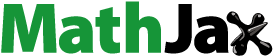
ABSTRACT
A self-supported electrode was designed and prepared for the first time with CeO2 nanoparticles decorated on porous Ni–Fe bimetallic phosphide nanosheets, which were grown on high-conductivity Ni foam. Owing to the strong electronic interactions between the heterostructure interfaces and the introduction of oxygen vacancies, such an electrode displays outstanding oxygen evolution reaction performance with an overpotential of 188 mV at 10 mA cm–2 and the electrolyzer using the electrode as both cathode and anode exhibits a cell voltage of 1.54 V at 10 mA cm–2.
GRAPHICAL ABSTRACT

IMPACT STATEMENT
The oxygen vacancy engineering strategy was easily achieved by electrodeposition of CeO2 and it can be extended to the design of other high-efficient and low-cost electrocatalysts.
1. Introduction
The electrocatalytic water splitting has emerged as a hydrogen production technology with advantages of high-purity products and zero carbon emissions, thus attracting much attentions [Citation1–3]. Generally speaking, overall water splitting consists of two half-reactions: the oxygen evolution reaction (OER) at the anode and the hydrogen evolution reaction (HER) at the cathode, and a larger operating voltage is required relative to the theoretical limit of 1.23 V [Citation4,Citation5]. At present, noble-metal Pt-based catalysts have been considered to be the most advanced HER catalysts, while RuO2 and IrO2 exhibit remarkable performance for OER [Citation6,Citation7]. Nevertheless, high expense, scarcity, and poor durability severely restrict their commercialization. It is thus necessary to explore electrocatalysts, which possess low cost, high activity and excellent durability, to replace the precious-metal ones. Particularly, bifunctional electrocatalysts with high activity for both reactions are more beneficial for practical applications because of the superiorities of simplifying the equipments and lowering the cost [Citation8]. Therefore, it is extremely important to develop efficient bifunctional electrocatalysts for both HER and OER.
In recent years, transition-metal phosphides (TMPs) are widely reported as effective catalysts for HER owing to their hydrogenase-like catalytic mechanism [Citation9–11]. Meanwhile, recent studies also indicate that TMPs also display excellent catalytic performance for OER, making them potential candidates for high-performance bifunctional electrocatalysts [Citation6,Citation12,Citation13]. More important, it has been reported that doping other metal elements can be an effective method to enhance the catalytic activity of TMPs owing to the adjustment of electronic structure and local coordination environment [Citation8,Citation14]. Hence, bimetallic phosphides usually show much superior catalytic activity compared to the monometallic ones [Citation15,Citation16]. However, TMPs generally exhibit disappointing long-term stability during the operation of OER as a result of corrosion and oxidation. Their catalytic activity also needs further improvement.
Recently, some researches have reported that the catalytic performance can be improved by adding co-catalysts [Citation17,Citation18]. Cerium oxide (CeO2) is a significant rare earth oxide, and it possesses abundant reserves, reversible surface oxygen ion exchange, good electronic or ionic conductivity, and high oxygen-storage capacity [Citation5,Citation19,Citation20]. The flexible transition between Ce3+ and Ce4+ of CeO2, which is conducive to the gain and loss of electrons, not only offers the opportunity to generate strong electronic interactions at the heterointerfaces between CeO2 and other substances, but also promotes the formation of oxygen vacancies (VO). Thus, numerous active sites are easy to generate [Citation21–24]. Besides, owing to the remarkable anti-corrosion ability, CeO2 could be beneficial for distinctly enhancing the stability of electrocatalysts [Citation4,Citation25]. Accordingly, the electrocatalytic performance of TMP-based materials may be improved via the efficient strategy of CeO2 decoration on bimetallic phosphides.
Based on the above considerations, a self-supported electrode was designed and prepared for the first time with CeO2 nanoparticles decorated on porous Ni-Fe bimetallic phosphide nanosheets, which were grown on high-conductivity Ni foam (denoted as CeO2/NiFeP/NF). Such an electrode combines the following advantages: (i) the decorated CeO2 nanoparticles can regulate the interface and enrich VO, promote the strong electronic interactions between NiFeP nanosheets and CeO2 nanoparticles, and thus improve the catalytic activity; (ii) CeO2 has the unique property of high oxygen storage capacity, which can immediately assimilate O2 generated during the reaction, thus promoting the process of OER; and (iii) the high conductivity and porous structure of NiFeP nanosheets greatly facilitate the transfer of electron and mass transport.
