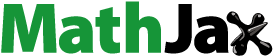
Abstract
Rational design of hollow mesoporous silicas with distinctive morphology has attracted considerable interest for their promising applications of different functions. Herein, we reported hollow mesoporous silica ellipsoids with quasiperiodic cocoon-shape, and fully investigated the evolution of external morphology and interior structure with solution concentrations. However, synthetic theories used so far hardly provide reasonable explanation. Therefore, a morphogenetic mechanism upon interfacial destabilization dynamics is proposed based on the experimental results, in which unstable interfaces of emulsions influenced by harmonic perturbations are employed as secondary templates for the nonequilibrium deposition/diffusion of rod-like micelles, explaining well the controllability of the morphology.
GRAPHICAL ABSTRACT

IMPACT STATEMENT
Taro-like hollow mesoporous silicas were produced via a secondary-templating strategy. A nonequilibrium growth based on interfacial destabilization dynamics is proposed for further design of mesoporous silicas with other distinctive patterns.
Introduction
Structure direction and morphology control are extremely important in terms of material functionality and utility [Citation1–6]. Recently, the rational design of hollow mesoporous silicas (HMSs) has attracted considerable interest due to their structural versatilities for applications in catalysis, adsorption and separation, electrochemical energy storage, drug delivery and controlled release [Citation7–12]. To date, enormous efforts have been dedicated to obtaining HMSs, such as templating, spray-drying and template-free strategies [Citation13–16].
Developing nanoparticles with anisotropic or hierarchically porous morphologies/structures is of great importance for multifunctional applications, but precise engineering of the external morphology and internal structure is still a significant challenge. Notably, there are various factors regulating morphology and structure of HMSs, which are mainly divided into two lines: formulation (e.g. altering the solvents/co-solvents, the ratio of surfactant template to silica source, reaction concentrations or adding the auxiliary agents) and processing parameters (e.g. pH values, temperature, stirring speed, reaction time, hydrothermal treatment or ageing) [Citation17–22]. For example, Fanizza, E. et al. demonstrated different key factors for homogenous and two-phase system in size tuning, suggesting that distinct mechanisms applied in the formation of MSNs were related to the reaction media [Citation18]. Therefore, a variety of HMSs, such as core–shell, multi-shell, multi-hollow, multi-compartment, hollow-dendritic, flower-shaped, paper-ball, hollow spheres with ordered/disordered pores, Janus-type nanoparticles, one-dimensional hollow tubes and cubes, have been reported [Citation23–31]. For instance, Chen, A. Z. et al. impregnated copper/iron in the silica walls to alter mesoporous architectures in sphero-ellipsoid shape due to the significant charge forces [Citation32]. Wang, X. F. et al. reported a novel swelling-double penetration mechanism, in which the number and location of hollow cavities were adjusted by varying the solution polarity of primary emulsion [Citation33]. He, J. et al. used TEOS to control polymer assemblies in solution, allowing continuous morphological transition from spherical micelles to vesicles and eventually to large compound vesicles [Citation34]. Interestingly, Ulrich Wiesner et al. proposed an epitaxial growth mechanism to produce MSNs containing both cubic and hexagonally structured compartments within one particle [Citation35]. Generally, driving forces in the traditional co-condensation process is the creation of an organized texture and the formation of an inorganic network during steady-state growth and the key is to tune the interaction between the solvent, template, auxiliary agents and precursors at the inorganic–organic hybrid surface. In this work, cocoon-shaped hollow mesoporous silica ellipsoids (C-HMSs) were reported, exhibiting high structural stability and effective diffusivity. However, the synthetic theories used thus far hardly elucidate the growth of the peculiar wave-pattern surface texture.
