve fast charging (20 C) and stable Na || Na3V2 (PO4) 3 battery cycles (90.8% retained after 40,000 cycles) (Figure a-b).
Figure 17. (a) Schematic illustration of dilution from an HCE to an LHCE. (b) Cycling performance of Na||NVP batteries at 20C after three formation cycles at C/10.Copyright 2018, American Chemical Society [Citation151]. (c) Simulated electric-field intensity distribution and schematic illustration of Li/Na plating patterns. Copyright 2018, Wiley-VCH [Citation152]. (d) A good wetting ability artificial interlayer during the plating of Na between the solid-state electrolyte and Na metal anode. Copyright 2017, American Chemical Society [Citation153].
![Figure 17. (a) Schematic illustration of dilution from an HCE to an LHCE. (b) Cycling performance of Na||NVP batteries at 20C after three formation cycles at C/10.Copyright 2018, American Chemical Society [Citation151]. (c) Simulated electric-field intensity distribution and schematic illustration of Li/Na plating patterns. Copyright 2018, Wiley-VCH [Citation152]. (d) A good wetting ability artificial interlayer during the plating of Na between the solid-state electrolyte and Na metal anode. Copyright 2017, American Chemical Society [Citation153].](/cms/asset/b2898c60-996b-4064-b393-798754075f91/tmrl_a_2092428_f0017_oc.jpg)
As mentioned in the electrolyte section above, appropriate additives are selected to significantly improve the SEI performance of the Na anode and increase its coulombic efficiency. Recently, people have conducted in-depth research on fluorine-containing compounds as additives in electrolytes. With these additives, the anode can be effectively protected by the formation of SEI film [Citation150–156]. For example, Lee and his colleagues used an FEC-based electrolyte with 1 M NaFSI, thus proving that the FEC-based electrolyte can effectively inhibit Na dendrites [Citation157]. This method can avoid dendritic Na deposition and construct a uniform ion intermediate layer to achieve a highly reversible Na plating/stripping reaction. Ding et al. demonstrated a novel mechanism that can fundamentally change the formation of dendrites, where additives replace the formation of a positively charged electrostatic shielding layer around the initial growth tip during metal deposition [Citation158]. For example, Zhang and his colleagues fabricated Li-Na hybrid electrolytes containing LiPF6, NaPF6 and DME. Li+ forms a positively charged electrostatic shielding layer, which inhibits dendrite growth by diffusing Na+ through the electrostatic shielding effect (Figure c) [Citation152]. Shi et al. found that the addition of bis (trifluoromethylsulfonyl) imide (KTFSI) provided a cationic shielding effect by K+ to inhibit dendrite growth, and TFSI- helps to form the N-containing SEI layer [Citation159].
7.1.2. Solid-state electrolyte engineering
Solid-state electrolyte (SE) materials of Na-based batteries are mainly divided into organic polymer electrolytes and inorganic solid electrolytes. The organic polymer electrolyte is composed of a polymer and Na salt, which has good flexibility and can adapt to changes in material volume during the reaction. But its ion conductivity and transfer number are low at room temperature. Ma et al. proposed a solid polymer electrolyte, composed of Na (fluorosulfonyl) (n-nonafluorobutanesulfonyl) imide (Na[(FSO2)(n-C4F9SO2)N], NaFNFSI) and poly(ethylene oxide) (PEO) [Citation160]. The ionic conductivity of the organic polymer electrolytes can be greatly improved, and the ionic conductivity of the mixed polymer electrolyte can reach 3.36×10−4 S cm−1 at 80 °C. In addition, inorganic solid electrolytes also possess high conductivity along with high stability. Na-β″-Al2O3, NASICON and sulfide-based systems have been extensively researched due to their high conductivity and good mechanical properties. Na-β″-Al2O3 is the most famous SE material, and Na+ conductivity can reach 7.80 mS cm−1 [Citation161].
In addition to the above SE materials, there are also composite hydrides, which show very high ionic conductivity. For example, Na2(B12H12)0.5(B10H10)0.5, the ionic conductivity can reach to 0.9 S cm−1 at room temperature [Citation153]. At the same time, recently, a thin interfacial interlayer was introduced between metallic-sodium anode and NASICON solid electrolyte, which enables highly reversible plating/stripping of a dendrite-free. The soft polymer layer limits the side reactions between the electrolyte and the Na metal, and suppresses the volume change of the Na anode (Figure d).
7.2. Sodium metal anode/electrolyte interface engineering
Modification of the anode surface by adding an artificial protective layer can effectively protect the anode material and inhibit dendritic problems during reactions. It is a common strategy to keep the Na metal anode stable. NaX (X = F, Br, I) and Al2O3 are commonly used as inorganic coatings. Luo et al. deposited a thin layer of Al2O3 artificial SEI on the surface of the Na metal anode, via a low-temperature plasma-enhanced atomic layer deposition (PEALD) treatment, which can well prevent Na metal from reacting with the electrolyte (Figure a) [Citation162]. As shown in Figure b, the battery using Na metal electrode with Al2O3 coated has been cycled for more than 450 h (900 cycles) at a constant current in a smaller voltage range. At the same time, Wang et al. prepared an inorganic–organic MLD-alucone on the Na metal anode by molecular layer deposition (MLD) (similar to ALD) [Citation163]. The curve with longer lifetime and stable polarization demonstrated that alucone-coated Na is better than ALD Al2O3. As shown in Figure c-d, for Na@25alucone, an almost flat voltage plateau is observed in both charging and discharging conditions, and it remains constant throughout the whole cycle life. However, after 160 and 270 h, the bare Na showed a significant increase and unstable overcharge potential. Zhao et al. directly coated a free-standing GO film with and adjustable thickness on the surface of Na metal, where the number of GO layers can be easily adjusted by controlling the experimental parameters of the chemical vapor deposition (CVD) system [Citation164]. A symmetric battery with Na metal electrode, which was coated by a multilayer GO film as a protective layer, shows high cycle stability within 300 h, and the current density was as high as 2 mA cm−2 and cycling capacity up to 3 mAh cm−2 (Figure e-f).
Figure 18. (a) Schematic illustration of the stabilization of the sodium metal anode by PEALD Al2O3 coating; (b) Electrochemical performance graph. Copyright 2016, WILEY-VCH [Citation162]. (c) Schematic diagram showing the GO film as a protective layer for Na anode. (d) Cycling performance of the symmetric cells made from ML/G-Na electrodes (red) and bare Na electrodes (blue). Copyright 2017, American Chemical Society [Citation163]. (e) Schematic diagrams of sodium stripping/plating on Na foil with MLD alucone coating; (f) Comparison of the cycling stability of the Na@25 alucone and the bare Na foil. Copyright 2017, American Chemical Society [Citation164]. (g) Preparation of NaOH and NaNH2 on Na metal surface by steam method. Reproduced with permission from. Copyright 2020, Elsevier [Citation165]. (h) Synthesis of MAI films on Na metal surfaces. Copyright 2020, Elsevier [Citation166].