2. Experimental procedures
The self-supported CeO2/NiFeP/NF hybrid electrode was fabricated through electrodeposition and the following phosphorization. Firstly, NiFe layered double hydroxide nanosheets were grown on Ni foam (NiFe LDH/NF) by using a potentiostatic electrodeposition method. Next, CeO2 nanoparticles were deposited on NiFe LDH/NF (CeO2/NiFe LDH/NF) via a galvanostatic electrodeposition route [Citation26]. Finally, CeO2/NiFe LDH/NF was converted to CeO2/NiFeP/NF through a thermal phosphorization procedure [Citation27]. (a) schematically shows the preparation process. For a comparison, the NiFeP/NF electrode was also fabricated through a similar process without the electrodeposition of CeO2. Please see Supporting Information for more details.
Figure 1. (a) Schematic illustration of the preparation of CeO2/NiFeP/NF. (b) SEM, (c) TEM, and (d) HRTEM images of CeO2/NiFeP. The inset of (c) shows a particle size distribution analysis. (e) XRD pattern of CeO2/NiFeP. (f) N2 adsorption/desorption isotherms and pore size distribution (the inset) of CeO2/NiFeP. (g) EPR spectra of CeO2/NiFeP and NiFeP.
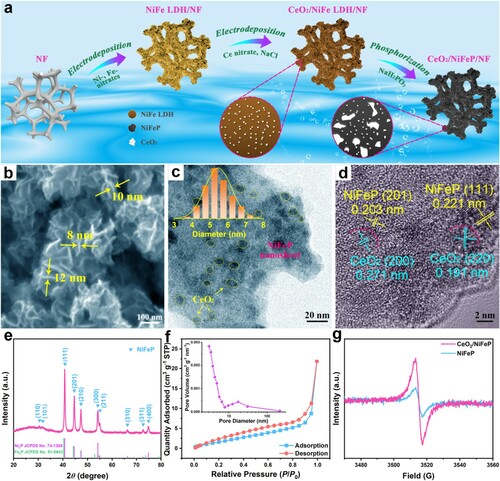
3. Results and discussion
The morphology of samples was investigated by scanning electron microscopy (SEM) and transmission electron microscopy (TEM) (see Figures (b,c), and S1–3). In (b), a close observation illustrates that CeO2/NiFeP still maintains the morphology of nanosheet (the thickness of ∼10 nm). These nanosheets are interconnected and stacked, thus generating open porous structure, which is favorable for exposing rich active sites and accelerating the mass transport [Citation28,Citation29]. In (c), it can be found that numerous CeO2 nanoparticles with the average diameter of ∼5 nm are uniformly distributed on CeO2/NiFeP nanosheets. (d) presents the high-resolution TEM (HRTEM) image of CeO2/NiFeP. Thereinto, the lattice distances of 0.203 and 0.221 nm are indexed to (201) and (111) planes of NiFeP, as well as 0.191 and 0.271 nm correspond to (220) and (200) planes of CeO2, respectively. Figure S4 shows that Ni, Fe, Ce, O, and P elements are uniformly distributed in the whole CeO2/NiFeP nanosheets. The crystal structure of samples was investigated by X-ray diffraction (XRD) (see Figures (e), S5, and S6). In (e), the XRD pattern shows diffraction peaks of NiFeP, which are nearly identical to those of Ni2P (JCPDS No. 74-1385) and Fe2P (JCPDS No. 51-0943). The characteristic peaks of CeO2 do not appear in the XRD pattern since their intensity is too weak, which may be caused by the small size, low crystallinity, and low content of CeO2 nanoparticles [Citation18]. After partially enlarging the XRD pattern, the faint peaks of CeO2 can be observed (see Figure S7). The Fourier transform infrared (FTIR) spectra of the samples show that the peak corresponding to the M–O (M = Ni or Fe) bond blue-shifts from 532 to 510 cm−1 after the introduction of CeO2 (see Figure S8). This indicates that the M–O bonds may be partly replaced by the Ce–O bonds at the interface between NiFeP and CeO2 for the formation of heterostructure [Citation30–33]. The specific surface area and pore size distribution of CeO2/NiFeP can be determined via Brunauer-Emmett-Teller (BET) analysis. From the inset of (f), the pore size distribution indicates that both mesopores (2∼50 nm) and macropores (> 50 nm) exist in CeO2/NiFeP. The smaller pores mainly account for the shrinkage of the nanosheets during the phosphating process, and simultaneously the generation of the larger pores is caused by the overlapping or cross-linking of nanosheets [Citation34,Citation35]. Such a porosity facilitates gas diffusion and electrolyte permeation during the water splitting, which is beneficial for enhancing the catalytic performance of the CeO2/NiFeP/NF electrode [Citation36–38]. Particularly, electron paramagnetic resonance (EPR) measurements were used to detect the concentration of VO. In (g), the signal at g = 2.003 is caught distinctly in CeO2/NiFeP, revealing the presence of VO. These results are attributed to the flexible conversion between Ce3+ and Ce4+, and it is favorable for the gain and loss of electrons, thus promoting the formation of VO [Citation39].