Impressively, inspired by nonequilibrium crystal growth with complex patterns and diffusion-limited morphological stability discussed by Ben-Jacob and W.W. Mullins for explanation of unusual natural architectures (Supplemental Materials for detail) [Citation36–44], we put forward a secondary-templating mechanism based on the nonequilibrium deposition/diffusion of self-assembled silicate-surfactant rod-like micelles around the emulsion surface to understand the physical–chemical process in the formation of C-HMSs. In contrast to the conventional primary-templating method, this mechanism built upon interfacial destabilization dynamics allows a new understanding of the rational design of special hollow mesostructures with promising applications in catalysis, drug delivery, absorption, energy storage and controlled release, because it not only provides novel material structures, new insights into the role of emulsion droplets in morphology evolution but also extends the design of other hollow mesoporous silicas with unique patterns.
Materials and methods
C-HMSs were synthesized via a surfactant-directing self-assembly process [Citation45,Citation46]. In this study, cetyltrimethylammonium bromide (CTAB) was used as a cationic surfactant, and tetraethyl orthosilicate (TEOS) served as the silica source, while 1,3,5-trimethylbenzene (TMB) was used as an auxiliary chemical, and ammonia (NH4OH) served as an alkaline catalyst [Citation47]. Reactions were performed at 353 K, and the synthesis conditions are presented in Table . The morphology and structure of the products changed with varying reaction compositions. As a typical run (Sample 6), the synthetic procedures were as follows: (1) 205 mL of NH4OH (25 wt.% solution) was mixed with 270 mL of distilled water; (2) 2.0 g of CTAB was dissolved into the solution with stirring and heating until the solution became homogeneous; (3) then 6 mL of TMB was added to the solution under stirring for approximately 10–15 min at 353 K; (4) 10 mL of TEOS was slowly added, forming a white slurry, and the above mixture was stirred for another 2 h; (5) afterward, the mixture was filtered, washed with distilled water at least three times, dried in an electric oven at 333 K and then calcined in a muffle furnace at 823 K for 5 h.
Table 1. Starting material compositions and properties of the products.
Results
Figure clearly reveals the characteristic morphologies/microstructures of the resulting materials. Here, the molar ratio of CTAB, TEOS and TMB is kept constant at 1:8:8, whereas the volume amounts of NH4OH and H2O are altered to observe the variation in the overall morphologies of silica samples prepared under different solution concentrations. MCM-41-type mesoporous silica rods maintaining highly ordered hexagonal arrays of pores were produced (Sample 6), revealing four distinct peaks corresponding to the diffraction of (100), (110), (200), (210); the rods were 0.2–1m in length and 0.1–0.2
m in width as shown in Figure (B, b). Comparatively, MCM-41-type mesoporous silica spheres with hexagonal mesoscopic order were observed (Sample 7) as the volume proportion of NH4OH and H2O increased to 1.5 times of the original. The diameters of these spheres were approximately 200 nm as shown in Figure (A, a). The morphology evolution from hexagons to rods and spheres can be interpreted by the joint behavior of silicate-surfactant micelles and pore-expanding agents in the alkaline solution. First, ion exchange occurs between Br- on the rod-like surfactant micelles and OH- on the hydrolysed silica source to form silicate-surfactant micelles (CTAD4R, double-four-ring,
). Second, TMB preferentially dissolves into the hydrophobic region of the silicate-surfactant micelles (CTAD4R@TMB) to increase the volume ratio of the micelles. Afterward, the self-deposition of free rod-like micelles (the dominant factor in the single-phase region) occurs, giving rise to highly ordered MCM-41-type mesoporous silicas with hexagonal pore arrangements after the removal of the surfactant as shown in Figure (b).
Figure 1. (A-G) SEM images and (a-g) TEM images of Sample 7 to Sample 1. (H) Comparison of FT-IR Spectrum between Sample 1, 4 and 6. (h) Effect of solution concentration to average particle size of the resulting samples.