![Figure 18. (a) Schematic illustration of the stabilization of the sodium metal anode by PEALD Al2O3 coating; (b) Electrochemical performance graph. Copyright 2016, WILEY-VCH [Citation162]. (c) Schematic diagram showing the GO film as a protective layer for Na anode. (d) Cycling performance of the symmetric cells made from ML/G-Na electrodes (red) and bare Na electrodes (blue). Copyright 2017, American Chemical Society [Citation163]. (e) Schematic diagrams of sodium stripping/plating on Na foil with MLD alucone coating; (f) Comparison of the cycling stability of the Na@25 alucone and the bare Na foil. Copyright 2017, American Chemical Society [Citation164]. (g) Preparation of NaOH and NaNH2 on Na metal surface by steam method. Reproduced with permission from. Copyright 2020, Elsevier [Citation165]. (h) Synthesis of MAI films on Na metal surfaces. Copyright 2020, Elsevier [Citation166].](/cms/asset/9f0358f6-11c3-4b0b-8987-981bb9919506/tmrl_a_2092428_f0018_oc.jpg)
Kumar et al. spontaneously grew an artificial SEI film constructed of NaNH2 and NaOH on the surface of Na metal by the vapor flux [Citation165]. Among them, NaOH possesses a high Young's modulus, and NaNH2 possesses a certain toughness (Figure g). After the combination of the two, the artificial SEI film also has the effect of promoting the transport of Na+. Moreover, it not only promotes the uniform electrodeposition of Na+ at the source, but also effectively weakens the growth of Na dendrites. And then, using it in Na-S batteries effectively promotes the long-cycle stability of the batteries. What’s more, the group also used SnCl4 vapor to construct an artificial metal alloy interface (MAI) on the surface of Na metal (Figure h). The MAI interface is composed of sodium-tin alloy (NaxSny) and has a high Young's modulus, which helps to suppress the growth of Na dendrites. And the interface effectively reduces the interface resistance and promotes the ion exchange of the Na anode [Citation166]. In addition to the above methods, Tian et al. used first-principles calculations to determine the key factors of the characteristics and feasibility of various 2D layered protective films (PF), and studied h-BN, GO, silylene, germanene, stanene, phosphorene, SnS, and SnSe as PFs for lithium or Na anode [Citation167,Citation168].
7.3. Nanostructured sodium metal anodes
The structure of the 3D current collector can make the electron transport more uniform and promote the uniform deposition of Na+, thereby suppressing the growth of Na dendrites. Among them, carbon nanostructures have the advantages of light weight, high conductivity, high mechanical strength and large surface area, etc., so that Na metal can be uniformly deposited into the carbon nanostructure without producing metal dendrites [Citation169–171]. It is considered as an ideal 3D current collector for Na metal anodes. For example, Luo et al. produced a stable Na carbonized wood (Na-wood) composite anode by quickly injecting molten Na into the channel of carbonized wood (Figure a) [Citation172]. These channels greatly reduce the effective current density and ensure the uniform nucleation of Na. As shown in Figure b, the Na-wood composite anode shows a small overpotential and a stable cycle plating/stripping curve within 500 h under the condition of 1 mA cm−2. Xu et al. introduced a 3D porous Ni@Cu scaffold with honeycomb-shaped nickel nanoparticles on a copper substrate prepared by a hydrogen bubble dynamic template electrodeposition method (Figure c) [Citation173]. It exhibits excellent reversible Na deposition cycle performance (over 240, 110 and 50 cycles for 0.5, 1.0 and 2.0 mAh cm−2 at 1.0 mA cm−2).
Figure 19. (a) Schematic and corresponding images of two-step synthetic procedures; (b) electrochemical performance of symmetric cells. Copyright 2017, American Chemical Society [Citation172]. (c) A schematic of the Na plating process on a honeycomb-like 3D Ni@Cu and a planar Cu foil. Copyright 2018, Elsevier [Citation173]. (d) Schematic illustration of the metallic Na striping/plating on Na metal anode and Na/NSCNT anode. Copyright 2018, Wiley-VCH [Citation174]. (e) Schematic illustration of the fabrication of Na-MoS2(CR) hybrid. Copyright 2017, American Chemical Society [Citation40].
![Figure 19. (a) Schematic and corresponding images of two-step synthetic procedures; (b) electrochemical performance of symmetric cells. Copyright 2017, American Chemical Society [Citation172]. (c) A schematic of the Na plating process on a honeycomb-like 3D Ni@Cu and a planar Cu foil. Copyright 2018, Elsevier [Citation173]. (d) Schematic illustration of the metallic Na striping/plating on Na metal anode and Na/NSCNT anode. Copyright 2018, Wiley-VCH [Citation174]. (e) Schematic illustration of the fabrication of Na-MoS2(CR) hybrid. Copyright 2017, American Chemical Society [Citation40].](/cms/asset/8aa2746e-ac41-4312-9797-bf36c0aaed4b/tmrl_a_2092428_f0019_oc.jpg)
The 3D current collector can be modified to enhance the ability to protect the Na anode [Citation175–178]. For example, Sun et al. synthesized nitrogen and sulfur co-doped carbon nanotubes (NSCNT) by a simple pyrolysis method and used it as an intermediate layer to control the Na nucleation behavior and inhibit the growth of Na dendrites [Citation174]. Due to the functional groups of N and S on the surface of NSCNT, the produced NSCNT exhibit a high degree of ‘sodiophilicity’, which can guide the initial Na nucleation and evenly distribute Na on the NSCNT paper (Figure d). The research shows that the Na/NSCNT||NSCNT/Na battery runs stable for more than 500 h under 1 mA cm−2, and the capacity is limited to 1 mAh cm−2. Zhang et al. proposed the direct composite of MoS2 2D sheets with Na as an anode [Citation40]. First, MoS2 undergoes a displacement reaction with Na, and an artificial SEI film rich in Na2S is constructed on the surface of Na. Furthermore, the MoS2 sheet also physically inhibits the volume change of Na and weakens the effect of Na dendrites (Figure e).
8. Conclusions and perspectives
In this work, starting from the background of RT-Na/S batteries, we review the recent research achievements of RT-Na/S batteries and emphasize the necessity of RT-Na/S batteries research. Firstly, this review takes the working principle of the RT-Na/S battery as an entry point and conducts a more in-depth discuss on the RT-Na/S battery through specific analysis. Secondly, the existing research results of RT-Na/S batteries are divided into four aspects: sulfur cathode modification, electrolyte optimization, separator modification and Na anode protection. Through the research results in the directions above, case research is carried out to analyze how to inhibit the shuttle of polysulfides, accelerate the transformation of polysulfides and protect the metal anode. In addition, the sulfur cathode, electrolyte, separator and Na anode are the basic components of the RT-Na/S batteries. Therefore, analyzing them can address the problems of the RT-Na/S batteries more comprehensively and effectively and obtain the best method to improve the performance of RT-Na/S batteries.
