Abstract
Mo2AlB2 is a lamellar transition metal boride that is prepared by selectively etching the MoAlB MAB phase precursor. Most methods for synthesizing Mo2AlB2 require the use of strong acids or bases and a long reaction time. In this study, we present a Lewis acid molten salt method for synthesizing the lamellar structured Mo2AlB2 by selectively etching a layer of aluminium atoms from the MoAlB precursor. The synthesized Mo2AlB2 shows excellent catalytic activity for hydrogen evolution reaction under alkaline conditions, with long-term stability, and a low overpotential of 145 mV and Tafel slope of 76 mV dec−1 at 10 mA cm−2.
GRAPHICAL ABSTRACT
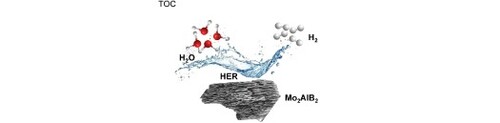
IMPACT STATEMENT
Mo2AlB2 was synthesized through a novel molten salt method of etching MoAlB, resulting in exceptional HER catalytic performance in alkaline conditions.
KEYWORDS:
1. Introduction
With growing concern over the energy crisis and ecological pollution, efforts are being made to develop sustainable and environmentally friendly energy [Citation1–3]. Hydrogen is considered the best alternative to traditional fossil fuels due to its high energy density, abundance, and zero-emission of carbonaceous species [Citation4–6]. Among the current hydrogen production methods, electrochemical water splitting is an environmentally friendly way to produce bulk hydrogen with high purity [Citation7]. The practical application of electrochemical water splitting relies on high-performance catalysts for hydrogen evolution reaction (HER), the half-reaction of water splitting [Citation8–11]. Platinum (Pt) shows excellent electrocatalytic activity on HER, but the scarcity of natural resources and high cost hinder its large-scale applications [Citation12–14]. Therefore, developing non-precious metal electrocatalysts for HER is attracting increasing research interest.
In recent years, 2D metal borides (MBenes) with similar structures to 2D metal carbides and/or nitrides (MXenes) have received increasing research interest. MBenes exhibit excellent electrical conductivity and thus hold great promise for HER [Citation15]. Similar to the preparation of MXene from MAX phase precursor, MBene could be prepared by selectively etching the A-site atoms from ternary transition metal borides (MAB, M is a transition metal, A stands for IIIA or IVA element, and B is boron) precursor [Citation16–18]. Although density functional theory (DFT) calculations have predicted many stable MBenes, only a few types of MBenes have been successfully synthesized [Citation19–23]. The current etching method is to fully or partially etch the A-site element by immersing the MAB phase precursors in an acid or alkali solution, which typically requires a long preparation time [Citation24]. For example, Zhang et al. synthesized 2D CrB by immersing Cr2AlB2 in 0.5 M HCl at room temperature for 7 days [Citation21]. MoAl1-xB of different sizes can be obtained by immersing MoAlB in 10% of aqueous NaOH for 24 h [Citation25]. Lucas T. Alameda et al. used NaOH by partially etching the Al layer in MoAlB single crystals and further investigated the HER activity in acidic electrolytes [Citation26]. It was found that the MoAlB precursor showed low activity towards HER in acidic media. The activity was further improved by partially etching the Al in MoAlB (301 mV vs 400 mV, 10 mA cm−2) [Citation26]. These recent studies provide ideas for exploring the HER of layered borides in alkaline electrolytes.
However, it is challenging to obtain molybdenum-based systems with high specific surface area lamellar structures that have the advantage of high activity and stability [Citation26,Citation27]. Therefore, adopting a green, safe, and short etching route may expand the variety of the lamellar transition metal borides and offer new possibilities for other application studies. Recently, a general Lewis acid molten salt method has been proposed to rapidly synthesize Ti-based MXenes [Citation28,Citation29]. This method is also expected to be applied to the rapid preparation of MBene. To the best of our knowledge, there are no reports about the synthesis of partially etched MABs or MBenes by the molten salt method.