The chemical valence states and surface compositions of CeO2/NiFeP/NF and NiFeP/NF were investigated by X-ray photoelectron spectroscopy (XPS). Figures and S9 present the corresponding results. After the introduction of CeO2, the characteristic peak of Ni2+ shifts to a more positive binding energy (∼0.2 eV) and the peak assigned to Feδ+ also shifts to a higher binding energy (∼0.3 eV) (see (a,b)). These results demonstrate that strong electronic interactions exist between Ni/Fe and Ce atoms, which could promote the electrocatalytic activity [Citation17,Citation40,Citation41]. The Ce 3d spectrum (see (c)) discloses the coexistence of Ce3+ and Ce4+ in CeO2/NiFeP [Citation42], which is due to the unique 4f electronic properties of CeO2, whose cations can flexibly transform between the Ce3+ and Ce4+ oxidation states. Notably, the amount of Ce3+ increases significantly in CeO2/NiFeP compared with pure CeO2 nanoparticles, which can introduce abundant VO [Citation43], further confirmed by the O 1s spectrum (see (d)) and Raman spectrum (see Figure S10). The defective O peak caused by VO cannot be found in NiFeP, which implies that abundant VO are generated by depositing CeO2. Rich VO can promote the adsorption of water molecules by the catalytic materials, improve the conductivity of the catalysts, as well as reduce the activation energy of water splitting reaction, further optimizing the electrocatalysis performance [Citation20,Citation23,Citation44,Citation45]. However, excessive VO may cause the damage of the catalysts structure and the lower valence state of the nearby metal cation, which is not conducive to further improvement of the catalytic performance of transition-metal materials. Therefore, the introduction of an appropriate amount of VO via the hybridization of NiFeP and CeO2 species should be a rational strategy to improve the OER activity by the oxygen vacancy engineering. Herein, the concentration of VO can be regulated by different contents of CeO2 (see Figure S11).
Figure 2. High-resolution XPS spectra of (a) Ni 2p, (b) Fe 2p, (c) Ce 3d, and (d) O 1s for CeO2/NiFeP/NF and NiFeP/NF.
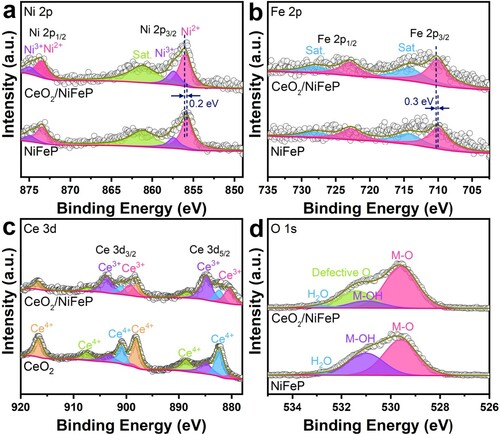
Electrochemical measurements were performed to test the OER performance with a typical three-electrode system in 1 M KOH electrolyte. To explore the optimal CeO2 content, different electrodeposition times (300, 600, and 900 s) were used for the galvanostatic electrodeposition of CeO2 nanoparticles. The obtained samples are donated as CeO2/NiFeP/NF (300 s), CeO2/NiFeP/NF, and CeO2/NiFeP/NF (900 s), respectively. Table S1 shows the CeO2 contents of these samples determined by inductively coupled plasma-optical emission spectroscopy (ICP-OES). As expected, the CeO2/NiFeP/NF electrode owns the highest activity (see Figure S12). (a) shows that the CeO2/NiFeP/NF electrode displays a smaller overpotential of 188 mV at 10 mA cm–2 compared with those of the NiFeP/NF (196 mV), CeO2/NiFe LDH/NF (215 mV), NiFe LDH/NF (225 mV), RuO2/NF (308 mV), and NF (393 mV) electrodes. These results indicate that both phosphorization and CeO2 modification play important roles in improving the OER activity. Besides, the catalytic activity of electrocatalysts can also be assessed by Tafel slope. The small Tafel slope generally represents the fast reaction kinetics [Citation46,Citation47]. From (b), the CeO2/NiFeP/NF electrode shows a lower Tafel slope of only 32 mV dec–1 compared with other electrodes (40, 44, 49, and 99 mV dec–1 for the NiFeP/NF, CeO2/NiFe LDH/NF, NiFe LDH/NF, and NF electrodes, respectively). Moreover, the charge transfer ability of various electrodes was investigated by the electrochemical impedance spectroscopy (EIS) tests (see Figure S13). In order to find out