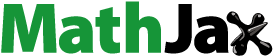
Abstract
Studying the flow behavior is critical to understand the deformation mechanism of amorphous solids. However, detecting the basic flow events in amorphous solids is challenging. Here, by simultaneous SAXS/WAXS, elementary flow carriers in wound metallic glasses are identified from flow-induced structural heterogeneities with a radius of gyration of 2.5∼3.5 nm. Their size increases and morphology changes from sphere-like to rod-like under flow. Moreover, the atomic structure exhibits an unusual change to a more disordered state during winding/annealing at the temperature of ∼0.8 Tg. This work provides an atomic-to-nanoscale description of the flow carriers of amorphous solids during deformation.
Impact Statement
The elementary flow carriers in metallic glasses have a radius of gyration of 2.5∼3.5 nm. During flow, their size increases and morphology changes from sphere-like to rod-like.
GRAPHICAL ABSTRACT
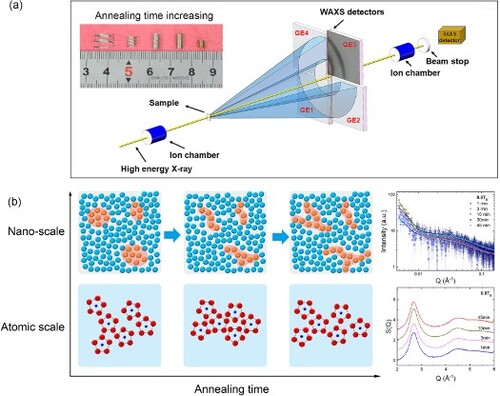
Introduction
The absence of sufficient plasticity is the Achilles’ heel that prevents the broad application of metallic glasses (MGs) [Citation1–3]. MGs often fail catastrophically due to localized plastic deformation via shear banding [Citation4]. Despite decades of research, a comprehensive understanding of the deformation mechanism and flow behavior of MGs is still missing [Citation5–8]. The free volume model [Citation9] was first introduced to explain the inhomogeneous flow behavior in MGs by individual atomic jumps but was unable to fully reveal the actual collective flow processes in MGs [Citation10]. The concept of shear transformation (ST) [Citation11,Citation12], which considers a group of atoms instead of a single atom to accommodate flow, was more successful in interpreting plastic deformation in MGs. Another term, the shear transformation zone (STZ) [Citation13,Citation14], which is different from ST, was latterly introduced to describe the pre-existing site imperfections as flow carriers in interpreting the plastic deformation of amorphous solids. The original STZ model is a simplified description that does not consider the fundamental amorphous structure, which was recently found to contain considerable dynamic and structural heterogeneities at multiple scales [Citation15,Citation16]. For example, elastic heterogeneities in MGs [Citation17] with a volume fraction up to 1/4 that behave like viscous liquid have been proposed on the basis of the results of in situ tensile creep experiments. Significant nanoscale mechanical heterogeneities in MGs were revealed by high-frequency dynamic micropillar tests [Citation18], atomic force acoustic microscopy [Citation19], and dynamic force microscopy [Citation20]. Furthermore, in recent experiments, there have been direct observations of nanoscale short-range and medium-range order [Citation21–24] in MGs, which makes the real microscopic flow mechanism more sophisticated than that described by the STZ model.