In this work, the Lewis acid molten salt method was proposed for etching the MoAlB precursor to obtain laminated Mo2AlB2 for the first time. When used as the catalyst for HER, Mo2AlB2 shows high catalytic activity under alkaline conditions. At current density of 10 mA cm−2, it delivers low overpotential of 145 mV, suggesting the potential applications as non-precious metal-based electrocatalysts for HER.
2. Materials and methods
2.1 Preparation of Mo2AlB2 powders
MoAlB (400 meshes, purchased from 11 Technology Co., Ltd), KCl, NaCl, and CuCl2, were weighed in a molar ratio of 1:1:1:6, where NaCl and KCl are used as the salt bed, and CuCl2 is the etching agent. Then, the mixture was well mixed and heated, and the etching reaction temperature was investigated in the range of 450–750°C at a rate of 10°C/min for 30 min. The product was obtained after being washed with deionized water to remove the residual salt. In addition, the 1 M ammonium persulphate (APS) solution was stirred for 10 min at room temperature with a magnetic stirrer to remove the copper monomers produced by the reaction. Finally, the product was washed three to four times with deionized water. The final sample was dried in a vacuum oven at 60°C for approximately 12 h.
2.2. Characterization
Phases identification of the synthesized products was characterized using an X-ray diffractometer (XRD, DX-2000) with Cu Kα radiation (λ = 0.15406 nm), and the tube voltage is 40 kV, and the current is 30 mA. Scanning electron microscopy (SEM, JSM-7900F) and energy dispersion spectrometer (EDS, UltimMax65) were used to observe the product's microstructure and analyze the element content and distribution. TEM images were taken by a Talos F200S G2 working at 200 kV. The electronic structure of the samples was analyzed by X-ray photoelectron spectroscopy (XPS, ESCALAB 250Xi) using Kα rays generated from an Al anode target at an energy of 1487 eV, etched at 1 nm/s for 800 s and calibrated using Fermi edge.
2.3 Electrochemical measurements
In brief, the as-prepared catalysts (1 mg) with 40 µL Nafion solution (DuPont, 5 wt%) and then dispersed in a water/ethanol solution (1 mL, 2:1 v/v) were ultrasonically dispersed 30 min to form the homogeneous ink. The obtained catalysts-loaded carbon paper (1 × 1 cm, TORAY) by drop coating with catalyst loading of 1 mg cm−2 acted as the working electrode. A graphite rod and a saturated calomel electrode (SCE) as the counter electrode and the reference electrode, respectively. All potentials were calculated with respect to the reversible hydrogen electrode (RHE) scale according to the Nernst equation (ERHE = ESCE + 0.0591 × pH + 0.241 V, at 25°C) [Citation30]. Linear sweep voltammetry (LSV) polarization curves were measured at a scan rate of 5 mV s−1 and all measured polarization curves were manually 80% iR compensated. The HER polarization curves were corrected by removing iRs from the measured potential, according to the following equation: Ecorrected = Emeasured – iRs80%. Where Ecorrected, Emeasured, and i are the iR-corrected potential, experimental potential measurement, and current, respectively. All the electrochemical measurements are evaluated by a typical three-electrode system in 1 M KOH at an Autolab M204 (Metrohm, Switzerland) electrochemical workstation. Electrochemical impedance spectroscopy (EIS) measurements were applied at an overpotential of open circuit voltages, along with the frequency range from 105 Hz to 10−1 Hz. The electrochemical active surface area (ECSA) can be estimated by the double-layer capacitance (Cdl), which was obtained by linear fitting of the catalyst and was measured by cyclic voltammetry (CV) over a range of non-faraday reaction potentials (0.18–0.23 V vs RHE) at scan rates of 20, 40, 60, 80, 100, 120, 140 mV s−1, respectively. The current density difference plot (ΔJ at 0.205 V vs RHE) was linear with different scan rates. Before measurement, the electrolyte solution was purged with N2 gas (99.999%) to remove O2.