By learning from the existing research results of Li-S batteries, the sulfur cathode of the original RT-Na/S batteries used 3D carbon materials as the sulfur host. The sulfur is uniformly dispersed through the carbon material, and the sulfur can perform fast electron transport through the carbon skeleton, thereby increasing the reaction rate. Furthermore, the carbon material also contributes to the full infiltration of the electrolyte, which is conducive to the rapid transport of ions. And by regulating the pores of the carbon material, the shuttle of polysulfides can be effectively restricted, so that the conversion of sulfur is controlled inside the 3D carbon skeleton. However, the adsorption and confinement capabilities of carbon materials are relatively limited. Therefore, in the follow-up research, the introduction of heteroatoms for recombination improved the adsorption and catalytic conversion capabilities of NaPSs and provided new ideas for the research of RT-Na/S batteries. Doping metal compounds such as metal oxides, sulfides, single atoms, etc. can be more effective in confining NaPSs and promoting the conversion of NaPSs. And through analysis, it can be seen that carbon materials are used to solve the electronic conductivity defect of the sulfur cathode and achieved results. The introduction of metal compounds and heteroatoms provides stronger polar adsorption, weakens the shuttle effect of polysulfides and improves the long-cycle stability of the RT-Na/S batteries. At the same time, some metal compounds also have a catalytic effect on NaPSs, which promotes its conversion reaction. Therefore, it is foreseeable that by adding composite materials with high electrical conductivity, abundant pores, polar adsorption and catalytic ability, the RT-Na/S batteries obstacles of low electrical conductivity, polysulfide shuttle and slow reaction kinetics can be overcame from multiple angles.
The electrolyte is the medium of positive and negative reactions, which isolates electron transport and provides a channel for ion transport. And the working mechanisms of RT-Na/S batteries based on different electrolyte systems are different. Therefore, the research of high-efficiency, low-cost, and high-stability electrolytes is also a hot research topic at present. Electrolytes can be roughly divided into solid electrolytes and liquid electrolytes. Due to the avoidance of dendrites and polysulfide shuttle problems and high chemical stability, solid electrolytes have always been a popular research direction. However, this type of electrolyte also has defects such as low ionic conductivity, high interfacial impedance, and poor interfacial compatibility. In contrast, liquid electrolytes are the current mainstream research direction, and with the help of liquid electrolytes, the reaction of Na-S batteries can introduce NaPSs as an intermediate product, so that the capacity can be released efficiently. The liquid electrolyte includes ester electrolyte and ether electrolyte. The ether-based electrolytes have excellent electrochemical performance and are relatively more stable in organic systems. And after the addition of NaPF6, a thinner, denser and stronger SEI film is formed on the Na metal surface. In addition, the reaction involves a solid–liquid-solid phase transition due to the high solubility of NaPSs in ether electrolytes. The reaction in the liquid phase can be more complete and faster, but also brings about a polysulfide shuttle effect. Therefore, the research on ether electrolytes should focus more on how to inhibit the shuttle of NaPSs through modification. Besides, the solubility of NaPSs in ester electrolytes is relatively low, and the reaction is transformed into solid–solid phase transformation, which weakens the shuttle effect of polysulfides. In recent years, there have also been breakthroughs in research based on ester electrolytes, such as using TMP as a flame retardant to improve the thermal stability of batteries. For example, adding FEC as an electrolyte additive promotes the formation of SEI film on the surface of Na metal, making its performance more excellent. Therefore, liquid electrolytes can effectively improve the electrochemical performance of RT-Na/S batteries, but how to improve the long-cycle stability is an essential research direction. The research results above show that solid electrolytes and liquid electrolytes have their advantages and disadvantages. Therefore, perhaps we can change our train of thought and research quasi-solid electrolytes, which have the advantages of both solid and liquid electrolytes. Quasi-solid electrolytes can weaken the problems existing in the solid electrolyte and liquid electrolyte systems, further improve the utilization rate of sulfur, and ultimately lead to further development of the RT-Na/S batteries.
The separator is used to isolate the cathode and anode to avoid their contact reaction and self-discharge. Meanwhile, because the separator needs to play the role of conducting ions, it has abundant pores, but this also provides a convenient channel for the shuttle of polysulfides. From the initial carbon materials used for RT-Na/S batteries separator modification, some success has been achieved. Then, referring to the method of modifying the sulfur cathode, the metal compound composite carbon material was used to modify the RT-Na/S batteries separator, and the electrochemical stability is greatly improved. Researchers tried to use materials with polar adsorption and catalytic effect on NaPSs at the same time for sulfur host and modification of the separator, setting the first line of defense on the anode to inhibit the dissolution of NaPSs. At the same time, modification on the membrane prevents the shuttle of polysulfide by establishing a second line of defense. Moreover, in order to solve the problem of Na dendrites in the anode at the same time, researchers also tried to modify the separator near the metal side to induce uniform deposition of Na+, and the dendrites problem has also been effectively suppressed. Therefore, the future research directions of separator modification for RT-Na/S batteries should be diversified. For example, increasing the wettability between the separator and the electrolyte by coating carbon materials to reduces the interface resistance and adsorbs polysulfides in the electrolyte. Besides, on the basis of carbon materials, polar adsorbents are supported to capture NaPSs, and catalysts are added to accelerate NaPSs conversion. And then, a suitable material can be modified on the surface of the separator near the metal side to suppress the growth of dendrites. Through the optimization of the above scheme, the problems of polysulfide shuttle, slow reaction kinetics and Na dendrite growth can be solved by minor modifications on the separator in the future.
In order to ensure the long-term stability of RT-Na/S batteries, not only the shuttle problem of polysulfides but also the growth of Na dendrites need to be addressed. Besides the above-mentioned modification on the Na metal side of the separator to induce the uniform deposition of Na+, the growth of Na dendrites can also be suppressed by constructing an artificial SEI film and using a 3D current collector. Firstly, due to the strong metallicity of Na, the problem of Na dendrites is more serious. Besides, Na is softer and breaks more easily, which allows more fresh sodium to react with the electrolyte. Therefore, directly modifying the anode surface to construct artificial SEI membranes is a simple and effective strategy. The growth of Na dendrites is suppressed by introducing materials with metal, which has electrolyte affinity and toughness, and the Na metal surface is protected from polysulfide contamination. Or by optimizing the composition of the electrolyte, an SEI film with good physical and chemical stability will be formed on the anode surface to protect the Na metal anode. Secondly, 3D current collectors as the carrier of Na metal and conductive networks to promote uniform electron transport are also effective ways to inhibit dendrite growth. It should be notably considered that, when optimizing the electrolyte, forming an excellent SEI film at the anode to suppress sodium dendrites and the inhibition of polysulfide shuttle in RT-Na/S batteries simultaneously. Besides, there are more and more researches on the use of biomass materials for anode protection of Zn and Li metals recently. Consequently, it is foreseeable to look for inspiration in nature and use biomass materials to build artificial SEI membranes for better RT-Na/S battery performance.
The improvement directions above can effectively improve the cycle stability of RT-Na/S batteries. Therefore, since the development of RT-Na/S batteries, people have carried out in-depth research on a series of problems such as low electronic conductivity of sulfur cathode, severe volume expansion, polysulfide shuttle effect and Na dendrites, and staged progress has been made. Through the modification of the sulfur cathode, the optimization of the electrolyte, the modification of the separator, and the protection of the Na metal anode, many problems existing in the RT-Na/S batteries have been solved. We believe that through the analysis of the above four research directions in this paper, subsequent researchers can better understand the working principle of RT-Na/S batteries and the existing problems, and get ideas for solving the problem. Moreover, we believe that, in the future, RT-Na/S batteries can be brought to the market.