the origin of the advantageous OER activity of the CeO2/NiFeP/NF electrode, the electrochemical surface area (ECSA) was derived from the double layer capacitance (Cdl) [Citation48,Citation49]. Herein, the Cdl values of various electrodes (see (c)) are gained via measuring cyclic voltammetry (CV) curves (see Figure S14) at different scan rates in the nonfaradic region. The results indicate that phosphorization brings a larger ECSA, thereby exposing more abundant active sites to the electrolyte. Furthermore, the specific activity of the CeO2/NiFeP/NF electrode is 0.8 mA c, which is 5.2 times higher than that of the NiFeP/NF electrode at the potential of 1.43 V (see Figure S15). The turnover frequencies (TOFs) of the obtained electrodes were calculated [Citation50,Citation51] and the corresponding results are shown in Figure S16. These demonstrates that CeO2 decoration can enhance the intrinsic activity of active sites. Figure S17 displays the multi-current step curves of the CeO2/NiFeP/NF electrode to study its steady-state activity. Moreover, the potentials of the CeO2/NiFeP/NF electrode change a little from 1.47 to 1.49 V after 400 h electrolysis test in (d), revealing its superior long-term stability. Owing to the self-supported structure without the introduction of insulative polymer binders, the CeO2/NiFeP/NF electrode could withstand violent gas evolution, prevent the agglomeration of active materials, and facilitate fast electron transfer and mass transport [Citation52,Citation53]. The SEM and TEM images show that the nanosheet structure of CeO2/NiFeP is still kept after such a long-term stability test. From Figure S18, the theoretical and experimental O2 generation amounts are almost consistent, which enables the faradaic efficiency of the CeO2/NiFeP/NF electrode to be as high as 98%. All these electrochemical measurement results demonstrate that the CeO2/NiFeP/NF electrode has outstanding performance towards OER in alkaline electrolyte, superior to most of the reported transition metal-based electrocatalysts (see Figure S19 and Table S2).
Figure 3. (a) Polarization curves of the CeO2/NiFeP/NF, NiFeP/NF, CeO2/NiFe LDH/NF, NiFe LDH/NF, RuO2/NF, and pure NF electrodes. (b) The corresponding Tafel plots. (c) Capacitive current densities at −0.25 V (vs. Hg/HgO) plotted against scan rates. (d) Chronopotentiometric test of the CeO2/NiFeP/NF electrode at a current density of 50 mA cm−2. The insets of (d) are SEM and TEM images of CeO2/NiFeP after the durability test. (e) Polarization curves of the CeO2/NiFeP/NF||CeO2/NiFeP/NF electrolyzer and the Pt/C/NF||RuO2/NF electrolyzer with a scan rate of 1 mV s–1 in 1 M KOH.
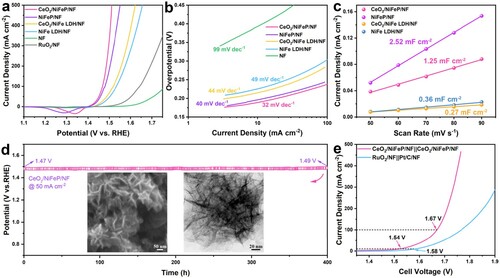
According to the previous studies [Citation7,Citation14,Citation54], the TMP-based materials can efficiently catalyze both HER and OER in alkaline media, which could serve to be potential bifunctional catalysts for water splitting and has great promise for further applications in fuel cell. As expected, the CeO2/NiFeP/NF electrode displays excellent HER performance in alkaline media (see Figure S20). To evaluate the water splitting catalytic performance of the self-supported CeO2/NiFeP/NF electrode, the electrolyzer (denoted as CeO2/NiFeP/NF||CeO2/NiFeP/NF) using it as both cathode and anode was measured. In (e), the CeO2/NiFeP/NF||CeO2/NiFeP/NF electrolyzer displays an excellent activity with cell voltages of 1.54 V to drive 10 mA cm–2 and 1.67 V to drive 100 mA cm–2, outperforming those of Pt/C/NF||RuO2/NF (1.58 and 1.77 V) and most of the reported transition metal-based bifunctional catalysts (see Figure S21 and Table S3). Besides, the CeO2/NiFeP/NF||CeO2/NiFeP/NF electrolyzer also shows excellent stability (see Figure S22).