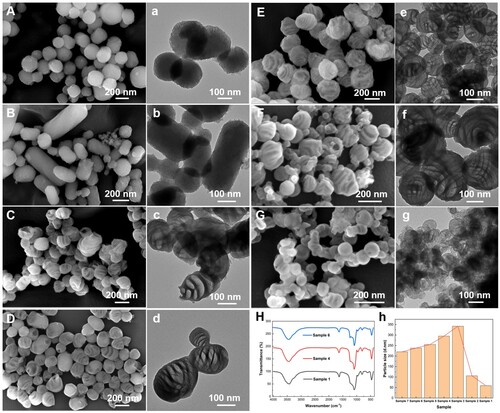
Figure 2. (a) Self-assembly and related behavior of CTAB, TEOS, and TMB in solution. The formation mechanism of mesoporous silicas in (b) dilute solution and (c) concentrated solution.
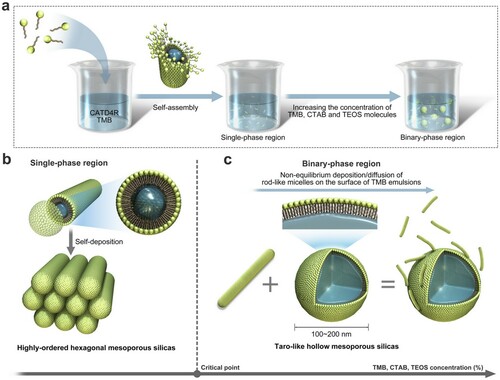
Impressively, as the volume proportion of NH4OH and H2O was reduced to 3/4, 1/2, 1/3, 1/4 of the original, an intriguing phenomenon appears. As indicated in Figure (C-F, c-f), the C-HMSs were obtained with approximately 200 nm in the long axis and 100 nm in the short axis and quasiperiodic variations in surface texture were clearly observed in the electron microscope images. However, as the volume proportion of NH4OH and H2O was reduced to 1/8 of the original, needle-like pores with a few taro-like stripe phases were observed in Figure (G, g). As shown in Figure , the pore structure and particle size distribution have changed greatly, whereas the chemical composition remains -Si-O-Si- framework shown in FT-IR comparison (Figure (H)). Figure (c) demonstrates the formation mechanism of C-HMSs. First, with the simultaneous increase in the concentration of TMB, CTAB and TEOS molecules, the biphasic region dominates from the perspective of solution thermodynamics [Citation18,Citation48], where independent oil droplets composed of TMB molecules are randomly dispersed in the solution. More importantly, the deposition of CTAD4R@TMB micelles on the hydrophobic surface of oil droplets with the hydrophobic end inward is more likely to occur in the concentrated solution than self-deposition because of the potential barrier. Therefore, 1,3,5-trimethylbenzene plays the following two crucial roles in the solution: (1) as pore-expanding agents inside CTAD4R micelles forming CTAD4R@TMB micelles and (2) as secondary templates in the binary phase allowing CTAD4R@TMB micelles to deposit. As a result, driven by electrostatic interactions and external perturbations, the nonequilibrium deposition/diffusion of CTAD4R@TMB micelles on the surface of TMB emulsions (the dominant factor in the binary phase region) occurs to assemble C-HMSs with disordered mesopores. The specific mechanism is further elucidated by interfacial destabilization dynamic and discussed in detail in the next section.
Figure 3. (a, e) XRD patterns, (b, f) N2 adsorption-desorption isotherms, (c, g) the corresponding BJH-adsorption pore size distribution curves and (d, h) particle size distribution curves of the resulting materials: (a-d) MCM-41-type mesoporous silicas in dilute solution and (e-h) cocoon-shaped hollow mesoporous silicas in concentrated solution.
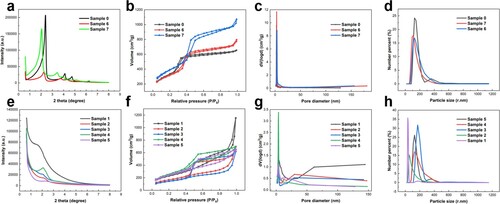
The nanostructure of cocoon-shaped hollow silica ellipsoids with disordered mesopores obtained in the concentrated surfactant solution endows the materials with excellent adsorption and penetration properties. As a result, these nanoparticles can be utilized as ‘nanoreactors’, providing independent reaction zones for guest molecules to achieve high-efficiency, controllable reactions and fast diffusion, and have potential applications as catalysts, sorbents, and controlled drug-delivery/release agents.