Disclosure statement
No potential conflict of interest was reported by the author(s).
Additional information
Funding
References
- Wang D-W, Zeng Q, Zhou G, et al. Carbon-sulfur composites for Li-S batteries: status and prospects. J Mater Chem A. 2013;1:9382–9394.
- Liang J, Sun Z-H, Li F, et al. Carbon materials for Li-S batteries: functional evolution and performance improvement. Energy Stor Mater. 2016;2:76–106.
- Liang J, Li F, Cheng H-M. On energy: batteries beyond lithium ion. Energy Stor Mater. 2017;7:A1–A3.
- Nikiforidis G, Jongerden GJ, Jongerden EF, et al. An electrochemical study on the cathode of the intermediate temperature tubular sodium-sulfur (NaS) battery. J Electrochem Soc. 2019;166:A135–A142.
- Li T, Xu J, Wang C, et al. The latest advances in the critical factors (positive electrode, electrolytes, separators) for sodium-sulfur battery. J Alloys Compd. 2019;792:797–817.
- Kairies K-P, Figgener J, Haberschusz D, et al. Market and technology development of PV home storage systems in Germany. J Energy Storage. 2019;23:416–424.
- Liu M, Bai W, Guo H, et al. A permselective and multifunctional 3D N-doped carbon nanotubes interlayer for high-performance lithium-sulfur batteries. Electrochim Acta. 2022;421:140430.
- Liu T, Zhang Y, Chen C, et al. Sustainability-inspired cell design for a fully recyclable sodium ion battery. Nat Commun. 2019;10:1965.
- Gross MM, Manthiram A. Development of low-cost sodium-aqueous polysulfide hybrid batteries. Energy Stor Mater. 2019;19:346–351.
- Wang Y, Zhou D, Palomares V, et al. Revitalising sodium-sulfur batteries for non-high-temperature operation: a crucial review. Energy Environ Sci. 2020;13:3848–3879.
- Liu H, Lai WH, Lei Y, et al. Electrolytes/interphases: enabling distinguishable sulfur redox processes in room-temperature sodium-sulfur batteries. Adv Energy Mater. 2022;12:2103304.
- Gope S, Singh DK, Eswaramoorthy M, et al. An extremely high surface area mesoporous-microporous-networked pillared carbon for high stability li-s and intermediate temperature Na-S batteries. Chem Select. 2017;2:9249–9255.
- Delmas C. Sodium and sodium-Ion batteries: 50 years of research. Adv Energy Mater. 2018;8:1703137.
- Ye H, Li Y. Room-temperature metal-sulfur batteries: What can we learn from lithium-sulfur? Info Mat. 2022;4:e12291.
- Ye C, Jin H, Shan J, et al. A Mo5N6 electrocatalyst for efficient Na2S electrodeposition in room-temperature sodium-sulfur batteries. Nat Commun. 2021;12:7195.
- Zhang BW, Sheng T, Wang YX, et al. Long-life room-temperature sodium-sulfur batteries by virtue of transition-metal-nanocluster-sulfur interactions. Angew Chem Int Ed Engl. 2019;58:1484–1488.
- Yan Z, Liang Y, Hua W, et al. Multiregion janus-featured cobalt phosphide-cobalt composite for highly reversible room-temperature sodium-sulfur batteries. ACS Nano. 2020;14:10284–10293.
- Jeon JW, Kim D-M, Lee J, et al. PIM-1-based carbon-sulfur composites for sodium-sulfur batteries that operate without the shuttle effect. J Mater Chem A. 2020;8:3580–3585.
- Zhang S, Pollard TP, Feng X, et al. Altering the electrochemical pathway of sulfur chemistry with oxygen for high energy density and low shuttling in a Na/S battery. ACS Energy Lett. 2020;5:1070–1076.
- Wang N, Wang Y, Bai Z, et al. High-performance room-temperature sodium-sulfur battery enabled by electrocatalytic sodium polysulfides full conversion. Energy Environ Sci. 2020;13:562–570.
- Liang Z, Zheng G, Liu C, et al. Polymer nanofiber-guided uniform lithium deposition for battery electrodes. Nano Lett. 2015;15:2910–2916.
- Sun B, Li P, Zhang J, et al. Dendrite-free sodium-metal anodes for high-energy sodium-metal batteries. Adv Mater. 2018;30:1801334.
- Hong X, Mei J, Wen L, et al. Nonlithium metal-sulfur batteries: steps toward a leap. Adv Mater. 2019;31:1802822.
- Zhang Y, Liu N. Nanostructured electrode materials for high-energy rechargeable Li, Na and Zn batteries. Chem Mater. 2017;29:9589–9604.
- Zhao Y, Adair KR, Sun X. Recent developments and insights into the understanding of Na metal anodes for Na-metal batteries. Energy Environ Sci. 2018;11:2673–2695.
- Li L, Peng S, Lee JKY, et al. Electrospun hollow nanofibers for advanced secondary batteries. Nano Energy. 2017;39:111–139.
- Wang Y-X, Zhang B, Lai W, et al. Room-temperature sodium-sulfur batteries: A comprehensive review on research progress and cell chemistry. Adv Energy Mater. 2017;7:1602829.
- Sun B, Xiong P, Maitra U, et al. Design strategies to enable the efficient use of sodium metal anodes in high-energy batteries. Adv Mater. 2020;32:1903891.
- Ye C, Jiao Y, Chao D, et al. Electron-state confinement of polysulfides for highly stable sodium-sulfur batteries. Adv Mater. 2020;32:1907557.
- Luo J, Lu X, Matios E, et al. Tunable MXene-derived 1d/2d hybrid nanoarchitectures as a stable matrix for dendrite-free and ultrahigh capacity sodium metal anode. Nano Lett. 2020;20:7700–7708.
- Wang Y, Shi H, Niu J, et al. Self-healing Sn4P3@hard carbon co-storage anode for sodium-ion batteries. J Alloys Compd. 2021;851:156746.
- Kumar D, Kuhar SB, Kanchan DK. Room temperature sodium-sulfur batteries as emerging energy source. J Energy Storage. 2018;18:133–148.
- Park K, Cho JH, Jang J-H, et al. Trapping lithium polysulfides of a Li-S battery by forming lithium bonds in a polymer matrix. Energy Environ Sci. 2015;8:2389–2395.
- Zhou J, Xu S, Yang Y. Strategies for polysulfide immobilization in sulfur cathodes for room-temperature sodium-sulfur batteries. Small. 2021;17:e2100057.
- Yu X, Manthiram A. Capacity enhancement and discharge mechanisms of room-temperature sodium-sulfur batteries. Chem Electro Chem. 2014;1:1275–1280.
- Zhang H, Diemant T, Qin B, et al. Solvent-dictated sodium sulfur redox reactions: investigation of carbonate and ether electrolytes. Energies. 2020;13:836.
- Yan Z, Tian Q, Liang Y, et al. Electrochemical release of catalysts in nanoreactors for solid sulfur redox reactions in room-temperature sodium-sulfur batteries. Cell Reports Phys Sci. 2021;2:100539.
- Zhang J, Wang D-W, Lv W, et al. Ethers illume sodium-based battery chemistry: uniqueness, surprise, and challenges. Adv Energy Mater. 2018;8:1801361.