To understand the nature behind, CeO2/NiFeP/NF after the OER durability test (marked as post-OER) was further investigated. Generally, metal phosphides always in situ form insulative oxides/hydroxides on their surface after the OER process, and they are considered to be the true OER active sites [Citation52,Citation55,Citation56]. In (a), the XRD pattern of the post-OER sample is compared with that of the original one (marked as initial). Except for the characteristic peaks of NiFeP, the peaks of NiFe LDH are also observed. Moreover, the HRTEM image (see Figure S23), the elemental mapping images (see Figure S24), the ICP-OES results (see Table S4), and the XPS results (see Figure S25) also confirm this point. In (b), the signal intensity of VO becomes a little weaker after 400 h OER test. Even so, there are still a larger number of VO in the post-OER sample than those in NiFeP, which ensures high activity during the OER process. After the OER test, the Ce 3d spectrum shows that the ratio of Ce4+:Ce3+ obviously increases, indicating that a part of Ce3+ was oxidized to Ce4+ under the OER condition (see (c)). In O 1s spectrum (see (d)), the intensity of the defective O peak of the post-OER sample relatively decreases compared to the initial sample, which indicates the reduction of VO.
Figure 4. (a) XRD pattern of the post-OER sample. (b) EPR spectra of CeO2/NiFeP before and after the durability test. High-resolution XPS spectra of (c) Ce 3d, (d) O 1s, for the post-OER sample.
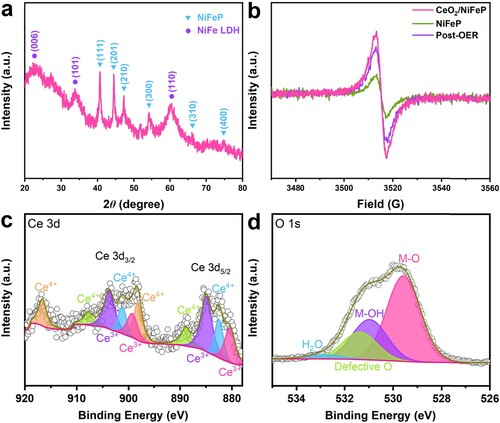
To disclose the mechanism behind the OER, DFT calculations were implemented by VASP 6.1.0. The most stable configurations of NiOOH and NiFeOOH are shown in Figure S26. Here, the Ni atoms on the surfaces of NiOOH and NiFeOOH were considered the active sites (donated as Nisite and Fe–Nisite, respectively, see Figure S27). After introducing Fe dopant, Fe–Nisite exhibits better catalytic activity than Nisite, with a lower overpotential (η) of 0.64 V (see Figure S28). Conversely, NiFeP possesses a higher η of 1.32 V, indicating that the oxidized species are the key to enhance the OER performance (see Figure S29). To further clarify the effect of Ce species in CeO2/NiFeP/NF, the free energies changes on Ce-doped NiFeOOH were calculated (see (a,b)). Particularly, one oxygen atom on the surface was removed to simulate the oxygen vacancy. Therefore, this vacancy was considered as the active site (donated as Ce–Nisite, see Figure S27). Furthermore, as a contrast, the catalytic activity of CeO2 (111) plane was also calculated. It was found that Ce–Nisite owns a much lower η of 0.34 V than that of the CeO2 (111) plane (η = 0.91 V), which is even better than Fe–Nisite (η = 0.64 V). What is more, the value of ΔGO*–ΔGOH* is often chosen as the OER descriptor [Citation37]. (c) shows that the Ce–Nisite has the lowest value of 1.57 eV, which is close to the optimal value (1.6 eV) [Citation57]. Furthermore, after the addition of Ce, more electrons of the Ni 3d orbitals are observed near the Fermi energy, which means a slightly positive shift of the d-band center (ϵd) (see (d)) [Citation53]. This is hopeful to enhance *OH adsorption energy, leading to more favorable OER thermodynamics [Citation58,Citation59].
Figure 5. DFT simulation results. (a) Schematic profile of adsorption atomic configuration on the Ce–Nisite during the OER elementary steps. (b) Free energy diagrams along the reaction pathways on Ce–Nisite, Fe–Nisite and CeO2 (111) plane. (c) Representation of the theoretical overpotential as a function of the difference in O* and OH* adsorption Gibbs energies. (d) PDOS of Ce–Nisite 3d orbitals and Fe–Nisite 3d orbitals. The Fermi energy was set to zero.