Discussion
Compared with Mullins’ theory of diffusion-controlled particle growth (Supplemental Materials for detail), the deposition/diffusion behavior of CTAD4R@TMB micelles at the secondary phase interface bear forms similar to it, whereupon a similar procedure can be followed as indicated in Equation (S2-S4). The principal approximations used are as follows: (1) independent oil droplets composed of TMB molecules are randomly dispersed in the solution with a radius of and are susceptible to deformation under perturbation; (2) the concentration of micelles satisfies Laplace’s equation (Equation Equation(1)
(1)
(1) ); (3) the diffusion velocity
is described by Fick's 1st law of diffusion determined by the diffusion coefficient D and concentration gradient
(Equation Equation(2)
(2)
(2) ); (4) we assume the uniform concentration of micelles far from the oil-droplet surface to be
, the position-dependent concentration of micelles at a general interface to be
and the equilibrium value at a flat interface to be
(Equation Equation(3)
(3)
(3) ), where
is surface curvature,
is capillary constant. Thus, the concentration of micelles on the interface (
) during the perturbation varies according to the following equations:
(1)
(1)
(2)
(2)
(3)
(3)
On the basis of this theoretical understanding, the unique morphology patterns in concentrated solution could be explained as follows. In the case of excessive surfactant concentration (Sample 1), CTAD4R@TMB micelles deposit/diffuse without orientation because of the space constraints of the TMB emulsion surface, leading to the aperiodic needle-like morphology with a few taro-like stripe phases shown in Figure (G, g). In the case of a moderately supersaturated solution (Samples 2–5), there is sufficient space on the surface of the TMB emulsion for the deposition and diffusion of CTAD4R@TMB micelles, thus, orientational deposition/diffusion occurs, leading to the quasi-periodic cocoon-shaped morphology shown in Figure (C-F, c-f).
Considering a two-dimensional facet on the spherical TMB-oil droplet initially, the micelles are deposited on the surfaces of oil droplets through electrostatic and other interactions (Figure (a)), and in the absence of perturbation, rod-like micelles are oriented and uniformly distributed in the solution (Figure (b)). However, the surface of the TMB-oil droplet is susceptible to deformation from external perturbations and gradually expands from spheres to ellipsoids, inducing curvature change. As a soft substrate, changes in the surface curvature will correspondingly induce changes in the interface micelle concentration as described in Equation Equation(3)(3)
(3) . Thus, in the two-dimensional case, we consider the linearized evolution of the initial sphere of radius
perturbed by a simple harmonic wave
to describe this interfacial disturbance (Figure (c)). The interfacial shape of the distorted sphere (
) follows Equation Equation(4)
(4)
(4) according to Ben-Jacob and Mullins-Sekerka instability theory [Citation39,Citation40], where
is the distortion coefficient of simple harmonic upon Fourier expansion related to the value of
and
represents different modes of perturbations, as follows:
(4)
(4)
The question of interfacial stability is determined based on whether the perturbation grows or decays. Assuming the micelle concentration near the interface satisfies the dilute solution theory, so the variation in interfacial micelle concentration with surface morphology still satisfies Equation Equation(3)
(3)
(3) . Therefore, the rate of growth in the perturbation amplitude over time
for the two-dimensional case is given by Equation Equation(5)
(5)
(5) , as follows:
(5)
(5)
where
is the micelle concentration on the undistorted surface. It can be observed that
is related to the concentration gradient and capillary condition, in which the positive former proportional to
is the concentration gradient that favors harmonic growth, whereas the negative latter proportional to