3. Results and discussion
3.1 Materials synthesis and characterization
Figure (a) shows a schematic of the Lewis acid molten salt synthesis process to prepare lamellar Mo2AlB2 under an Ar atmosphere. In brief, the CuCl2/NaCl/KCl salts were mixed with the MoAlB precursor in a certain ratio and heated to the reaction temperature under Ar protection for 30 min, then cooled to room temperature. At the etching temperature, Cu2+ in molten salts oxidized the Al in the MoAlB MAB phase and formed reduced Cu and volatile AlCl4 [Citation31]. The Cu particles generated during the etching process were removed with 1 M ammonium persulphate (APS) solution, and the excess salts were washed with deionized water. The final products were vacuum filtered and dried at 60°C under vacuum for 12 h. Figure (b) depicts the X-ray diffraction (XRD) patterns of the pristine MoAlB precursor and products after etching in molten salt at 450–750°C for 30 min, respectively. After etching at 450°C, the peak intensities of the pristine MoAlB precursor are significantly weakened, but the characteristic peaks of MoAlB remained, indicating the etching is incomplete. When the etching temperature increases to 650°C, peaks of the MoAlB precursor disappear completely, and a new set of diffraction peaks appear. Besides, the (020) diffraction peak at 2θ = 12.64° shifts to 13.56° (Figure S1), suggesting the interlayer distance (dinter) of the precursor changes from 7.0 to 6.5 Å, which explains the Al layer in MoAlB is partial removal that is consistent with the reported XRD pattern of Mo2AlB2 etched from MoAlB [Citation32]. To further determine the purity of the molten salt-derived Mo2AlB2, high-intensity XRD diffraction data were tested for structural refinement of the diffraction data using the Rietveld method (Figure S2), and the cif file for Mo2AlB2 was derived from theoretical calculations (DFT) [Citation33]. The results showed that Mo2AlB2, Mo, and MoAlB were present in the sample at 95.58, 2.78, and 1.64 wt%, respectively, indicating the successful synthesis of Mo2AlB2. A higher etching temperature of 750°C is also explored. The dominant phase of the product after being etched at 750°C belongs to Mo (PDF#97-005-2267), suggesting the higher temperature may destroy the structure of MoAlB or Mo2AlB2. Thus, Mo2AlB2 with a monolayer of Al is synthesized by the Lewis acid molten salt method using CuCl2 as the etchant at 650°C for only 30 min, which is in a much shorter preparation time than 48 h reported in previous work. Moreover, this method also avoids using dangerous high-concentration LiF + HCl solutions [Citation32].
Figure 1. (a) Scheme of molten salt method of Mo2AlB2 in Ar. (b) XRD patterns of pristine MoAlB precursor and products after etched at 450–750°C for 30 min, respectively. (c-f) SEM images of pristine MoAlB precursor and products at different etching temperatures.
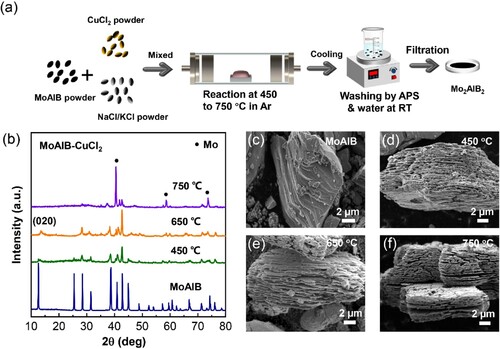
Figure (c–f) displays the SEM images of the pristine MoAlB and the resulting products after etching at different temperatures. The ternary lamellar structure of MoAlB is partially exfoliated during etching and a distinct accordion-like layered pattern can be observed, which aligns with previous research [Citation24]. Additionally, the interlayer expansion and dilatancy degree increase with the increase in etching temperature during the molten salt etching process. The Mo2AlB2 derived from molten salt has a thickness ranging from 20 to 30 nm (Figure S4). The Energy Dispersive Spectra (EDS) and elemental distribution mapping (Table S1 and Figure S3) indicate that the Mo, Al, and B elements are uniformly distributed throughout the particles. The Mo: Al atomic ratio of MoAlB shifts from 1.01:1 to 1.98:1, further evidencing the selective removal of an Al atomic layer from MoAlB to form Mo2AlB2.