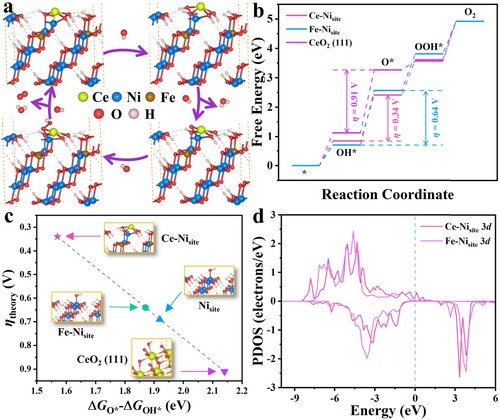
4. Conclusions
Overall, a self-supported CeO2/NiFeP/NF electrode was designed and prepared as efficient bifunctional electrocatalyst, which displays outstanding performance towards OER with a low overpotential of 188 mV at 10 mA cm–2 and Tafel slope of 32 mV dec–1. Besides, the CeO2/NiFeP/NF||CeO2/NiFeP/NF electrolyzer requires only a cell voltage of 1.54 V to drive 10 mA cm–2 for overall water splitting, exceeding the majority of previously reported transition metal-based electrocatalysts. Such superior performance primarily originates from the distinctive properties of CeO2 nanoparticles, which bring the strong electronic interactions between the interfaces of the heterostructure hybrid, and also introduce rich oxygen vacancies. These could be favorable for the conformation of active sites and the improvement of the intrinsic catalytic activity. Furthermore, DFT simulations indicate that Ce species can efficiently regulate the energetics for OER intermediates, resulting in more favorable OER thermodynamics.
Supplemental Material
Download MS Word (4.7 MB)Disclosure statement
No potential conflict of interest was reported by the author(s).
Additional information
Funding
References
- Dresselhaus MS, Thomas IL. Alternative energy technologies. Nature. 2001;414:332–337.
- Seitz LC, Dickens CF, Nishio K, et al. A highly active and stable IrOx/SrIrO3 catalyst for the oxygen evolution reaction. Science. 2016;353:1011.
- Zhang H, Zhou W, Dong J, et al. Intramolecular electronic coupling in porous iron cobalt (oxy)phosphide nanoboxes enhances the electrocatalytic activity for oxygen evolution. Energy Environ Sci. 2019;12:3348–3355.
- Sun H, Tian C, Fan G, et al. Boosting activity on Co4N porous nanosheet by coupling CeO2 for efficient electrochemical overall water splitting at high current densities. Adv Funct Mater. 2020;30:1910596.
- Qiu B, Wang C, Zhang N, et al. CeO2-induced interfacial Co2+ octahedral sites and oxygen vacancies for water oxidation. ACS Catal. 2019;9:6484–6490.
- Yan L, Cao L, Dai P, et al. Metal-organic frameworks derived nanotube of nickel–cobalt bimetal phosphides as highly efficient electrocatalysts for overall water splitting. Adv Funct Mater. 2017;27:1703455.
- Wu L, Yu L, Zhang F, et al. Heterogeneous bimetallic phosphide Ni2P-Fe2P as an efficient bifunctional catalyst for water/seawater splitting. Adv Funct Mater. 2021;31:2006484.
- Lia RQ, Wang BL, Gao T, et al. Monolithic electrode integrated of ultrathin NiFeP on 3D strutted graphene for bifunctionally efficient overall water splitting. Nano Energy. 2019;58:870–876.
- Pan Y, Sun K, Liu S, et al. Core-shell ZIF-8@ZIF-67-derived CoP nanoparticle-embedded N-doped carbon nanotube hollow polyhedron for efficient overall water splitting. J Am Chem Soc. 2018;140:2610–2618.
- Yu J, Cheng G, Luo W. Hierarchical NiFeP microflowers directly grown on Ni foam for efficient electrocatalytic oxygen evolution. J Mater Chem A. 2017;5:11229–11235.
- Ren YC, Li ZR, Deng B, et al. Superior hydrogen evolution electrocatalysis enabled by CoP nanowire array on graphite felt. Int J Hydrog Energy. 2022;47:3580–3586.
- Weng B, Wang X, Grice CR, et al. A new metal–organic open framework enabling facile synthesis of carbon encapsulated transition metal phosphide/sulfide nanoparticle electrocatalysts. J Mater Chem A. 2019;7:7168–7178.
- Guo Y, Tang J, Wang Z, et al. Hollow porous heterometallic phosphide nanocubes for enhanced electrochemical water splitting. Small. 2018;14:1802442.
- Tang C, Zhang R, Lu W, et al. Fe-doped CoP nanoarray: a monolithic multifunctional catalyst for highly efficient hydrogen generation. Adv Mater. 2017;29:1602441.
- Zhang B, Lui YH, Ni H, et al. Bimetallic (FexNi1−x)2P nanoarrays as exceptionally efficient electrocatalysts for oxygen evolution in alkaline and neutral media. Nano Energy. 2017;38:553–560.
- Li J, Yan M, Zhou X, et al. Mechanistic insights on ternary Ni2−xCoxP for hydrogen evolution and their hybrids with graphene as highly efficient and robust catalysts for overall water splitting. Adv Funct Mater. 2016;26:6785.
- Feng JX, Ye SH, Xu H, et al. Design and synthesis of FeOOH/CeO2 heterolayered nanotube electrocatalysts for the oxygen evolution reaction. Adv Mater. 2016;28:4698–4703.