is the capillary condition that favors harmonic decay. Overall, both the gradient effect and capillary effect are present in the system, and the perturbation amplitude depends on the dominant factor. Only if
is positive, which means the value in the brackets of Equation Equation(5)
(5)
(5) is positive, will the harmonic perturbation grow, that is, there will be interfacial instability. Conversely, all higher harmonics that do not satisfy Equation Equation(6)
(6)
(6) will finally vanish. The value of
satisfies the following relationship where
is the critical nucleation radius (constant,
):
(6)
(6)
Then, the critical value of stable growth (
) under different modes of perturbations is given by Equation Equation(7)
(7)
(7) , as follows:
(7)
(7)
Importantly, it has been proven from the Fourier transform that the behavior of an arbitrary two-dimensional infinitesimal perturbation can be obtained by superposition of harmonic spectra since the distortions we have considered are linear. Therefore, when perturbations contain modes that satisfy the critical condition (Equation Equation(6)
(6)
(6) ), the amplitude of the perturbation can be increased. Specifically, when the radius of independent oil droplets (
) is greater than the critical value (
) under certain modes of perturbation (
), the perturbation amplitude grows over time; that is, nonequilibrium deposition and diffusion of the micelles on the interface occurs and is accompanied by a change in the interfacial shape and micelle concentration (Equation Equation(4)
(4)
(4) and Equation Equation(3)
(3)
(3) ) as shown in Figure (b-d). Thus, due to the changes in the shape of the emulsion and the micelle concentration fluctuations caused by simple harmonic perturbation based on interfacial destabilization dynamics, silicate-surfactant micelles vary from a single-rooted state to an aggregation state of periodic function on the interface, leading to the ultimate cocoon-shaped morphology.
Figure 4. The nonequilibrium deposition/diffusion behavior of rod-like micelles on the emulsion surface, supposing a two-dimensional facet on the spherical emulsion subject to simple harmonic perturbation .
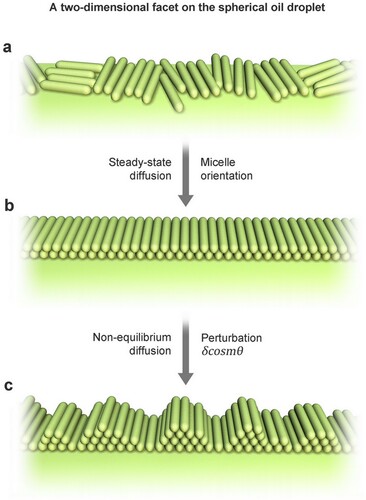
Figure 5. Representative comparison between (a-d) theoretical simulations based on Equation Equation(4)(4)
(4) (
,
) and (e) experimental results. Representative theoretical simulations of the morphology on the zOx plane with (a) a steady-state diffusion of
, simple harmonic perturbation of (b)
, (c)
, and (d)
.
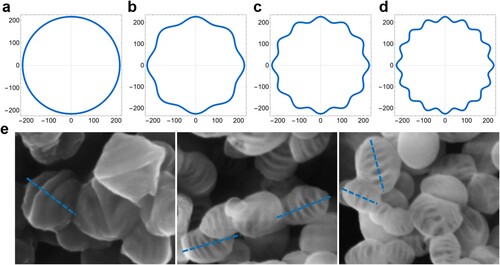
Moreover, the conclusion can be extended to the whole emulsion surface, that is, the two-dimensional facet can be rotated around the center of the ellipsoids to gain a three-dimensional spherical or ellipsoid morphology. The theoretical simulation results built upon Equation (1–7) under different modes of perturbation are consistent with the experimental results, in which values of ultimately correspond to the number of stripes of the unique cocoon-shaped morphology shown in Figure . It should be noted that only in the case of a high but suitable surfactant concentration does the two-dimensional isotactic nonequilibrium deposition/diffusion occur, leading to the quasi-periodic structure during perturbations.
Therefore, there are three types of micelle behavior in the solution. Excessive concentrations of CTAB, TMB and TEOS lead to an oil phase, in which micelles are deposited on the emulsion surface without orientation, forming a disordered needle-like morphology at large. A moderately supersaturated solution leads to a binary phase, in which the deposition/diffusion of micelles on the surface of oil droplets is orientational, nonequilibrant and controlled by interfacial destabilization dynamics forming the cocoon-shaped morphology. Instead, a dilute solution leads to a single aqueous phase, in which the self-deposition of micelles occurs, forming highly ordered hexagonal mesopores.
