Abstract
We report the effect of grain boundary diffusion of the Nd-Cu eutectic phase and the influence of Nb on the microstructure and magnetic properties of Nd-Fe-B melt-spun ribbons. The Atom probe tomography analysis indicated the presence of Nb clusters (∼10 nm), which possibly restricts grain growth during diffusion annealing while facilitating Nd-Cu grain boundary decoration. Correspondingly, the coercivity was enhanced from the initial 1.2 T of the as-prepared to 2.1 T for the 20 wt.% Nd-Cu diffusion annealed (600°C, 2 h). Incidentally, the as-diffused sample exhibited a high coercivity of ∼1 T at 150°C, making it suitable for high-temperature applications.
GRAPHICAL ABSTRACT
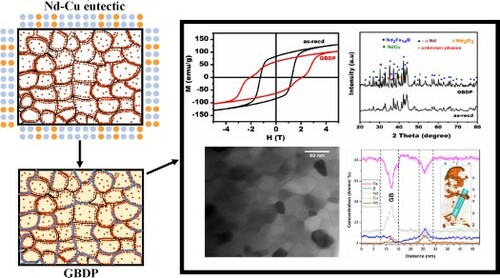
Introduction
Since its discovery by Sagawa in 1984, the Nd-Fe-B magnet has been one of the most sought-after permanent magnetic materials for many applications due to its exceptional combination of magnetic properties [Citation1]. The use of magnets in traction motors of hybrid and electric vehicles has led to a surge in demand for Nd-Fe-B magnetic material. The relatively low coercivity (∼1.2 T) of the Nd-Fe-B magnet is an issue as it further deteriorates at a higher temperature, making the magnet unusable at about 150°C, the typical operating temperature of the traction motors [Citation2]. Hence, the room temperature (RT) coercivity of Nd-Fe-B magnets must be enhanced to realize the required coercivity of 0.8 T at 150°C [Citation3]. Therefore, achieving higher coercivity at RT is a key research topic in the area of Nd-Fe-B magnet. It is well known that coercivity depends on the magnetocrystalline anisotropy and the microstructure of the alloy [Citation3,Citation4]. Attempts have been made to increase the magnetocrystalline anisotropy by replacing some of the Nd with heavy rare earth elements, such as Dy and Tb, as Dy2Fe14B and Tb2Fe14B have higher magnetocrystalline anisotropy than Nd2Fe14B [Citation5,Citation6]. Although this method is commercially adopted, it is not favoured due to the scarcity, and Dy (543$/kg) and Tb (2766$/kg) are costlier than Nd (173$/kg) [Citation7]. Another approach to enhance the coercivity of Nd-Fe-B magnets is to tailor the microstructure (viz. grain size, grain boundary (GB) engineering) as coercivity is a microstructure-sensitive property. Generally, Nd-Fe-B powders are synthesized by different processing methods, such as powder metallurgy [Citation8], hydrogenation disproportionation desorption recombination (HDDR) [Citation9], and melt-spinning [Citation10,Citation11]. The melt-spinning process yields fine nano-sized Nd2Fe14B grains (size < 100 nm), which is favourable for obtaining high coercivity [Citation12]. The typical phases present in the melt-spun Nd-Fe-B ribbons are hard Nd2Fe14B, soft α-Fe, and Nd2Fe17. The soft α-Fe phase is known to reduce the coercivity of Nd-Fe-B [Citation13]. Hence, the alternative method to enhance the coercivity is to optimize the microstructure, viz., suppressing the formation of the soft α-Fe phase [Citation13], reducing the grain size, and tuning the grain boundary (GB) chemistry [Citation14]. The doping elements, such as Ga, Al, Nb, and Cu, have been used in this context and have enhanced the coercivity albeit with different mechanisms [Citation15,Citation16]. Among all the dopants, Nb has shown a significant effect on enhancing coercivity [Citation16]. However, there are limited reports on the Nb-doped Nd-Fe-B melt-spun ribbons, especially for the cases where GB mobility is to be restricted during annealing. The main benefit of Nb doping is that it forms a phase with Fe, namely NbFeB, thereby suppressing the formation of α-Fe [Citation17,Citation18]. The formation of the NbFeB phase is attributed to the coercivity enhancement in Nb, containing Nd-Fe-B magnet as it pins the domain walls and restricts the growth of Nd2Fe14B grains. The pinning of magnetic domain walls also has a significant impact on coercivity [Citation19,Citation20]. It is reported that 1.5 at.