- Gao W, Xia Z, Cao F, et al. Comprehensive understanding of the spatial configurations of CeO2 in NiO for the electrocatalytic oxygen evolution reaction: embedded or surface-loaded. Adv Funct Mater. 2018;28:1706056.
- Xia J, Zhao H, Huang B, et al. Efficient optimization of electron/oxygen pathway by constructing ceria/hydroxide interface for highly active oxygen evolution reaction. Adv Funct Mater. 2020;30:1908367.
- Liu Y, Ma C, Zhang Q, et al. 2D electron gas and oxygen vacancy induced high oxygen evolution performances for advanced Co3O4/CeO2 nanohybrids. Adv Mater. 2019;31:1900062.
- Esch F, Fabris S, Zhou L, et al. Electron localization determines defect formation on ceria substrates. Science. 2005;309:752.
- Zhao D, Pi Y, Shao Q, et al. Enhancing oxygen evolution electrocatalysis via the intimate hydroxide–oxide interface. ACS Nano. 2018;12:6245–6251.
- Li M, Pan X, Jiang M, et al. Interface engineering of oxygen-vacancy-rich CoP/CeO2 heterostructure boosts oxygen evolution reaction. Chem Eng J. 2020;395:125160.
- Kim JH, Shin K, Kawashima K, et al. Enhanced activity promoted by CeOx on a CoOx electrocatalyst for the oxygen evolution reaction. ACS Catal. 2018;8:4257–4265.
- Obata K, Takanabe K. A permselective CeOx coating to improve the stability of oxygen evolution electrocatalysts. Angew Chem. 2018;130:1632–1636.
- Wang X, Yang Y, Diao L, et al. CeOx-decorated NiFe-layered double hydroxide for efficient alkaline hydrogen evolution by oxygen vacancy engineering. ACS Appl Mater Interfaces. 2018;10:35145–35153.
- Wang X, Sun C, He F, et al. Enhanced hydrogen evolution reaction performance of NiCo2P by filling oxygen vacancies by phosphorus in thin-coating CeO2. ACS Appl Mater Interfaces. 2019;11:32460–32468.
- Sun H, Yan Z, Liu F, et al. Self-supported transition-metal-based electrocatalysts for hydrogen and oxygen evolution. Adv Mater. 2019;32:1806326.
- Chen G, Hu Z, Zhu Y, et al. A universal strategy to design superior water-splitting electrocatalysts based on fast in situ reconstruction of amorphous nanofilm precursors. Adv Mater. 2018;30:1804333.
- Ahmad I, Ahmed J, Batool S, et al. Design and fabrication of Fe2O3/FeP heterostructure for oxygen evolution reaction electrocatalysis. J Alloys Compd. 2022;894:162409.
- Xiao FX. Construction of highly ordered ZnO–TiO2 nanotube arrays (ZnO/TNTs) heterostructure for photocatalytic application. ACS Appl Mater Interfaces. 2012;4:7055–7063.
- Jia Y, Zhu L, Pan H, et al. Excellent electrocatalytic hydrogen evolution performance of hexagonal NiCoP porous nanosheets in alkaline solution. Appl Surf Sci. 2022;580:152314.
- Maria Magdalane C, Kaviyarasu K, Judith Vijaya J, et al. Facile synthesis of heterostructured cerium oxide/yttrium oxide nanocomposite in UV light induced photocatalytic degradation and catalytic reduction: synergistic effect of antimicrobial studies. J Photochem Photobiol B. 2017;173:23–34.
- Li X, Bai Y, Wang M, et al. Dual carbonaceous materials synergetic protection silicon as a high-performance free-standing anode for lithium-ion battery. Nanomaterials. 2019;9(4):650.
- Zhang Y, Hui ZX, Zhou HY, et al. Ga doping enables superior alkaline hydrogen evolution reaction performances of CoP. Chem Eng J. 2022;429:132012.
- Cui RC, Xu B, Dong HJ, et al. N/O dual-doped environment-friendly hard carbon as advanced anode for potassium-ion batteries. Adv Sci. 2020;7:1902547.
- Jiang J, Sun F, Zhou S, et al. Atomic-level insight into super-efficient electrocatalytic oxygen evolution on iron and vanadium co-doped nickel (oxy)hydroxide. Nat Commun. 2018;9:2885.
- Bae SH, Kim JE, Randriamahazaka H, et al. Seamlessly conductive 3D nanoarchitecture of core–shell Ni–Co nanowire network for highly efficient oxygen evolution. Adv Energy Mater. 2017;7:1601492.
- Yu Y, Wang X, Gao W, et al. Trivalent cerium-preponderant CeO2/graphene sandwich-structured nanocomposite with greatly enhanced catalytic activity for the oxygen reduction reaction. J Mater Chem A. 2017;5:6656–6663.