Conclusion
In conclusion, we have successfully developed a flexible secondary-templating strategy to synthesize cocoon-shaped hollow mesoporous silica ellipsoids by employing TMB as the pore-expanding agent as well as the secondary template in an ammonia solution. Remarkably, this methodology allows the external morphology and interior structure to be regulated from spheres and rods with hexagonal ordered pores to hollow ellipsoids with cocoon-shaped surfaces by varying the concentrations of TEOS, CTAB and TMB. Moreover, this quasiperiodic wave-pattern surface endows the materials with the advantages of high structural stability and high effective diffusivity in one single unit because of the quasiperiodic variation in the shell thickness, exhibiting great application value in many fields, such as catalysis, adsorption, drug delivery and energy storage.
More importantly, unlike the traditional equilibrium-dependent particle growth governed by Gibbs-Thompson Theorem [Citation49], the nonequilibrium interfacial destabilization dynamic mechanism has been reported for the first time based on the deposition/diffusion behavior of rod-like micelles on the surface of droplets influenced by perturbations to elucidate this quasiperiodic cocoon-shaped (wave-pattern) morphology. Excessive TMB droplets serving as secondary soft templates in solution are susceptible to perturbation and deformation, resulting in changes in the interfacial morphology, as well as the micelle concentration at the interface. The theoretical simulation results well explain the morphological adjustment, which is in good agreement with the experimental results. This strategy can be used as a potential exploration for rationally designing hollow structures with diverse morphologies in the future, giving an important reference significance for future research on materials morphology and structure control. It also allows new insight into the role of emulsion droplets during morphology evolution.
Supplemental Material
Download MS Word (741.5 KB)Acknowledgements
We gratefully acknowledge the guidance of Professor Hui Wu, Ph.D. from State Key Lab of New Ceramics and Fine Processing, School of Materials Science and Engineering, Tsinghua University, Beijing 100084, China.
Disclosure statement
No potential conflict of interest was reported by the author(s).
Additional information
Funding
References
- Zhu YL, Liang YJ, Liu SQ, et al. Fabrication of morphology predictable nanomaterials by leveraging mesoporous silica as fabrication reactors. Micropor Mesopor Mat. 2018;258:189–196.
- Soltani R, Marjani A, Shirazian S. Novel mesoporous crumpled paper-like silica balls. Mater Lett. 2020;281:4.
- Qi J, Qin B, Liu J, et al. MCM-41 single crystal of hexagonal circular bicone with pseudo-singular surface and morphogenesis. Crystengcomm. 2011 2011;13(14):4666–4675.
- Zhu FJ, Fang HK, Liu W, et al. Helical mesoporous organic-inorganic hybrid silica nanofibers prepared using a TPE-based silane for explosive detection. Mater Lett. 2022;306:4.
- Wang YB, Zhang BL, Ding XP, et al. Dendritic mesoporous organosilica nanoparticles (DMONs): Chemical composition, structural architecture, and promising applications. Nano Today. 2021;39:23.
- Jiang X, Cui MS, Zhang W, et al. Design of chiral mesoporous silica nanorods using ursodeoxycholic acid/ chenodeoxycholic acid and CTAB as templates for chiral-selective release of achiral drugs. Mater Lett. 2021;285:4.
- Yilmaz MS. Graphene oxide/hollow mesoporous silica composite for selective adsorption of methylene blue. Micropor Mesopor Mat. 2022;330:13.
- Xue ML, Liu XB, Wu H, et al. Construction of Cu2+-doped CeO2 nanocrystals hierarchical hollow structure and its enhanced photocatalytic performance. J Mater Sci-Mater Electron. 2021;32(23):27576–27586.