% Nb doping in Nd-Fe-B can increase the coercivity to 1.4 T at RT but is still low for high-temperature applications [Citation21]. It is reported that magnetic isolation of Nd2Fe14B grains by non-ferromagnetic species along the GBs reduces the exchange interactions and thereby increases the coercivity of Nd-Fe-B [Citation22]. Therefore, to attain high coercivity, it is very critical to develop nanosized Nd-Fe-B grains with non-ferromagnetic species, decorating the GBs. It is known that the grain boundary diffusion process (GBDP), involving low-melting eutectic alloys, is the most notable and effective approach for enhancing coercivity [Citation23]. However, this process can lead to grain growth, partially negating the benefits of GBDP [Citation24]. H. Sepehri-Amin et al. [Citation25] discussed the significance of restricting the grain size during diffusion heat treatment to achieve high coercivity. As Nb doping in Nd-Fe-B restricts grain growth, GBDP in Nb-contained Nd-Fe-B can be advantageous for coercivity enhancement [Citation21]. Therefore, the objective of the present work is to enhance the coercivity of Nb-containing Nd-Fe-B melt-spun ribbon by GDBP using Nd70Cu30 low melting eutectic alloy. Magnetic measurements, exchange interaction studies, and microstructural investigations are performed to elucidate the role of Nb and correspondingly the mechanism for the observed coercivity enhancement.
Experiments
Commercial Nd-Fe-B melt-spun flakes with a nominal composition of Nd12Fe80.5B6Nb1.5 (at.%, hereafter referred to as ‘as-recd’) with an average particle size of 250 µm were used as starting powders. Binary Nd70Cu30 (at.%; hereafter referred to as Nd-Cu) eutectic alloy with a melting temperature of 520°C was used as a diffusion source. Nd70Cu30 was prepared by arc melting of elemental Neodymium (Nd; 99.9% purity; American elements) and elemental copper (Cu; 99.9% purity; American elements) in a water-cooled Cu hearth. The sample preparation, including GBDP employed, was as follows: pulverizing as-recd and Nd-Cu to ∼14 µm and ∼18 µm, respectively, consequently mixing and packing the as-recd with Nd-Cu pulverized powders in a quartz tube under an inert atmosphere inside a glove box (O2 and H2O ≤1 ppm). The diffusion heat treatment of the packaged samples was carried out at 600°C with different proportions of Nd-Cu eutectic (10, 20, and 30 wt%) and also the duration of heat treatment (1hr and 2hr) in a vacuum (10–5 mbar). The magnetic measurements of the as-received and 20 wt.% Nd-Cu eutectic diffused GBDP (herein after GBDP represents 20 wt.% Nd-Cu-diffused Nd-Fe-B-Nb) samples were taken using the Physical Property Measurement System (PPMS: Quantum design, USA) at a maximum field of 9 T. The X-ray diffraction (XRD: Rigaku Smart Lab X-ray diffractometer, Japan using Cu-K radiation (λ = 1.5406) in the scan range of 20–70° at a scan speed of 2°/min) was used to analyse the crystal structure of the phases. Microstructural studies along with Energy Dispersive X-ray spectroscopy (EDS)-based chemical mapping were carried out using Tecnai T20 Transmission Electron Microscope (TEM) and Talos F200S Scanning Transmission Electron Microscope (STEM). Three-dimensional atom-probe tomography (3D-APT) measurements were carried out using a local electrode atom probe (LEAP 5000XR, Cameca Instruments) applying laser pulses at 250 kHz frequency with laser energy of 30 pJ to study the composition of the intergranular phases and the Nd-rich regions. The hemispherical tips for the APT analyses were prepared using a Dual Beam Microscope with Focussed Ion Beam (FIB), namely, Helios G4 UX by the lift-out method [Citation26,Citation27].