The elemental valence and chemical composition of Mo2AlB2 were further determined by X-ray photoelectron spectroscopy (XPS). Figure S6 (a) shows an overview XPS spectrum of the MoAlB precursor (yellow) and Mo2AlB2 (cyan). The signals of Mo, Al, B and O elements can be detected in both MoAlB and Mo2AlB2. A weakening of the Al signal can be clearly observed in the spectrum of Mo2AlB2, indicating a partial etching of Al. Figure S6 (b) shows the high-resolution spectra of Mo 3d of MoAlB, the peaks at 228.22 and 231.45 eV are associated with the Mo-Al-B (3d5/2) and Mo-Al-B (3d3/2) bond [Citation34]. Another two pairs of peaks are attributed to MoO2 (3d5/2 at 229.2 eV) and MoO3 (3d5/2 at 230 eV) [Citation35]. Figure S6 (c) shows the high-resolution spectrum of Mo 3d for Mo2AlB2, obtained after etching at an etching rate of 1 nm/s for 800 s. We find that the binding energy is reduced by nearly 1 eV compared to MoAlB, indicating that the partial etching of Al causes a change in the elemental surface valence state, with peaks at 227.2 eV (3d5/2) and 229.1 eV (3d5/2) are associated with Mo2AlB2 and MoO2. In addition, the high-resolution Al 2p and B 1s spectra are shown in Figure S6 (d and e). The peak area of Al for Mo2AlB2 in Figure S6 (d) is lower than that of MoAlB, also indicating the partial removal of Al. Figure S6 (e) shows the high-resolution spectrum of B 1s for MoAlB and Mo2AlB2, the signals at 188.3 and 188 eV are related to the Mo-Al-B, and Mo2AlB2, respectively [Citation34].
The microstructure of the etched product was further investigated by transmission electron microscopy (TEM). The TEM image of Mo2AlB2 shows a sheet-like multi-layer structure (Figure (a)). From the high-resolution TEM (HRTEM) (Figure (b)) image, a dinter value of 6.1 Å was identified for the etched sample, which is consistent with the XRD result. Additionally, the corresponding SAED pattern (Figure (c)) further confirms the formation of Mo2AlB2. EDS mapping shows Mo, Al, and B elements are uniformly distributed across the flakes. The above characterizations demonstrate that the MoAlB MAB phase precursor with a zigzag double aluminium layer can be transformed to Mo2AlB2 with a single aluminium layer by selective etching of a layer of Al atoms through the Lewis acid melt salt method.
3.2. The HER performance of Mo2AlB2
The electrocatalytic HER performance of the obtained electrocatalysts was evaluated in a three-electrode system configuration using 1 M KOH as the electrolyte. For comparison, the polarization curves of MoAlB, Mo2AlB2, commercial Pt/C (20 wt%, TANAKA), and pure CP were recorded (Figure (a)). The pure CP showed negligible cathodic current densities, implying intrinsically poor catalytic activities toward the HER. The MoAlB precursor shows low activity, while the HER activity of the layered Mo2AlB2 obtained after selective etching is significantly enhanced. The hydrogen precipitation overpotentials of the three catalysts at 10 mA cm−2 are shown in Figure (b). Commercial Pt/C showed the lowest overpotential at 10 mA cm−2, indicating the best catalytic performance. It is worth noting that the overpotential of Mo2AlB2 is much lower than that of MoAlB precursor (145 mV vs 410 mV) when the current density at 10 mA cm−2. It indicates that the layered structure obtained after etching exposed more active sites, significantly improving catalytic performance. We conducted additional experiments on the LSV curves of Pt/C and Mo2AlB2 at a high current of 200 mA/cm2, as illustrated in Figure S8. To provide a clearer representation of the raw data, we chose not to compensate for the iR drop. The results show that Mo2AlB2 outperforms Pt/C at 200 mA/cm2, which can be attributed to its superior catalyst properties and high electrical conductivity. To gain more insight into the HER kinetics of the catalyst after etching, Tafel slopes were calculated as they are traditionally used to evaluate the rate-limiting step of a multi-step HER reaction. Correspondingly, the Tafel slope of the Mo2AlB2 (76 mV dec−1) was notably lower than the MoAlB (219.7 mV dec−1), as seen in Figure (c). The results indicate that laminated Mo2AlB2 shows much faster reaction kinetics, which may be attributed to the partial removal of Al to form etched cavities [Citation36], which enhanced the interfacial reaction kinetics and further improved the catalytic activity.