- Wan B, Xu S, Yuan X, et al. Diversities of stoichiometry and electrical conductivity in sodium sulfides. J Mater Chem A. 2019;7:16472–16478.
- Zhang D, Li B, Wang S, et al. Simultaneous formation of artificial sei film and 3d host for stable metallic sodium anodes. ACS Appl Mater Interfaces. 2017;9:40265–40272.
- Zheng M, Chi Y, Hu Q, et al. Carbon nanotube-based materials for lithium-sulfur batteries. J Mater Chem A. 2019;7:17204–17241.
- Wu X, Qian C, Wu H, et al. Gestated uniform yolk-shell Sn@N-doped hollow mesoporous carbon spheres with buffer space for boosting lithium storage performance. Chem Commun. 2020;56:7629–7632.
- Ni L, Zhao G, Wang Y, et al. Coaxial carbon/MnO2 hollow nanofibers as sulfur hosts for high-performance lithium-sulfur batteries. Chem Asian J. 2017;12:3128–3134.
- Yan Z, Liang Y, Xiao J, et al. A high-kinetics sulfur cathode with a highly efficient mechanism for superior room-temperature Na-S batteries. Adv Mater. 2020;32:1906700.
- Luo C, Zhu Y, Borodin O, et al. Activation of oxygen-stabilized sulfur for Li and Na batteries. Adv Funct Mater. 2016;26:745–752.
- Eng A, Nguyen D, Kumar V, et al. Tailoring binder-cathode interactions for long-life room-temperature sodium-sulfur batteries. J Mater Chem A. 2020;8:22983–22997.
- Kim I, Kim CH, Sh C, et al. A singular flexible cathode for room temperature sodium/sulfur battery. J Power Sources. 2016;307:31–37.
- Wang Y, Li X, Wang W, et al. Chalcogen cathode and its conversion electrochemistry in rechargeable Li/Na batteries. Sci China Chem. 2020;63:1402–1415.
- Salama M R, Attias R, et al. Metal-sulfur batteries: overview and research methods. ACS Energy Lett. 2019;4:436–446.
- Wu Z, Wang W, Wang Y, et al. Three-dimensional graphene hollow spheres with high sulfur loading for high-performance lithium-sulfur batteries. Electrochim Acta. 2017;224:527–533.
- Gomes R, Bhattacharyya AJ. Carbon nanotube-templated covalent organic framework nanosheets as an efficient sulfur host for room-temperature metal-sulfur batteries. ACS Sustain Chem Eng. 2020;8:5946–5953.
- Wang W, Li Y, Feng Y, et al. Asymmetric self-supporting hybrid fluorinated carbon nanotubes/carbon nanotubes sponge electrode for high-performance lithium-polysulfide battery. Chem Eng J. 2018;349:756–765.
- Liu F, Cheng X, Xu R, et al. Binding sulfur-doped Nb2O5 hollow nanospheres on sulfur-doped graphene networks for highly reversible sodium storage. Adv Funct Mater. 2018;28:1800394.
- Li D, Gong B, Cheng X, et al. An efficient strategy toward multichambered carbon nanoboxes with multiple spatial confinement for advanced sodium-sulfur batteries. ACS Nano. 2021;15:20607–20618.
- Nersisyan HH, Joo SH, Yoo BU, et al. Combustion-mediated synthesis of hollow carbon nanospheres for high-performance cathode material in lithium-sulfur battery. Carbon. 2016;103:255–262.
- Tzadikov J, Levy NR, Abisdris L, et al. Bottom-up synthesis of advanced carbonaceous anode materials containing sulfur for Na-ion batteries. Adv Funct Mater. 2020;30:2000592.
- Yang Q, Yang T, Gao W, et al. An MXene-based aerogel with cobalt nanoparticles as an efficient sulfur host for room-temperature Na-S batteries. Inorg Chem Front. 2020;7:4396–4403.
- Zhou H, Xia X, Lv P, et al. C@TiO2/MoO3 composite nanofibers with 1T-phase MoS2 nanograin dopant and stabilized interfaces as anodes for Li- and Na-ion batteries. Chem Sus Chem. 2018;11:4060–4070.
- Yao Y, Liu P, Zhang Q, et al. Nitrogen-doped micropores binder-free carbon-sulphur composites as the cathode for long-life lithium-sulphur batteries. Mater Lett. 2018;231:159–162.
- Zhao S, Tian X, Zhou Y, et al. Three-dimensionally interconnected Co9S8/MWCNTs composite cathode host for lithium-sulfur batteries. J Energy Chem. 2020;46:22–29.
- Wu XW, Xie H, Deng Q, et al. Three-dimensional carbon nanotubes forest/carbon cloth as an efficient electrode for lithium-polysulfide batteries. ACS Appl Mater Interfaces. 2017;9:1553–1561.
- Xu X, Zhou D, Qin X, et al. A room-temperature sodium-sulfur battery with high capacity and stable cycling performance. Nat Commun. 2018;9:3870.
- Xin S, Yin YX, Guo YG, et al. A high-energy room-temperature sodium-sulfur battery. Adv Mater. 2014;26:1261–1265.
- Wang YX, Yang J, Lai W, et al. Achieving high-performance room-temperature sodium-sulfur batteries with S@interconnected mesoporous carbon hollow nanospheres. J Am Chem Soc. 2016;138:16576–16579.
- Guo Q, Li S, Liu X, et al. Ultrastable sodium-sulfur batteries without polysulfides formation using slit ultramicropore carbon carrier. Adv Sci. 2020;7:1903246.
- Yang J, Wang Y, Chou S, et al. Yolk-shell silicon-mesoporous carbon anode with compact solid electrolyte interphase film for superior lithium-ion batteries. Nano Energy. 2015;18:133–142.
- Sajjad M, Hussain T, Singh N, et al. Superior anchoring of sodium polysulfides to the polar C2N 2D material: A potential electrode enhancer in sodium-sulfur batteries. Langmuir. 2020;36:13104–13111.
- Wu C, Lei Y, Simonelli L, et al. Continuous carbon channels enable full Na-ion accessibility for superior room-temperature Na-S batteries. Adv Mater. 2022;34:2108363.
- Eng AYS, Wang Y, Nguyen DT, et al. Tunable nitrogen-doping of sulfur host nanostructures for stable and shuttle-free room-temperature sodium-sulfur batteries. Nano Lett. 2021;21:5401–5408.
- Xia G, Zhang L, Chen X, et al. Carbon hollow nanobubbles on porous carbon nanofibers: An ideal host for high-performance sodium-sulfur batteries and hydrogen storage. Energy Stor Mater. 2018;14:314–323.
- Ni L, Wu Z, Zhao G, et al. Core-shell structure and interaction mechanism of γ-MnO2 coated sulfur for improved lithium-sulfur batteries. Small. 2017;13:1603466.
- Zhang L, Zhang B, Dou Y, et al. Self-assembling hollow carbon nanobeads into double-shell microspheres as a hierarchical sulfur host for sustainable room-temperature sodium-sulfur batteries. ACS Appl Mater Interfaces. 2018;10:20422–20428.