- Liu T, Qu YH, Liu JH, et al. Core-shell structured C@SiO2 hollow spheres decorated with nickel nanoparticles as anode materials for lithium-ion batteries. Small. 2021;17(49):10.
- He XL, Hao Y, Chu BY, et al. Redox-activatable photothermal therapy and enzyme-mediated tumor starvation for synergistic cancer therapy. Nano Today. 2021;39:12.
- Ji YZ, Ma S, Lv SQ, et al. Nanomaterials for targeted delivery of agrochemicals by an all-in-one combination strategy and deep learning. ACS Appl Mater Interfaces. 2021;13(36):43374–43386.
- Du QQ, Liu Q. ROS-responsive hollow mesoporous silica nanoparticles loaded with Glabridin for anti-pigmentation properties. Micropor Mesopor Mat. 2021;327:10.
- Bao Y, Shi CH, Wang T, et al. Recent progress in hollow silica: Template synthesis, morphologies and applications. Micropor Mesopor Mat. 2016;227:121–136.
- Li YS, Shi JL. Hollow-Structured mesoporous materials: chemical synthesis, functionalization and applications. Adv Mater. 2014;26(20):3176–3205.
- Wu SH, Mou CY, Lin HP. Synthesis of mesoporous silica nanoparticles. Chem Soc Rev. 2013;42(9):3862–3875.
- Xiao N, Lu XH, Yan WJ, et al. Self-template synthesis of hollow nano-spherical nickel silicate/nickel sulfide composite and its application for lithium-sulfur battery. Mater Lett. 2021;300:4.
- Kankala RK, Wang SB, Chen AZ. Nanoarchitecting hierarchical mesoporous siliceous frameworks: A new way forward. iScience. 2020;23(11):101687.
- Rizzi F, Castaldo R, Latronico T, et al. High surface area mesoporous silica nanoparticles with tunable size in the sub-micrometer regime: Insights on the size and porosity control mechanisms. Molecules. 2021;26(14):4247.
- Kankala RK, Zhang H, Liu CG, et al. Metal species–encapsulated mesoporous silica nanoparticles: Current advancements and latest breakthroughs. ADV FUNCT MATER. 2019;29(43):1902652.
- Wang YB, Du X, Liu Z, et al. Dendritic fibrous nano-particles (DFNPs): rising stars of mesoporous materials. J Mater Chem A. 2019;7(10):5111–5152.
- Ban QF, Zheng YC, Qin YS, et al. Co-assembly-driven nanocomposite formation techniques toward mesoporous nanosphere engineering: A review. Micropor Mesopor Mat. 2021;324:32.
- Wang J, Pan M, Yuan J, et al. Hollow mesoporous silica with a hierarchical shell from in situ synergistic soft-hard double templates. Nanoscale. 2020;12(19):10863–10871.
- Tang L, Cheng J. Nonporous silica nanoparticles for nanomedicine application. Nano Today. 2013;8(3):290–312.
- Liu S, Wei M, Rao J, et al. A controlled formation of cage-like nanoporous hollow silica microspheres. Mater Lett. 2011;65(13):2083–2085.
- Zhao B, Collinson MM. Hollow silica capsules with well-defined asymmetric windows in the shell. Langmuir. 2012;28(19):7492–7497.
- Qin D, Chen A, Mamat X, et al. Double-shelled yolk-shell Si@C microspheres based electrochemical sensor for determination of cadmium and lead ions. Anal Chim Acta. 2019;1078:32–41.
- Feyen M, Weidenthaler C, Schüth F, et al. Regioselectively controlled synthesis of colloidal mushroom nanostructures and their hollow derivatives. J. Am. Chem. Soc. 2010;132(19):6791–6799.
- Acar H, Garifullin R, Guler MO. Self-assembled template-directed synthesis of one-dimensional silica and titania nanostructures. Langmuir. 2011;27(3):1079–1084.
- Sun Q, Kooyman PJ, Grossmann JG, et al. The formation of well-defined hollow silica spheres with multilamellar shell structure. Adv Mater. 2003;15(13):1097–1100.