Results and discussions
The magnetic properties of all the GBDP samples are shown in Table . The maximum coercivity is obtained for the sample with 20 wt.% Nd-Cu diffusion heat treated at 600°C for 2 h (referred: GBDP Sample) and hence is chosen for a detailed analysis to elucidate the coercivity mechanism. Figure (a) shows the hysteresis loop measured for the as-recd and GBDP samples, respectively. The GBDP sample exhibited a coercivity of 2.1 T, much higher than that of the as-recd sample (1.2 T). The energy product (BHmax) of the as-recd and GBDP sample is 13 and 7 MGOe, respectively. The temperature dependence of coercivity values for the as-recd and GBDP samples is shown in Figure (b). A coercivity of ∼1 T is obtained in the GBDP sample at 150°C compared to 0.5 T in the as-recd sample, which will be suitable for operation at elevated temperature (150°C–200°C) applications.
Figure 1. (a) Magnetic properties of the as-received (as-recd) and grain boundary diffused sample (20 wt.% Nd-Cu in Nd-Fe-B-Nb (GBDP)) with (a) showing the enhancement in room temperature coercivity for GBDP and (b) temperature dependence of coercivity with GBDP exhibiting higher coercivity across the entire temperature range.
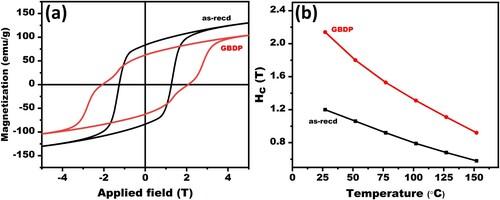
Table 1. Coercivity values obtained for various diffusion conditions to obtain the optimal combination of parameters.
Figure (a) shows the XRD pattern, which confirms the presence of Nd2Fe14B as the major phase in the as-received sample. It could be noted that no prominent peak corresponding to α-Fe (BCC structure) is detected from the XRD pattern as it is apparent that the addition of Nb prevents the formation of the α-Fe phase [Citation17,Citation18]. The XRD pattern of the GBDP sample shows the presence of additional peaks along with Nd2Fe14B, which were identified as α-Nd and NdCu phases. The peak profiles were broad and overlapping, typical for the melt-spun samples, suggesting the presence of nano-sized grains. According to the Debye Scherrer method, the grain size of the as-recd and GBDP sample was 22 ± 4 nm and 25 ± 4 nm, respectively. Transmission electron micrographs of the as-recd and GBDP sample (as shown in Figure (b,c)) are in good conformity with the grain size estimated from the XRD measurements. The average grain size for the as-recd and GBDP sample was 23 ± 1.5 nm and 26 ± 2.3 nm, respectively estimated from the grain size distribution, shown as an insert in the respective bright-field TEM images. There is no significant grain size difference between the as-recd and GBDP samples, unlike earlier reports wherein a significant grain growth has been reported for similar annealing conditions during grain boundary diffusion affecting the coercivity [Citation25,Citation28]. In the present case, there is an insignificant grain growth during the diffusion process, which could be attributed to the presence of the Nb-rich clusters that hinders the grain growth.
Figure 2. (a) shows the X-ray diffraction pattern for the as-recd and GBDP (20 wt.% Nd-Cu in Nd-Fe-B-Nb) sample along with the TEM micrographs showing a similar grain size for (b) as-recd and (c) GBDP samples. The grain size distribution plot along with magnified images of Nd-Fe-B grains are shown in the set. The dark sporadic contrast (indicated by arrows) visible in as-recd magnified image represents the Nb clusters, while the GBDP sample had Nd-Cu segregation along grain boundaries as visible in bright contrast (indicated by arrows) in the STEM-HAADF insert image. (d) STEM-EDS mapping of Nd and Cu corresponding to HAADF image in the inset of GBDP sample indicating the predominant segregation along grain boundaries.
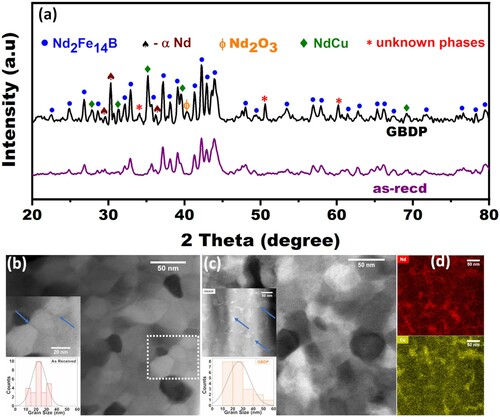
For the as-recd condition, the magnified bright field TEM image in the inset of Figure (b) shows sporadic dark contrast regions (indicated by arrows) around GBs possibly representing the Nb-rich clusters. Similarly, the STEM High Angle Annular Dark Field (HAADF) image in the inset of Figure (c) indicates GBs where Nd-Cu eutectics have diffused as bright contrast regions (∼70% area fraction indicated by arrows). This observation indicates that the GBDP has resulted in the inhomogeneous decoration of GBs. Furthermore, STEM-EDS mapping of eutectic species, namely, Nd and Cu, was performed to ascertain the distribution characteristics, as shown in Figure (d). It is apparent that not all GBs are decorated by the 20 wt.% Nd-Cu eutectic.