- Carter R, Oakes L, Douglas A, et al. A sugar-derived room-temperature sodium sulfur battery with long term cycling stability. Nano Lett. 2017;17:1863–1869.
- Qiang Z, Chen Y-M, Xia Y, et al. Ultra-long cycle life, low-cost room temperature sodium-sulfur batteries enabled by highly doped (N,S) nanoporous carbons. Nano Energy. 2017;32:59–66.
- Yan J, Li W, Wang R, et al. An in situ prepared covalent sulfur-carbon composite electrode for high-performance room-temperature sodium-sulfur batteries. ACS Energy Lett. 2020;5:1307–1315.
- Lim CYJ, Eng AYS, Handoko AD, et al. Sulfurized cyclopentadienyl nanocomposites for shuttle-free room-temperature sodium-sulfur batteries. Nano Lett. 2021;21:10538–10546.
- Dong C, Zhou H, Liu H, et al. Inhibited shuttle effect by functional separator for room-temperature sodium-sulfur batteries. J Mater Sci Technol. 2022;113:207–216.
- Hao Y, Li X, Sun X, et al. Nitrogen-doped graphene nanosheets/S composites as cathode in room-temperature sodium-sulfur batteries. Chem Select. 2017;2:9425–9432.
- Shi Z, Yang Y, Huang Y, et al. Organic alkali metal salt derived three-dimensional N-doped porous carbon/carbon nanotubes composites with superior Li-S battery performance. ACS Sustain Chem Eng. 2019;7:3995–4003.
- Li H, Zhao M, Jin B, et al. Mesoporous nitrogen-doped carbon nanospheres as sulfur matrix and a novel chelate-modified separator for high-performance room-temperature Na-S batteries. Small. 2020;16:1907464.
- Shen Y, Huang C, Li Y, et al. Enhanced sodium and potassium ions storage of soft carbon by a S/O co-doped strategy. Electrochim Acta. 2021;367:137526.
- Wang Y, Zhang Y, Shi J, et al. Tin sulfide nanoparticles embedded in sulfur and nitrogen dual-doped mesoporous carbon fibers as high-performance anodes with battery-capacitive sodium storage. Energy Stor Mater. 2019;18:366–374.
- Yan Z, Xiao J, Lai W, et al. Nickel sulfide nanocrystals on nitrogen-doped porous carbon nanotubes with high-efficiency electrocatalysis for room-temperature sodium-sulfur batteries. Nat Commun. 2019;10:4793.
- Lei Y, Wu C, Lu X, et al. Streamline sulfur redox reactions to achieve efficient room-temperature sodium-sulfur batteries. Angew Chem Int Ed. 2022;61:e202200384. doi:https://doi.org/10.1002/anie.202200384.
- Ma D, Li Y, Yang J, et al. New strategy for polysulfide protection based on atomic layer deposition of TiO2 onto ferroelectric-encapsulated cathode: toward ultrastable free-standing room temperature sodium-sulfur batteries. Adv Funct Mater. 2018;28:1705537.
- Guo B, Du W, Yang T, et al. Nickel hollow spheres concatenated by nitrogen-doped carbon fibers for enhancing electrochemical kinetics of sodium-sulfur batteries. Adv Sci. 2020;7:1902617.
- Qin G, Liu Y, Han P, et al. High performance room temperature Na-S batteries based on FCNT modified Co3C-Co nanocubes. Chem Eng J. 2020;396:125295.
- Liu H, Lai W-H, Liang Y, et al. Sustainable S cathodes with synergic electrocatalysis for room-temperature Na-S batteries. J Mater Chem A. 2021;9:566–574.
- Zhang X, Zhao R, Wu Q, et al. Petal-like MoS2 nanosheets space-confined in hollow mesoporous carbon spheres for enhanced lithium storage performance. ACS Nano. 2017;11:8429–8436.
- Ma Q, Du G, Zhong W, et al. Template method for fabricating Co and Ni nanoparticles/porous channels carbon for solid-state sodium-sulfur battery. J Colloid Interface Sci. 2020;578:710–716.
- Tanibata N, Tsukasaki H, Deguchi M, et al. Characterization of sulfur nanocomposite electrodes containing phosphorus sulfide for high-capacity all-solid-state Na/S batteries. Solid State Ionics. 2017;311:6–13.
- Aslam MK, Seymour ID, Katyal N, et al. Metal chalcogenide hollow polar bipyramid prisms as efficient sulfur hosts for Na-S batteries. Nat Commun. 2020;11:5242.
- Sun Y, Shi P, Xiang H, et al. High-safety nonaqueous electrolytes and interphases for sodium-ion batteries. Small. 2019;15:1805479.
- Bao C, Wang B, Liu P, et al. Solid electrolyte interphases on sodium metal anodes. Adv Funct Mater. 2020;30:2004891.
- Zhao C, Liu L, Qi X, et al. Solid-state sodium batteries. Adv Energy Mater. 2018;8:1703012.
- Basile A, Hilder M, Makhlooghiazad F, et al. Ionic liquids and organic ionic plastic crystals: advanced electrolytes for safer high performance sodium energy storage technologies. Adv Energy Mater. 2018;8:1703491.
- Fan X, Yue J, Han F, et al. High-performance all-solid-state Na-S battery enabled by casting-annealing technology. ACS Nano. 2018;12:3360–3368.
- Liu Q, Wu F, Mu D, et al. A theoretical study on Na+ solvation in carbonate ester and ether solvents for sodium-ion batteries. Phys Chem Chem Phys. 2020;22:2164–2175.
- Syali MS, Kumar D, Mishra K, et al. Recent advances in electrolytes for room-temperature sodium-sulfur batteries: A review. Energy Stor Mater. 2020;31:352–372.
- Manthiram A, Yu X. Ambient temperature sodium-sulfur batteries. Small. 2015;11:2108–2114.
- Liu D, Li Z, Li X, et al. Stable room-temperature sodium-sulfur batteries in ether-based electrolytes enabled by the fluoroethylene carbonate additive. ACS Appl Mater Interfaces. 2022;14:6658–6666.
- Zhao X, Zhu Q, Xu S, et al. Fluoroethylene carbonate as an additive in a carbonates-based electrolyte for enhancing the specific capacity of room-temperature sodium-sulfur cell. J Electroanal Chem. 2019;832:392–398.
- Wu J, Liu J, Lu Z, et al. Non-flammable electrolyte for dendrite-free sodium-sulfur battery. Energy Stor Mater. 2019;23:8–16.
- Murugan S, Klostermann SV, Frey W, et al. A sodium bis(perfluoropinacol) borate-based electrolyte for stable, high-performance room temperature sodium-sulfur batteries based on sulfurized poly(acrylonitrile). Electrochem Commun. 2021;132:107137.
- Le PML, Vo TD, Pan H, et al. Excellent cycling stability of sodium anode enabled by a stable solid electrolyte interphase formed in ether-based electrolytes. Adv Funct Mater. 2020;30:2001151.
- Zhen Y, Sa R, Zhou K, et al. Breaking the limitation of sodium-ion storage for nanostructured carbon anode by engineering desolvation barrier with neat electrolytes. Nano Energy. 2020;74:104895.