Figure 3. HER catalytic activity of MoAlB, Mo2AlB2, and commercial Pt/C in N2-saturated 1 M KOH solution at 25°C. (a) Polarization curves, (b) Overpotentials at 10 mA cm−2 of MoAlB, Mo2AlB2 and commercial Pt/C electrocatalysts, and (c) Corresponding Tafel slopes, (d) EIS plot and (e) Calculated electrochemical double-layer capacitance electrocatalyst for MoAlB and Mo2AlB2 electrocatalysts, (f) Stability curves of MoAlB and Mo2AlB2 tested at a constant current density of 10 mA cm−2 without iR compensation.
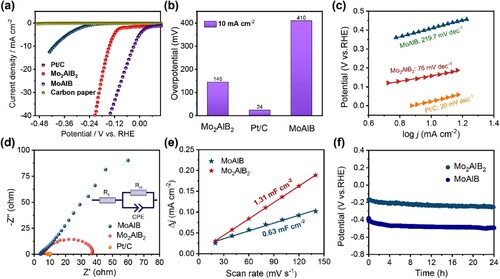
Electrochemical impedance spectroscopy (EIS) tests were carried out at the frequency from 105 to 10−1 Hz to investigate the HER kinetics further. The charge transfer resistances (Rct) were acquired by fitting the Nyquist plots with an equivalent circuit. The EIS plots are shown in Figure (d). The diameter of the semicircle of Mo2AlB2 is much smaller than MoAlB, indicating that the etched layered Mo2AlB2 catalyst has lower charge transfer resistance, which is conducive to the HER reaction.
Additionally, the electrochemical surface areas (ECSAs) of the MoAlB and Mo2AlB2 catalysts were evaluated using the double-layer capacitance (Cdl) as a descriptor that was measured by cyclic voltammetry (CV). Figure S7(a–b) shows the CV curves of MoAlB and Mo2AlB2 electrocatalysts at different scan rates from 20 to 140 mV s−1. The ECSAs of two catalysts were compared based on the double-layer capacitance calculated from the CV curves at different scan rates. Electrochemical double-layer capacitance (Cdl) obtained by linear fitting, with slopes as Cdl value as shown in Figure (e), and the calculated Cdl value of Mo2AlB2 is 1.31 mF cm−2, which is much higher than that of MoAlB (0.63 mF cm−2), indicating the molten salt etching of MoAlB precursor yields Mo2AlB2 with a layered structure exposing more active sites, which in turn effectively expands the electrochemically active region and thus improves the catalytic activity. To assess the stability of Mo2AlB2. Figure (f) shows the long-term stability of Mo2AlB2 at 10 mA cm−2, and it retains good stability after 24 h. The above results indicate that selective etching of a layer of Al atoms from the MoAlB MAB phase precursor by the molten salt method results in stable layered Mo2AlB2 with good catalytic activity for HER in an alkaline medium. The HER performance of Mo2AlB2 was compared to that of Mo-based materials and MXenes (as shown in Tables S2 and S3). Mo2AlB2 was found to have a low overpotential of 145 mV at 10 mA cm−2, which is lower than most Mo-based materials and MXenes, demonstrating its potential for use in the HER process.