Figure (a) shows the proximity histogram corresponding to the Nb cluster (indicated by the rectangle in the inset) delineated with an isodensity surface in the Nb elemental distribution map of the as-received condition. The Nb concentration in the core of the cluster was 29 ± 4 at.%; the corresponding Nb content in the matrix region was 1.3 ± 0.17 at.%. The enlarged view of the Nb cluster is shown in Figure (a). The three-dimensional distribution maps of the constituent elements, such as Fe, B, and Nd, appear to be homogeneously distributed within the analysed volume, as indicated in Figure (b) for the as-received condition.
Figure 3. Three-dimensional atom probe measurements with (a) proximity histogram of the selected Nb cluster in the inset along with the 3D distribution of clusters delineated with 3 at.% the Nb isoconcentration surface of the as-recd sample; (b) Elemental distribution maps showing the homogeneous distribution of Fe, B and Nd in the as-recd sample; (c) proximity histogram of the selected Nb cluster in inset along with the 3D distribution of Nb clusters delineated with 3 at.% the Nb isoconcentration surface of the GBDP (20 wt.% Nd-Cu in Nd-Fe-B-Nb) sample (d) 1D Compositional profile obtained from 10 nm diameter cylindrical region of interest with 0.5 nm bin width along the Cu-enriched region (highlighted by isodensity surface) of the GBDP sample.
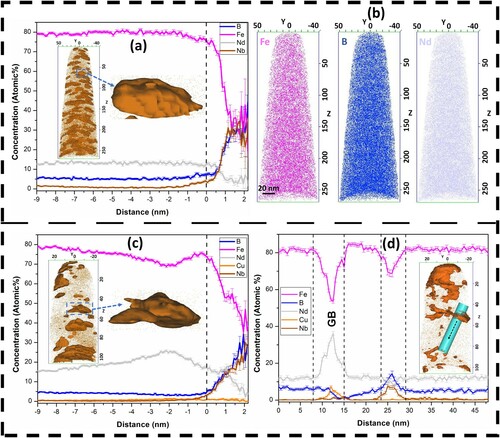
Similarly, the proximity histogram for the GBDP sample is shown in Figure (c). The presence of ∼10 nm diameter-sized Nb-rich clusters is apparent (inset of Figure (c)), which did not coarsen during annealing, as can be evidenced from the delineated Nb-rich regions using a 3 at.% Nb iso-concentration surface. The average Nb concentration in the core of the clusters was 23 ± 3.5 at.% and that in the matrix was 0.62 ± 0.08 at.%. According to the earlier studies, the low solubility of only ∼0.7 wt.% Nb in the Nd2Fe14B phase [Citation29] results in the formation of Nb-rich clusters, which hinders the grain growth, thereby facilitating the retention of nanosized grains during subsequent heat treatment [Citation21]. Figure (d) presents the 1D concentration profile taken along the 10 nm diameter cylindrical region of interest as shown in the inset, which also depicts the local enrichment of Cu distinguished using an isodensity surface. An apparent enrichment of Nd and Cu, along with the supposed GB together with the depletion of the Fe and B, can be noticed.
Figure 4. Schematic representing the process of GBDP where intentional Nb was added to suppress the grain boundary mobility in comparison with grain coarsening by annealing in the absence of Nb.