ST/STZ concepts [Citation11,Citation13] provided physical pictures of the elementary plastic deformation in amorphous solids and successful theoretical tools for interpreting experiments or simulations [Citation25]. Based on the STZ model, the concept of flow units (FUs) [Citation26–28], representing the regions in a MG with a soft mechanical response, was thus introduced to explain the deformation and flow mechanism. The FUs concept shares general features with the well-recognized ST/STZ models, but the former emphasizes dynamic and structural heterogeneities. FUs can be activated by external stress at elevated temperatures [Citation29,Citation30]. The activation energy for FUs was found to be similar to the flow of viscous liquids and scaled with Tg [Citation31]. It is believed that MGs with a more pronounced β-relaxation peak contain more FUs [Citation26,Citation32]. However, it is still under debate whether the elementary carriers of flow, e.g. FUs, have any specific structural origins [Citation33–36]. Robust experimental evidence linking dynamic heterogeneities with structural heterogeneities in MGs has rarely been reported [Citation28]. The practical difficulties come from two aspects. First, basic flow events exist in very fast time domains and localized regions, making them extremely difficult to control and probe [Citation11,Citation14]. Second, there may be only subtle differences between the atomic structures linked to the flow carriers and the surrounding matrix [Citation37]. Nevertheless, if one could steadily activate flow events and control the volume fraction of FUs [Citation38], then there would be opportunities to capture the structural signatures from the changes in local density and atomic ordering induced by the activation and flow of the FUs. Therefore, by analyzing the multiscale structural information, the relationship between FUs and microstructure could be established.
In this study, a mandrel-winding method [Citation30,Citation38] was employed to control the flow behavior in a Zr50Cu40Al10 MG. By this method, FUs were steadily activated by the stored elastic energy, and homogeneous plastic flow in MGs was achieved, which permitted the accurate study of flow-induced structural change. Through simultaneous high energy synchrotron small- and wide-angle X-ray scattering (SAXS/WAXS) on wound MG ribbons, we discovered the evolution of structural heterogeneities with a radius of gyration of 2.5∼3.5 nm in samples with different states of activated FUs. The size and morphology of the structural heterogeneities were analyzed accordingly. Simultaneously, an abnormal structure change to a more disordered state could be revealed during the winding/annealing process around 0.8 Tg (i.e. around the β-relaxation peak temperature 554 K, corresponding to the characteristic timescale of 1 s) [Citation39], at which most of the FUs were activated [Citation28]. The results of our study provide the first atomic to nanoscale structural information that correlates closely with elementary flow carriers and thus promotes a deeper understanding of the dynamics-structure relationship of MGs.
Materials and methods
Sample preparation
The master Zr50Cu40Al10 alloys were prepared by arc-melting elemental metals with purities above 99.99%. Glassy ribbons with a uniform thickness of ∼22 μm were prepared by a melt-spinning method. The glass transition temperature (Tg = 693 K) was obtained from calorimetric measurements by a Perkin-Elmer DSC 8000 with high-purity standard aluminum crucibles under a constant flow of high-purity argon gas at a heating rate of 20 K/min.
Mandrel winding
The ribbons were helically wound around a stainless steel mandrel with a diameter of 3 mm. The fully wound states were mechanically constrained, placed in a furnace for a period of holding time t at a specific temperature T, and then released from the mandrel. Temperature fluctuations during the experiments did not exceed 0.5 K. In this work, the maximum strain on the surface of the wound ribbon is estimated as ϵ = d/(D−d), where d is the thickness of the ribbon and D is the diameter of the circle formed by helically winding [Citation30]. The initial imposed maximum strain ϵ was 0.728% (within the elastic regime).
Transmission electron microscopy
TEM specimen was made by twin-jet electro-polishing process. High-resolution electron microscopy (HREM) pictures were taken by JEM-F200 TFEG at a flat region and a wound region at the edge of the thinned region in a TEM specimen.
Simultaneous SAXS/WAXS
Simultaneous synchrotron SAXS/WAXS was conducted to probe the multiscale structural information directly through a single X-ray shot in the transmission geometry at beamline 1-ID at the Advanced Photon Source, Argonne National Laboratory, using 70 keV X-rays. The momentum transfer, Q, where Q is the magnitude of the scattering vector defined as Q = 4πsinθ/λ and 2θ is the scattering angle, was in the 0.005-0.168 Å−1 range for SAXS data and the 0.8-7.2 Å−1 range for WAXS data. SAXS data were reduced with data corrected for the background and transmission and transformed onto an absolute scale. The static structure factor, S(Q), was derived from the WAXS data by masking bad pixels, integrating images, subtracting the background, and correcting for oblique incidence, absorption, multiple scattering, fluorescence, Compton scattering, and Laue correction using Fit2D and PDFgetX2.