- Chen K, Li H, Xu Y, et al. Untying thioether bond structures enabled by “voltage-scissors” for stable room temperature sodium-sulfur batteries. Nanoscale. 2019;11:5967–5973.
- Zhang C, Wang F, Han F, et al. Improved electrochemical performance of sodium/potassium-ion batteries in ether-based electrolyte: cases study of MoS2@C and Fe7S8@C anodes. Adv Mater Interfaces. 2020;7:2000486.
- Ryu H, Kim T, Kim K, et al. Discharge reaction mechanism of room-temperature sodium-sulfur battery with tetra ethylene glycol dimethyl ether liquid electrolyte. J Power Sources. 2011;196:5186–5190.
- Su NC, Noor SAM, Roslee MF, et al. Potential complexes of NaCF3SO3-tetraethylene dimethyl glycol ether (tetraglyme)-based electrolytes for sodium rechargeable battery application. Ionics. 2018;25:541–549.
- Seh ZW, Sun J, Sun Y, et al. A highly reversible room-temperature sodium metal anode. ACS Cent Sci. 2015;1:449–455.
- Wu J, Tian Y, Gao Y, et al. Rational electrolyte design toward cyclability remedy for room-temperature sodium-sulfur batteries. Angew Chem Int Ed. 2022: e202205416. doi:https://doi.org/10.1002/anie.202205416.
- Xiao F, Yang X, Wang H, et al. Covalent encapsulation of sulfur in a MOF-derived S, N-doped porous carbon host realized via the vapor-infiltration method results in enhanced sodium-sulfur battery performance. Adv Energy Mater. 2020;10:2000931.
- Hu P, Xiao F, Wu Y, et al. Covalent encapsulation of sulfur in a graphene/N-doped carbon host for enhanced sodium-sulfur batteries. Chem Eng J. 2022;443:136257.
- Luo S, Ruan J, Wang Y, et al. Flower-like interlayer-expanded MoS2-x nanosheets confined in hollow carbon spheres with high-efficiency electrocatalysis sites for advanced sodium-sulfur battery. Small. 2021;17:2101879.
- Ye H, Ma L, Zhou Y, et al. Amorphous MoS3 as the sulfur-equivalent cathode material for room-temperature Li-S and Na-S batteries. Proc Natl Acad Sci USA. 2017;114:13091–13096.
- Aslam MK, Hussain T, Tabassum H, et al. Sulfur encapsulation into yolk-shell Fe2N@nitrogen doped carbon for ambient-temperature sodium-sulfur battery cathode. Chem Eng J. 2022;429:132389.
- Ma Q, Zhong W, Du G, et al. Multi-step controllable catalysis method for the defense of sodium polysulfide dissolution in room-temperature Na-S batteries. ACS Appl Mater Interfaces. 2021;13:11852–11860.
- Tanibata N, Deguchi M, Hayashi A, et al. All-solid-state Na/S batteries with a Na3PS4 electrolyte operating at room temperature. Chem Mater. 2017;29:5232–5238.
- Sun J, Lin Y, Sun Z, et al. Highly cross-linked carbon sponge enables room-temperature long-life semi-liquid Na/polysulfide battery. Mater Today Energy. 2019;14:100342.
- Nikiforidis G, van de Sanden MCM, Tsampas MN. High and intermediate temperature sodium-sulfur batteries for energy storage: development, challenges and perspectives. RSC Adv. 2019;9:5649–5673.
- Murgia F, Brighi M, Černý R. Room-temperature-operating Na solid-state battery with complex hydride as electrolyte. Electrochem Commun. 2019;106:106534.
- Tang B, Jaschin PW, Li X, et al. Critical interface between inorganic solid-state electrolyte and sodium metal. Mater Today. 2020;41:200–218.
- Youcef HB, Orayech B, Del Amo JML, et al. Functionalized cellulose as quasi single-ion conductors in polymer electrolyte for all-solid-state Li/Na and Li-S batteries. Solid State Ionics. 2020;345:115168.
- Lu K, Li B, Zhan X, et al. Elastic NaxMoS2-carbon-base triple interface direct robust solid-solid interface for all-solid-state Na-S batteries. Nano Lett. 2020;20:6837–6844.
- Lu Y, Li L, Zhang Q, et al. Electrolyte and interface engineering for solid-state sodium batteries. Joule. 2018;2:1747–1770.
- Zhang Z, Wenzel S, Zhu Y, et al. Na3Zr2Si2PO12: a stable Na+-ion solid electrolyte for solid-state batteries. ACS Appl Energy Mater. 2020;3:7427–7437.
- Yu X, Manthiram A. Sodium-sulfur batteries with a polymer-coated Nasicon-type sodium-ion solid electrolyte. Matter. 2019;1:439–451.
- Zhao J, Yan G, Zhang X, et al. In situ interfacial polymerization of lithiophilic COF@PP and POP@PP separators with lower shuttle effect and higher ion transport for high-performance Li-S batteries. Chem Eng J. 2022;442:136352.
- Zhao Q, Wang R, Wen J, et al. Separator engineering toward practical Li-S batteries: targeted electrocatalytic sulfur conversion, lithium plating regulation, and thermal tolerance. Nano Energy. 2022;95:106982.
- Yang T, Guo B, Du W, et al. Design and construction of sodium polysulfides defense system for room-temperature Na-S battery. Adv Sci. 2019;6:1901557.
- Li H, Zhao M, Jin B, et al. Mesoporous nitrogen-doped carbon nanospheres as sulfur matrix and a novel chelate-modified separator for high-performance room-temperature Na-S batteries. Small. 2020;16:1907464.
- Saroha R, Heo J, Liu Y, et al. V2O3-decorated carbon nanofibers as a robust interlayer for long-lived, high-performance, room-temperature sodium-sulfur batteries. Chem Eng J. 2022;431:134205.
- Wang C, Wu K, Cui J, et al. Robust room-temperature sodium-sulfur batteries enabled by a sandwich-structured MXene@C/polyolefin/MXene@C dual-functional separator. Small. 2022;2106983; doi:https://doi.org/10.1002/smll.202106983.
- Wang YX, Lai WH, Chou SL, et al. Remedies for polysulfide dissolution in room-temperature sodium-sulfur batteries. Adv Mater. 2020;32:1903952.
- Fan L, Li X. Recent advances in effective protection of sodium metal anode. Nano Energy. 2018;53:630–642.
- Yang X, Gao X, Sun Q, et al. Promoting the transformation of Li2S2 to Li2S: significantly increasing utilization of active materials for high-sulfur-loading Li-S batteries. Adv Mater. 2019;31:1901220.
- Eng AYS, Kumar V, Zhang Y, et al. Room-temperature sodium-sulfur batteries and beyond: realizing practical high energy systems through anode, cathode, and electrolyte engineering. Adv Energy Mater. 2021;11:2003493.
- Ge P, Li S, Shuai H, et al. Ultrafast sodium full batteries derived from X-Fe (X = Co, Ni, Mn) prussian blue analogs. Adv Mater. 2019;31:1806092.
- Zhao C, Lu Y, Yue J, et al. Advanced Na metal anodes. J Energy Chem. 2018;27:1584–1596.
- Lee B, Paek E, Mitlin D, et al. Sodium metal anodes: emerging solutions to dendrite growth. Chem Rev. 2019;119:5416–5460.