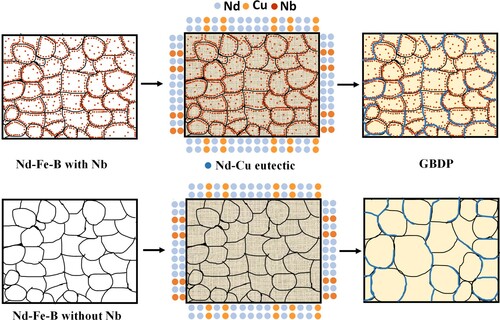
Figure represents the mechanism of GBDP in the presence of Nb, which, by forming nano-scale clusters, hinders the mobility of Nd-Fe-B GBs during diffusion annealing and retaining small grain sizes with a large fraction of GBs. On the contrary, the same annealing process without the presence of Nb results in grain coarsening, as depicted in the schematic. The nanoscale Nb clusters did not coarsen during GBDP and hence assisted in the enhancement of coercivity by facilitating the diffusion of Nd-Cu eutectic. The GB-decorated Nd-Fe-B grains may more respond to the applied magnetic field in isolation than the non-GB decorated Nd-Fe-B grains.
The initial magnetization curves (IMC) of both the samples shown in Figure (a) exhibit an S-shaped curve with a change in slope of the magnetization curve (indicated by arrows) at 1.2 T for the as-received sample, while it has increased to ∼3 T for the GBDP sample. Figure (a) shows the derivative of the IMC of both the samples, which helps identify the fields at which slopes (the shaded region) deviate from the IMC. The low-field peak around 1.2 T is observed in the as-recd and the GBDP samples, due to Nb-rich clusters. However, the high pinning field peak is observed only for the GBDP sample, which is attributed to the pinning by the Nd-enriched grain boundary phase. The observations from the APT data are in good agreement with this proposition as the as-recd sample lacks a distinct GB phase, while in the case of the GBDP sample (shown in Figure (d)), it is obvious that the low melting Nd-Cu eutectic phase decorates the GB, making it discernible and weaken the exchange interaction. Thus, the combined effect of Nb-rich clusters and the effective pinning of domain walls at the Nd-Cu-enriched GBs lead to the observed enhancement in coercivity. The reduction in exchange interaction in the GBDP sample due to the distinct GB decoration is also proved from the Henkel plots shown in Figure (b), wherein the intensity of the δM peak is almost reduced to half compared to the as-recd sample. Also, the typical GB composition of the GBDP sample was Nd27Fe66B3.7Cu3.3 from the APT GB analysis, which shows the presence of substantial Fe content in the GB. Akimasa Sakuma et al. have reported that in those cases where the Nd content is lower than 70 at%, the GB was ferromagnetic [Citation30], which explains the observed positive δM values of the GBDP sample (Figure (b)). The effect of pinning is further confirmed by the coercivity measured as a function of the applied field, as shown in Figure (c). A higher applied field is required to obtain the saturated coercivity of 2.1 T for the GBDP sample compared to the as-recd sample, which confirms the effective domain wall pinning in the former sample.
Figure 5. (a) Initial magnetization curve of the as-recd and GBDP (20 wt.% Nd-Cu in Nd-Fe-B-Nb) along with the inset showing the derivative identifying the kinks due to the pinning of domain walls with (b) and (c) showing the Henkel plot and coercivity measured as a function of the applied field, respectively showing the reduction in exchange in interaction and effective domain wall pinning and due to grain boundary in the GBDP sample.
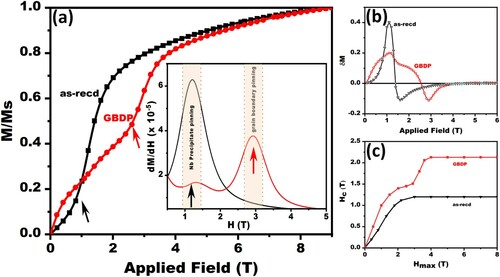
Conclusions
Grain boundary diffusion, using the Nd-Cu eutectic phase, was carried out in 1.5 at.% Nb containing Nd-Fe-B melt-spun ribbons. The GBDP (20 wt.% Nd-Cu-diffused Nd-Fe-B-Nb) sample exhibited a coercivity of 2.1 T compared to the 1.2 T of the as-prepared melt-spun ribbons. The following conclusions can be drawn as the reasons for the 75% enhancement in coercivity.
Nb-rich (>22 at.% at the core) clusters were effective in restricting the grain growth (∼25 nm) during the diffusion annealing (600°C for 2 h) process as the grain size of Nd2Fe14B grains was compared with the as-prepared condition.
STEM-EDS observation of GBs confirmed the GB diffusion of Nd-Cu eutectic of which atleast 70% by area fraction were decorated.