- Shao D, Zhang X, Liu W, et al. Janus silver-mesoporous silica nanocarriers for SERS traceable and pH-sensitive drug delivery in cancer therapy. ACS Appl Mater Interfaces. 2016;8(7):4303–4308.
- Xuan M, Wu Z, Shao J, et al. Near infrared light-powered Janus mesoporous silica nanoparticle motors. J Am Chem Soc. 2016;138(20):6492–6497.
- Liu C-G, Han Y-H, Zhang J-T, et al. Rerouting engineered metal-dependent shapes of mesoporous silica nanocontainers to biodegradable Janus-type (sphero-ellipsoid) nanoreactors for chemodynamic therapy. Chem Eng J. 2019;370:1188–1199.
- Zhao YJ, Lyu HL, Liu YJ, et al. Rational design and synthesis of multimorphology mesoporous carbon@silica nanoparticles with tailored structure. Carbon N Y. 2021;183:912–928.
- Jin L, Liu CH, Cintron D, et al. Structural engineering in the self-assembly of amphiphilic block copolymers with reactive additives: micelles, vesicles, and beyond. Langmuir. 2021;37(32):9865–9872.
- Suteewong T, Sai H, Hovden R, et al. Multicompartment mesoporous silica nanoparticles with branched shapes: an epitaxial growth mechanism. Science. 2013;340(6130):337–341.
- Ben-Jacob E, Garik P. The formation of patterns in nonequilibrium growth. Nature. 1990;343(6258):523–530.
- Ben-Jacob E, Godbey R, Goldenfeld N, et al. Experimental demonstration of the role of anisotropy in interfacial pattern formation. Phys Rev Lett. 1985;55(12):1315–1318.
- Li J, Shi Y, Cai Q, et al. Patterning of nanostructured cuprous oxide by surfactant-assisted electrochemical deposition. Cryst Growth Des. 2008;8(8):2652–2659.
- Mullins WW, Sekerka RF. Morphological stability of a particle growing by diffusion or heat flow. J Appl Phys. 1963;34(2):323–329.
- Sander LM, Ramanlal P, Ben-Jacob E. Diffusion-limited aggregation as a deterministic growth process. Phys Rev A Gen Phys. 1985;32(5):3160–3163.
- Cristini V, Lowengrub J. Three-dimensional crystal growth - I: linear analysis and self-similar evolution. J Cryst Growth. 2002;240(1–2):267–276.
- Cristini V, Lowengrub J. Three-dimensional crystal growth—II: nonlinear simulation and control of the Mullins–Sekerka instability. J Cryst Growth. 2004;266(4):552–567.
- Li S, Lowengrub JS, Leo PH, et al. Nonlinear stability analysis of self-similar crystal growth: control of the Mullins–Sekerka instability. J Cryst Growth. 2005;277(1–4):578–592.
- Li S, Lowengrub J S, Leo P H, et al. Nonlinear theory of self-similar crystal growth and melting. J Cryst Growth. 2004;267(3–4):703–713.
- Cai Q, Luo ZS, Pang WQ, et al. Dilute solution routes to various controllable morphologies of MCM-41 silica with a basic medium. Chem Mat. 2001;13(2):258–263.
- Cai Q, Lin WY, Xiao FS, et al. The preparation of highly ordered MCM-41 with extremely low surfactant concentration. Micropor Mesopor Mat. 1999;32(1–2):1–15.
- Mizutani M, Yamada Y, Nakamura T, et al. Anomalous pore expansion of highly monodispersed mesoporous silica spheres and its application to the synthesis of porous ferromagnetic composite. Chem Mat. 2012;20(14):4777–4782.
- Cai Q, Geng Y, Zhao X, et al. Morphological classification of mesoporous silicas synthesized in a binary water-ether solvent system. Micropor Mesopor Mat. 2008;108(1–3):123–135.
- Holman JP. Thermodynamics. 3rd ed. New York: McGraw-Hill; 1980.