- Bose R, Karuppasamy K, Rajan H, et al. Electrodeposition of unary oxide on a bimetallic hydroxide as a highly active and stable catalyst for water oxidation. ACS Sustain Chem Eng. 2019;7(19):16392–16400.
- Liu Z, Li N, Zhao H, et al. Regulating the active species of Ni(OH)2 using CeO2: 3D CeO2/Ni(OH)2/carbon foam as an efficient electrode for the oxygen evolution reaction. Chem Sci. 2017;8:3211–3217.
- Dai ZX, Du XQ, Wang YH, et al. Promoting urea oxidation and water oxidation through interface construction on a CeO2@CoFe2O4 heterostructure. Dalton Trans. 2021;50:12301–12307.
- Li T, Li S, Liu Q, et al. Hollow Co3O4/CeO2 heterostructures in situ embedded in N-doped carbon nanofibers enable outstanding oxygen evolution. ACS Sustain Chem Eng. 2019;7(21):17950–17957.
- Xu L, Jiang Q, Xiao Z, et al. Plasma-engraved Co3O4 nanosheets with oxygen vacancies and high surface area for the oxygen evolution reaction. Angew Chem Int Ed. 2016;55:5277.
- Yang F, Bao X, Li P, et al. Boosting hydrogen oxidation activity of Ni in alkaline media through oxygen-vacancy-rich CeO2/Ni heterostructures. Angew Chem Int Ed. 2019;58:14179–14183.
- Du XQ, Ding YY, Zhang XS. Selectively Se-doped Co3O4@CeO2 nanoparticle-dotted nanoneedle arrays for high-efficiency overall water splitting. Appl Surf Sci. 2021;562:150227.
- Du XQ, Zhang CY, Wang HB, et al. Controlled synthesis of Co9S8@NiCo2O4 nanorod arrays as binder-free electrodes for water splitting with impressive performance. J Alloys Compd. 2021;885:160972.
- Zai SF, Dong AQ, Li J, et al. Low-crystallinity mesoporous NiGaFe hydroxide nanosheets on macroporous Ni foam for high-efficiency oxygen evolution electrocatalysis. J Mater Chem A. 2021;9:6223–6231.
- Luo X, Shao Q, Pi Y, et al. Trimetallic molybdate nanobelts as active and stable electrocatalysts for the oxygen evolution reaction. ACS Catal. 2019;9(2):1013–1018.
- Li J, Chen LX, Liu XX, et al. Eggshell-like MoS2 nanostructures with negative curvature and stepped faces for efficient hydrogen evolution reactions. ACS Appl Nano Mater. 2021;4:14086–14093.
- Benck JD, Chen Z, Kuritzky LY, et al. Amorphous molybdenum sulfide catalysts for electrochemical hydrogen production: insights into the origin of their catalytic activity. ACS Catal. 2012;2:1916–1923.
- Yao RQ, Shi H, Wan WB, et al. Flexible Co–Mo–N/Au electrodes with a hierarchical nanoporous architecture as highly efficient electrocatalysts for oxygen evolution reaction. Adv Mater. 2020;32:1907214.
- Sun JS, Zhou YT, Yao RQ, et al. Nanoporous gold supported chromium-doped NiFe oxyhydroxides as high-performance catalysts for the oxygen evolution reaction. J Mater Chem A. 2019;7:9690–9697.
- Zhou G, Li M, Li Y, et al. Regulating the electronic structure of CoP nanosheets by O incorporation for high-efficiency electrochemical overall water splitting. Adv Funct Mater. 2020;30:1905252.
- Wygant BR, Kawashima K, Mullins CB. Catalyst or precatalyst? The effect of oxidation on transition metal carbide, pnictide, and chalcogenide oxygen evolution catalysts. ACS Energy Lett. 2018;3:2956–2966.
- Zhang YQ, Ouyang B, Xu J, et al. Rapid synthesis of cobalt nitride nanowires: highly efficient and low-cost catalysts for oxygen evolution. Angew Chem. 2016;128:8812–8816.
- Ng JWD, García-Melchor M, Bajdich M, et al. Gold-supported cerium-doped NiOx catalysts for water oxidation. Nat Energy. 2016;1:16053.
- Zai SF, Gao XY, Yang CC, et al. Ce-modified Ni(OH)2 nanoflowers supported on NiSe2 octahedra nanoparticles as high-efficient oxygen evolution electrocatalyst. Adv Energy Mater. 2021;11:2101266.
- Chen J, Li H, Yu Z, et al. Octahedral coordinated trivalent cobalt enriched multimetal oxygen-evolution catalysts. Adv Energy Mater. 2020;10:2002593.