The Nd-Cu segregation along the GBs has reduced the exchange coupling between the Nd2Fe14B grains.
Thus, Nb clusters play a crucial role in the GBDP of Nd-Fe-B to enhance coercivity.
Acknowledgement
The authors would like to acknowledge the financial support from the Technical Research Centre (TRC), Science and Engineering Research Board (SERB) (REPM project: IR/SB/EF/07/2021-C), Government of India, for the completion of this work. They also thank Dr. G. Padmanabham, former Director, and Dr. T. N. Rao, Director (Additional Charge) ARCI. The authors thank Dr. S. Kavita for magnetic measurements. They acknowledge the support of the National Facility for Atom Probe Tomography for performing APT measurements. MS and KGP acknowledge the funding support from the Ministry of Education through the Institute of Eminence (IoE) initiative for establishing the Center of Excellence in Correlative Microscopy. KGP is grateful for the funding support from Science and Engineering Research Board (SERB) under project No. ECR/2018/002938.
Disclosure statement
No potential conflict of interest was reported by the author(s).
Additional information
Funding
References
- Kim AS, Camp FE. High performance NdFeB magnets (invited). J Appl Phys. 1996;79:5035–5039. DOI:10.1063/1.361566
- Vaimann T, Kallaste A, Kilk A, et al. Magnetic properties of reduced Dy NdFeB permanent magnets and their usage in electrical machines. IEEE AFRICON Conf.; 2013. DOI:10.1109/AFRCON.2013.6757787
- Woodcock TG, Zhang Y, Hrkac G, et al. Understanding the microstructure and coercivity of high performance NdFeB-based magnets. Scr Mater. 2012;67:536–541. DOI:10.1016/j.scriptamat.2012.05.038
- Gao X, Li J, Zhao W, et al. Microstructure evolution and coercivity enhancement of sintered Nd-Fe-B magnets by grain boundary diffusion with Cu aided TbF3. Mater Res Express. 2020;7:16101. DOI:10.1088/2053-1591/ab56f4.
- De Groot CH, Buschow KHJ, De Boer FR, et al. Two-powder Nd 2 Fe 14 B magnets with DyGa-addition two-powder Nd 2 Fe 14 B magnets with DyGa addition. 2010;388. DOI:10.1063/1.366734
- Lee YI, Chang HW, Huang GY, et al. Comparison on the coercivity enhancement of hot deformed Nd2Fe14B-type magnets by doping R70Cu30 (R = Nd, Dy and Tb) alloy powders. IEEE Trans Magn. 2017;53:1–4. DOI:10.1109/TMAG.2017.2703128
- Mancheri NA. World trade in rare earths, Chinese export restrictions, and implications. Resour Policy. 2015;46:262–271. DOI:10.1016/j.resourpol.2015.10.009
- Jurczyk M, Cook JS, Collocott SJ. Application of high energy ball milling to the production of magnetic powders from NdFeB-type alloys. J Alloys Compd. 1995;217:65–68. DOI:10.1016/0925-8388(94)01291-O
- Gutfleisch O, Eckert D, Schäfer R, et al. Magnetization processes in two different types of anisotropic, fully dense NdFeB hydrogenation, disproportionation, desorption, and recombination magnets. J Appl Phys. 2000;87:6119–6121. DOI:10.1063/1.372628
- Hua HC, Wang GY, Zheng CH, et al. Microstructure of melt-spun NdFeB magnet. Mater Lett. 1988;7:65–67. DOI:10.1016/0167-577X(88)90085-7
- Brown D, Ma BM, Chen Z. Developments in the processing and properties of NdFeb-type permanent magnets. J Magn Magn Mater. 2002;248:432–440. DOI:10.1016/S0304-8853(02)00334-7
- Madugundo R, Rama Rao NV, Schönhöbel AM, et al. Recent developments in nanostructured permanent magnet materials and their processing methods. 2018. DOI:10.1016/B978-0-12-813904-2.00006-1