- Zhao H, Deng N, Yan J, et al. A review on anode for lithium-sulfur batteries: progress and prospects. Chem Eng J. 2018;347:343–365.
- Wang Y, Huang XL, Liu H, et al. Nanostructure engineering strategies of cathode materials for room-temperature Na-S batteries. ACS Nano. 2022;16:5103.
- Qian J, Henderson WA, Xu W, et al. High rate and stable cycling of lithium metal anode. Nat Commun. 2015;6:6362.
- Yamada Y, Wang J, Ko S, et al. Advances and issues in developing salt-concentrated battery electrolytes. Nat Energy. 2019;4:269–280.
- He M, Lau KC, Ren X, et al. Concentrated electrolyte for the sodium-oxygen battery: solvation structure and improved cycle life. Angew Chem Int Ed Engl. 2016;55:15310–15314.
- Raccichini R, Dibden JW, Brew A, et al. Ion speciation and transport properties of LiTFSI in 1,3-dioxolane solutions: A case study for Li-S battery applications. J Phys Chem B. 2018;122:267–274.
- Ge P, Hou HS, Li S, et al. Tailoring rod-like FeSe2 coated with nitrogen-doped carbon for high-performance sodium storage. Adv Funct Mater. 2018;28:1801765.
- Cao Y, Yang C, Liu Y, et al. A new polyanion Na3Fe2(PO4)P2O7 cathode with high electrochemical performance for sodium-ion batteries. ACS Energy Lett. 2020;5:3788–3796.
- Lee J, Lee Y, Lee J, et al. Ultraconcentrated sodium bis(fluorosulfonyl)imide-based electrolytes for high-performance sodium metal batteries. ACS Appl Mater Interfaces. 2017;9:3723–3732.
- Zheng J, Chen S, Zhao W, et al. Extremely stable sodium metal batteries enabled by localized high-concentration electrolytes. ACS Energy Lett. 2018;3:315–321.
- Zhang Q, Lu Y, Miao L, et al. An alternative to lithium metal anodes: Non-dendritic and highly reversible sodium metal anodes for Li-Na hybrid batteries. Angew Chem Int Ed Engl. 2018;57:14796–14800.
- Zhou W, Li Y, Xin S, et al. Rechargeable sodium all-solid-state battery. ACS Cent Sci. 2017;3:52–57.
- Wang H, Wang C, Matios E, et al. Facile stabilization of the sodium metal anode with additives: unexpected key role of sodium polysulfide and adverse effect of sodium nitrate. Angew Chem Int Ed Engl. 2018;57:7734–7737.
- Zhang X-Q, Cheng X-B, Chen X, et al. Fluoroethylene carbonate additives to render uniform Li deposits in lithium metal batteries. Adv Funct Mater. 2017;27:1605989.
- Han M, Zhu C, Ma T, et al. In situ atomic force microscopy study of nano-micro sodium deposition in ester-based electrolytes. Chem Commun. 2018;54:2381–2384.
- Lee Y, Lee J, Lee J, et al. Fluoroethylene carbonate-based electrolyte with 1 m sodium bis(fluorosulfonyl)imide enables high-performance sodium metal electrodes. ACS Appl Mater Interfaces. 2018;10:15270–15280.
- Ding F, Xu W, Graff GL, et al. Dendrite-free lithium deposition via self-healing electrostatic shield mechanism. J Am Chem Soc. 2013;135:4450–4456.
- Shi Q, Zhong Y, Wu M, et al. High-performance sodium metal anodes enabled by a bifunctional potassium salt. Angew Chem Int Ed Engl. 2018;57:9069–9072.
- Ma Q, Liu J, Qi X, et al. A new Na[(FSO2)(n-C4F9SO2)N]-based polymer electrolyte for solid-state sodium batteries. J Mater Chem A. 2017;5:7738–7743.
- Sun B, Pompe C, Dongmo S, et al. Challenges for developing rechargeable room-temperature sodium oxygen batteries. Adv Mater Technol. 2018;3:1800110.
- Luo W, Lin C, Zhao O, et al. Ultrathin surface coating enables the stable sodium metal anode. Adv Energy Mater. 2017;7:1601526.
- Wang H, Wang C, Matios E, et al. Critical role of ultrathin graphene films with tunable thickness in enabling highly stable sodium metal anodes. Nano Lett. 2017;17:6808–6815.
- Zhao Y, Goncharova L, Zhang Q, et al. Inorganic-organic coating via molecular layer deposition enables long life sodium metal anode. Nano Lett. 2017;17:5653–5659.
- Kumar V, Wang Y, Eng AYS, et al. A biphasic interphase design enabling high performance in room temperature sodium-sulfur batteries. Cell Reports Phys Sci. 2020;1:100044.
- Kumar V, Eng AYS, Wang Y, et al. An artificial metal-alloy interphase for high-rate and long-life sodium–sulfur batteries. Energy Stor Mater. 2020;29:1–8.
- Kim YJ, Lee H, Noh H, et al. Enhancing the cycling stability of sodium metal electrodes by building an inorganic-organic composite protective layer. ACS Appl Mater Interfaces. 2017;9:6000–6006.
- Tian H, Seh ZW, Yan K, et al. Theoretical investigation of 2D layered materials as protective films for lithium and sodium metal anodes. Adv Energy Mater. 2017;7:1602528.
- Ma C, Xu T, Wang Y. Advanced carbon nanostructures for future high performance sodium metal anodes. Energy Stor Mater. 2020;25:811–826.
- Wang A, Hu X, Tang H, et al. Processable and moldable sodium-metal anodes. Angew Chem Int Ed Engl. 2017;56:11921–11926.
- Chi S-S, Qi X-G, Hu Y-S, et al. 3D flexible carbon felt host for highly stable sodium metal anodes. Adv Energy Mater. 2018;8:1702764.
- Luo W, Zhang Y, Xu S, et al. Encapsulation of metallic Na in an electrically conductive host with porous channels as a highly stable Na metal anode. Nano Lett. 2017;17:3792–3797.
- Xu Y, Menon AS, Harks PPRML, et al. Honeycomb-like porous 3D nickel electrodeposition for stable Li and Na metal anodes. Energy Stor Mater. 2018;12:69–78.
- Sun B, Li P, Zhang J, et al. Dendrite-free sodium-metal anodes for high-energy sodium-metal batteries. Adv Mater. 2018;30:1801334.
- Li Y, Wang C, Wang W, et al. Enhanced chemical immobilization and catalytic conversion of polysulfide intermediates using metallic Mo nanoclusters for high-performance Li-S batteries. ACS Nano. 2020;14:1148–1157.
- Lu Y, Zhang Q, Han M, et al. Stable Na plating/stripping electrochemistry realized by a 3D Cu current collector with thin nanowires. Chem Commun. 2017;53:12910–12913.
- Liu S, Tang S, Zhang X, et al. Porous al current collector for dendrite-free Na metal anodes. Nano Lett. 2017;17:5862–5868.
- Ge P, Li S, Xu L, et al. Hierarchical hollow-microsphere metal-selenide@carbon composites with rational surface engineering for advanced sodium storage. Adv Energy Mater. 2019;9:1803035.