4. Conclusion
In summary, Mo2AlB2 is successfully obtained by selectively etching a layer of Al atoms from the MoAlB MAB phase precursor via a Lewis acid molten salt method. SEM and TEM observations showed an accordion-like structure similar to molten salt derived multi-layered MXene. The HER electrocatalytic performance of Mo2AlB2 in 1 M KOH solution is significantly improved compared to MoAlB. It's believed that the interfacial interactions between the layers of Mo2AlB2 make its activity in alkaline electrolytes superior to that of unetched MoAlB, and its layered structure increases the electrochemically active specific surface area of the catalyst and reduces the charge transfer resistance. This work provides an effective strategy for etching other MAB phases and demonstrates the great promise of layered transition metal boride materials in electrocatalysis.
Supplemental Material
Download MS Word (1.6 MB)Disclosure statement
No potential conflict of interest was reported by the author(s).
Additional information
Funding
References
- Lei Y, Wang Y, Liu Y, et al. Designing atomic active centers for hydrogen evolution electrocatalysts. Angew Chem Int Ed. 2020;59:20794–20812.
- Zhao R, Li Q, Jiang X, et al. Interface engineering in transition metal-based heterostructures for oxygen electrocatalysis. Mater Chem Front. 2021;5:1033–1059.
- Huang T, Shen T, Gong M, et al. Ultrafine Ni-B nanoparticles for efficient hydrogen evolution reaction. Chin J Catal. 2019;40:1867–1873.
- Chen Q, Nie Y, Ming M, et al. Sustainable synthesis of supported metal nanocatalysts for electrochemical hydrogen evolution. Chin J Catal. 2020;41:1791–1811.
- Cheng P-F, Feng T, Liu Z-W, et al. Laser-direct-writing of 3D self-supported NiS2/MoS2 heterostructures as an efficient electrocatalyst for hydrogen evolution reaction in alkaline and neutral electrolytes. Chin J Catal. 2019;40:1147–1152.
- Gao Y, Wu Y, He H, et al. Potentiostatic electrodeposition of Ni–Se–Cu on nickel foam as an electrocatalyst for hydrogen evolution reaction. J Colloid Interf Sci. 2020;578:555–564.
- Tiwari JN, Dang NK, Sultan S, et al. Multi-heteroatom-doped carbon from waste-yeast biomass for sustained water splitting. Nat Sustain. 2020;3:556–563.
- Zhou F, Sa R, Zhang X, et al. Robust ruthenium diphosphide nanoparticles for pH-universal hydrogen evolution reaction with platinum-like activity. Appl Catal B: Environ. 2020;274:119092.
- Li M, Pan X, Jiang M, et al. Interface engineering of oxygen-vacancy-rich CoP/CeO2 heterostructure boosts oxygen evolution reaction. Chem Eng J. 2020;395:125160.
- Zhou W, Wu M, Li G. Rambutan-like CoP@ Mo-Co-O hollow microspheres for efficient hydrogen evolution reaction in alkaline solution. Chin J Catal. 2020;41:691–697.
- Gao Y, Zhao Y, Liu H, et al. N, P-doped carbon supported ruthenium doped Rhenium phosphide with porous nanostructure for hydrogen evolution reaction using sustainable energies. J Colloid Interf Sci. 2022;606:1874–1881.
- Popczun EJ, McKone JR, Read CG, et al. Nanostructured nickel phosphide as an electrocatalyst for the hydrogen evolution reaction. J Am Chem Soc. 2013;135:9267–9270.
- Lu Y, Zhu N, Yin F, et al. Biomass-derived heteroatoms-doped mesoporous carbon for efficient oxygen reduction in microbial fuel cells. Biosens Bioelectron. 2017;98:350–356.
- Wang N, Bo X, Zhou M. Laser conversion of biomass into porous carbon composite under ambient condition for pH-Universal electrochemical hydrogen evolution reaction. J Colloid Interf Sci. 2021;604:885–893.
- Zhou S, Yang X, Pei W, et al. MXene and MBene as efficient catalysts for energy conversion: roles of surface, edge and interface. J Phys Energy. 2020;3:012002.
- Ade M, Hillebrecht H. Ternary borides Cr2AlB2, Cr3AlB4, and Cr4AlB6: The first members of the series (CrB2)nCrAl with n=1, 2, 3 and a unifying concept for ternary borides as MAB-phases. lnorg Chem. 2015;54:6122–6135.