- Ahmad I, Davies HA, Buckley RA. The effect of Nd content on the structure and properties of melt spun Nd-rich NdFeB alloys. J Magn Magn Mater. 1996;157–158:31–32. DOI:10.1016/0304-8853(95)01050-5
- Suresh K, Ohkubo T, Takahashi YK, et al. Consolidation of hydrogenation-disproportionation-desorption-recombination processed Nd-Fe-B magnets by spark plasma sintering. J Magn Magn Mater. 2009;321:3681–3686. DOI:10.1016/j.jmmm.2009.07.010
- Davies BE, Mottram RS, Harris IR. Recent developments in the sintering of NdFeB. Mater Chem Physcis. 2001;67:272–281. DOI:10.1002/chin.200120238
- Pandian S, Chandrasekaran V, Markandeyulu G, et al. Effect of Al, Cu, Ga, and Nb additions on the magnetic properties and microstructural features of sintered NdFeB effect of Al, Cu, Ga, and Nb additions on the magnetic properties and microstructural features of sintered NdFeB. 2014;6082. DOI:10.1063/1.1513879
- Pandian S, Chandrasekaran V, Iyer KJL, et al. Microstructural and magnetic studies on P/M processed (Nd14.9Dy1.9) (Fe65.0Co8.0Cu1.0Ga1.0 Nb0.7) B7.5 alloy. J Mater Sci. 2001;36:5903–5907. DOI:10.1023/A:1012932827861
- Ahmed FM, Edgley DS, Harris IR. Investigation of the homogenisation behaviour of Nd-Fe-Nb-B alloys. J Alloys Compd. 1995;224:135–139. DOI:10.1016/0925-8388(94)01502-3
- Ferguson GB, O’Grady K, Popplewel J, et al. Magnetisation mechanisms and magnetic viscosity in NdFeB alloys. IEEE Trans Magn. 1989;25:3449–3451. DOI:10.1109/20.42331
- Li J, Sepehri-Amin H, Sasaki T, et al. Most frequently asked questions about the coercivity of Nd-Fe-B permanent magnets. Sci Technol Adv Mater. 2021;22:386–403. DOI:10.1080/14686996.2021.1916377
- Chen Z, Wu YQ, Kramer MJ, et al. A study on the role of Nb in melt-spun nanocrystalline Nd-Fe-B magnets. J Magn Magn Mater. 2004;268:105–113. DOI:10.1016/S0304-8853(03)00481-5
- Hono K, Sepehri-Amin H. Strategy for high-coercivity Nd-Fe-B magnets. Scr Mater. 2012;67:530–535. DOI:10.1016/j.scriptamat.2012.06.038
- Sawatzki S, Kübel C, Ener S, et al. Grain boundary diffusion in nanocrystalline Nd-Fe-B permanent magnets with low-melting eutectics. Acta Mater. 2016;115:354–363. DOI:10.1016/j.actamat.2016.05.048
- Liu Z, He J, Ramanujan RV. Significant progress of grain boundary diffusion process for cost-effective rare earth permanent magnets: a review. Mater Des. 2021;209:110004. DOI:10.1016/j.matdes.2021.110004
- Sepehri-Amin H, Prabhu D, Hayashi M, et al. Coercivity enhancement of rapidly solidified Nd-Fe-B magnet powders. Scr Mater. 2013;68:167–170. DOI:10.1016/j.scriptamat.2012.10.005
- Basu I, Pradeep KG, Mießen C, et al. The role of atomic scale segregation in designing highly ductile magnesium alloys. Acta Mater. 2016;116:77–94. DOI:10.1016/j.actamat.2016.06.024
- Mandal S, Pradeep KG, Zaefferer S, et al. A novel approach to measure grain boundary segregation in bulk polycrystalline materials in dependence of the boundaries’ five rotational degrees of freedom. Scr Mater. 2014;81:16–19. DOI:10.1016/j.scriptamat.2014.02.016
- Xie J, Yuan C, Luo Y, et al. Coercivity enhancement and thermal-stability improvement in the melt-spun NdFeB ribbons by grain boundary diffusion. J Magn Magn Mater. 2017. DOI:10.1016/j.jmmm.2017.08.047
- Rodewald W, Wall B. Structure and magnetic properties of sintered Nd-Fe-Nb-B magnets. J Magn Magn Mater. 1989;80:57–60. DOI:10.1016/0304-8853(89)90324-7
- Sakuma A, Suzuki T, Furuuchi T, et al. Magnetism of Nd-Fe films as a model of grain boundary phase in Nd-Fe-B permanent magnets. Appl Phys Express. 2016;9:013002. DOI:10.7567/APEX.9.013002