Results
Homogeneous flow through mandrel-winding
The Zr50Cu40Al10 glassy ribbons show a Tg = 693 K at a heating rate of 20 K/min from DSC results. Four annealing temperatures close to Tg (0.6, 0.7, 0.8 and 0.9 Tg) [Citation38] were chosen during the winding process to ensure significant homogeneous flows in the glassy ribbons at reasonable experimental timescales. The inset in Figure a is a photograph of a wound glassy ribbon annealed at 0.8 Tg with permanent plastic deformation induced by the homogeneous flow of the MG. A schematic diagram of the experimental setup of simultaneous synchrotron SAXS/WAXS [Citation40] is shown in Figure a. The representative SAXS/WAXS profiles are shown in Figure b and c, respectively, and they reveal the nanoscale heterogeneities and atomic structural evolution for the wound Zr50Cu40Al10 MGs. Crystallization is excluded, as no Bragg peaks belonging to crystals are found. For the whole set of SAXS/WAXS profiles, please see Supplementary Figures S1 and S2.
Figure 1. a. Schematic diagram of the setup at beamline 1-ID of the advanced photon source (APS) concurrent with wide-angle X-ray scattering (WAXS) and small-angle X-ray scattering (SAXS). The inset is a photograph of a wound glassy ribbon annealed at 0.8 Tg. Simultaneous b. SAXS and c. WAXS profiles of samples annealed at 0.8 Tg.
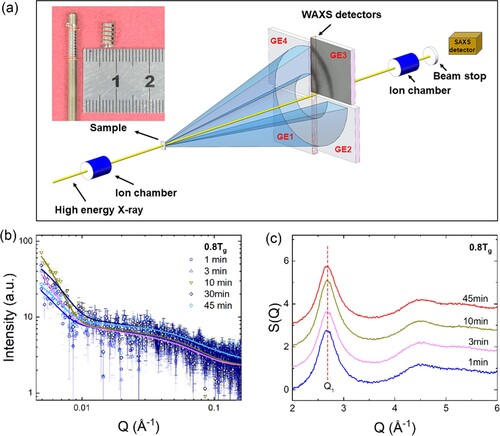
The evolution of the size and morphology of structural heterogeneities
Figure a illustrates different regions in a SAXS profile used to extract nanoscale structural information for a sample. The Guinier region, marked in the blue square, is used to determine the radius of gyration Rg of structural heterogeneities. Moreover, the power law region (in the brown square) is used to describe the geometry, and the Porod region (in the red square) shows the surface fractal dimensions for structural heterogeneities [Citation41]. In the Guinier region, the radius of gyration Rg is obtained by plotting the logarithm of the intensity vs. Q2, , where I0 is the extrapolated zero-angle intensity and Q is the magnitude of the scattering vector defined as Q = 4πsinθ/λ where 2θ is the scattering angle and λ is the wavelength of incident X-rays. The Rg values of all wound samples are shown in Figure b and have a value from approximately 2.5–3.5 nm. For samples annealed at 0.6 and 0.7 Tg, Rg increases gradually during annealing. However, when the temperature approaches the β-relaxation peak temperature (∼0.8 Tg) [Citation30,Citation42], Rg decreases upon annealing. As Rg depends on both the volume and shape of the structural heterogeneities [Citation41,Citation43], the underlying mechanism of its evolution is discussed later. In the power law region, where I(Q) is plotted with Q-DM, the DM parameter reflects the mass fractal dimension of the structural heterogeneities [Citation44]. DM values of 1, 2, and 3 correspond to random or disordered rods, disks, and spheres [Citation44]. As shown in Figure c, the overall trend of DM decreased during annealing at all temperatures. Among all the results, the DM value for samples annealed at 0.8 Tg was the lowest. It decreased gradually to 1, which reflected the morphological change of the local heterogeneities from sphere-like to rod-like. In a Porod analysis, the surface fractal dimension, PS (as summarized in Figure c), also showed that the interface between the structural heterogeneity and matrix became smoother, especially at 0.8 Tg. For samples annealed at 0.9 Tg, we could not obtain the whole trend of structural evolution due to the limited annealing time. Nevertheless, Rg and the morphology of structural heterogeneities in samples annealed at 0.9 Tg showed values similar to those of the samples annealed at 0.8 Tg.