- Zhang H, Dai F-z, Xiang H, et al. Crystal structure of Cr4AlB4: A new MAB phase compound discovered in Cr-Al-B system. J Mater Sci Technol. 2019;35:530–534.
- Music D, Schneider JM. The correlation between the electronic structure and elastic properties of nanolaminates. J Org Chem. 2007;59:60–64.
- Jiang Z, Wang P, Jiang X, et al. MBene (MnB): a new type of 2D metallic ferromagnet with high Curie temperature. Nanoscale Horiz. 2018;3:335–341.
- Guo Z, Zhou J, Sun Z. New two-dimensional transition metal borides for Li ion batteries and electrocatalysis. J Mater Chem A. 2017;5:23530–23535.
- Zhang H, Dai F-Z, Xiang H, et al. Phase pure and well crystalline Cr2AlB2: A key precursor for two-dimensional CrB. J Mater Sci Technol. 2019;35:1593–1600.
- Barsoum MW, Radovic M. Elastic and mechanical properties of the MAX phases. Annu Rev Mater Sci. 2011;41:195–227.
- Naguib M, Mashtalir O, Carle J, et al. Two-dimensional transition metal carbides. ACS Nano. 2012;6:1322–1331.
- Jakubczak M, Szuplewska A, Rozmysłowska-Wojciechowska A, et al. Novel 2D MBenes—synthesis, structure, and biotechnological potential. Adv Funct Mater. 2021;31:2103048.
- Alameda LT, Lord RW, Barr JA, et al. Multi-step topochemical pathway to metastable Mo2AlB2 and related two-dimensional nanosheet heterostructures. J Am Chem Soc. 2019;141:10852–10861.
- Alameda LT, Holder CF, Fenton JL, et al. Partial etching of Al from MoAlB single crystals to expose catalytically active basal planes for the hydrogen evolution reaction. Chem Mater. 2017;29:8953–8957.
- Akopov G, Yeung MT, Kaner RB. Rediscovering the crystal chemistry of borides. Adv Mater. 2017;29:1604506.
- Chen J, Jin Q, Li Y, et al. Molten Salt-Shielded Synthesis (MS3) of MXenes in air. Energy Environ Mater. 2022. https://doi.org/10.1002/eem2.12328.
- Liu P, Xiao P, Lu M, et al. Lithium storage properties of Ti3C2Tx (Tx=F, Cl, Br) MXenes. Chin Chem Lett. 2022;34:107426.
- Song H, Wu M, Tang Z, et al. Single atom ruthenium-doped CoP/CDs nanosheets via splicing of carbon-dots for robust hydrogen production. Angew Chem Int Ed. 2021;60:7234–7244.
- Li Y, Shao H, Lin Z, et al. A general Lewis acidic etching route for preparing MXenes with enhanced electrochemical performance in non-aqueous electrolyte. Nat Mater. 2020;19:894–899.
- Kim K, Chen C, Nishio-Hamane D, et al. Topochemical synthesis of phase-pure Mo2AlB2 through staging mechanism. Chem Commun. 2019;55:9295–9298.
- Zhou Y, Xiang H, Zhang H, et al. Theoretical prediction on the stability, electronic structure, room and elevated temperature properties of a new MAB phase Mo2AlB2. J Maters Sci Technol. 2019;35:2926–2934.
- Natu V, Kota SS, Barsoum MW. X-ray photoelectron spectroscopy of the MAB phases, MoAlB, M2AlB2 (M=Cr, Fe), Cr3AlB4 and their binary monoborides. J Eur Ceram Soc. 2020;40:305–314.
- Baltrusaitis J, Mendoza-Sanchez B, Fernandez V, et al. Generalized molybdenum oxide surface chemical state XPS determination via informed amorphous sample model. Appl Surf Sci. 2015;326:151–161.
- Alameda LT, Moradifar P, Metzger ZP, et al. Topochemical deintercalation of Al from MoAlB: stepwise etching pathway, layered intergrowth structures, and two-dimensional MBene. J Am Chem Soc. 2018;140:8833–8840.