Figure 2. a. Illustration of different regions of SAXS data for analysis. The blue, brown, and red squares illustrate the Guinier region, power-law region, and Porod region, respectively. The red line represents the Guinier fit. b. The radius of gyration Rg. c. The surface fractal dimension, PS (filled symbol), and power law exponent, DM (open symbol), for structural heterogeneities as functions of annealing time during the winding process.
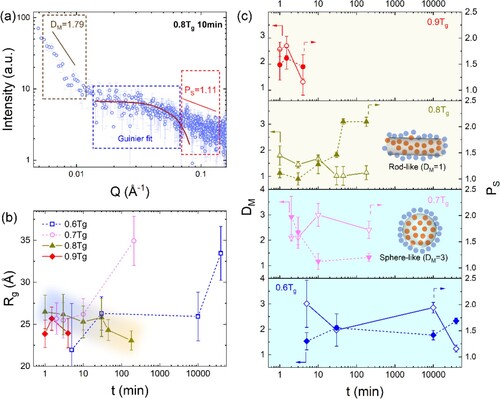
The morphology and size analysis of the structural heterogeneities offered direct evidence for the flow-induced structural evolution, which could be related to the structural origin of the FUs. Transmission Electron Microscopy analysis also observed signs of enhanced nanoscale structural heterogeneities in the wound part of thin regions (see Supplementary Figures S3 and S4). As the annealing temperature and time during the winding process increased, more FUs with higher activation energy started to flow [Citation30], leading to a higher degree of structural change (e.g. atomic ordering or density) along the flow pathway. These results were reflected by the SAXS results for samples annealed at 0.6 and 0.7 Tg, in which the Rg values of the sphere-like structural heterogeneities increased. Notably, the Rg values of the structural heterogeneities decreased slightly in samples annealed at 0.8 Tg and kept the low value at and above 0.8 Tg, although more FUs have been activated [Citation28]. The reason could be that the structural heterogeneities have the strongest tendency to change their shape from sphere-like to rod-like atomic configurations at and above 0.8 Tg (as illustrated by the inset picture in Figure c). The rod-like structure may be achieved through the connection between one end of the flowing atomic cluster and the other, making one dimension elongate and the other dimension shrink to accommodate the macroscopic flow [Citation30]. The resultant shortened dimension of the structural heterogeneities was thus reflected in the decrease in Rg according to the SAXS data [Citation43,Citation44].
Structural evolution at the atomic scale
Detailed structural information at the atomic level of samples with different flow states is obtained from the analysis of the first sharp diffraction peak (FSDP), Q1 (Figure c) [Citation45]. The analysis of moments was often used to avoid the subjectivity of assumed functions for the peak shape of the asymmetric FSDP (refer to the calculation details of moments in the Supplementary Material). It was suggested that the peak position (first moment [Citation46,Citation47]) and width (directly related to the second moment [Citation48]) of the FSDP were linked to the packing density and correlation length for glasses/liquids [Citation22]. The first moments of Q1 of samples annealed at different temperatures are plotted in Figure a. As MGs are thermodynamically metastable, they continually relax to an equilibrium state through the annihilation process of structural defects [Citation49,Citation50], resulting in densification, which is reflected in the increase in Q1 during annealing (ρ∝Q1D). This was consistent with the data for samples annealed below 0.8 Tg. The decrease in the second moment of Q1 and the increase in the peak height of Q1 (Figure b and c) [Citation45] further indicated that the structure became more ordered for samples annealed below 0.8 Tg. In contrast, the structure at 0.8 Tg returned to the less ordered state after only several minutes of annealing. This abnormal behavior indicated that the mechanism for atomic structural evolution differed for the samples at 0.8 Tg and other temperatures. The structural disordering at 0.8 Tg indicates that FUs with less-ordered atomic structure may be activated by an applied stress and elevated temperature within the experimental time period. It is also noted that the peak heights of Q1 for samples annealed at 0.6 and 0.7 Tg after 10000 mins show the decreasing trend, which may be because of the partial activation of the FUs at the end of the treatment. Therefore, the FU activation may contribute to the decrease in the peak height of Q1 after long time annealing at 0.6 and 0.7 Tg. For samples annealed at 0.9 Tg, the data points were insufficient to reveal the whole structural evolution trend due to the limited time resolution caused by fast dynamics. Thus, they are not discussed.
Discussion
The above SAXS/WAXS results provide comprehensive structural information for the wound MGs annealed at sub-Tg temperatures, where the structural characteristics related to the microscopic flow were captured for the first time by scattering techniques. It has been shown that the study of heterogeneities in metallic glasses may cover a range of orders of both time and length scales [Citation51,Citation52]. For example, hierarchical heterogeneity was found in a Zr-based bulk metallic glass using dynamic modulus mapping on a nanoindentation platform [Citation51]. Specifically, heterogeneities at the nanometer scale would be closely linked to the elementary flow carriers. Liu et al. [Citation20] found that the mechanical heterogeneity of a Zr55Cu30Ni5Al10 metallic glass had a correlation length of ∼2.5 nm. It was [Citation29] proposed that the volume of FUs should be ∼3-6 nm3. Wagner et al. [Citation19] reported a wide distribution of local indentation moduli on a scale less than 10 nm. Cao et al. [Citation53] found that amorphous Pd-Si nanoparticles smaller than 3 nm exhibit liquid-like behaviors. Our SAXS results showed the radius of gyration of the flow-induced structural heterogeneities to be 2.5∼3.5 nm, which was very close to the scale of dynamic heterogeneities in the above previously reported results. At the β-relaxation temperature (0.8 Tg), most of the elementary flow carriers were activated and reached the most considerable volume fraction. This resulted in the enhanced connection of atomic clusters to accommodate the macroscopic flow, which was reflected in the morphological change of the structural heterogeneities from sphere-like to rod-like.
The atomic structural analysis further revealed an order-to-disorder transition of wound samples annealed at approximately the β-relaxation temperature. The structure underwent densification and structure ordering at first, which could be attributed to aging. It has been recognized that aging may increase density and enhance the formation of structural order [Citation54–56]. After approximately 10 min, the structure of samples annealed at the β-relaxation temperature returned to a disordered state, which meant that the MG may have been thermally rejuvenated [Citation57,Citation58] by the stored elastic energy through the winding. Our study indicated that applying slight stress at the β-relaxation temperature should be sufficient to overcome structural aging through the activation of a significant amount of FUs, which change the local atomic order and density within the flow pathways and thus lead to overall structural disordering and rejuvenation.
Based on the simultaneous SAXS/WAXS results, the whole picture of the structural evolution of the atomic flow by the FU model could be depicted, as shown in Figure . As the annealing time increased at sub-Tg temperatures, the wound metallic glass started to flow macroscopically. The macroscopic flow was achieved by the activation and morphology change of the nanoscale FUs as elementary flow carriers. At the atomic scale, there were two competitive processes, structural aging and FU activation. The former made the MG denser and more ordered, while the latter made it disordered. At lower temperatures and shorter times, the aging process dominated, while when the temperature reached 0.8 Tg, the FU activation process gradually took over.
Figure 4. Schematic diagram of structural evolution from atomic to nanoscale for samples annealed at 0.8 Tg based on the FU model.
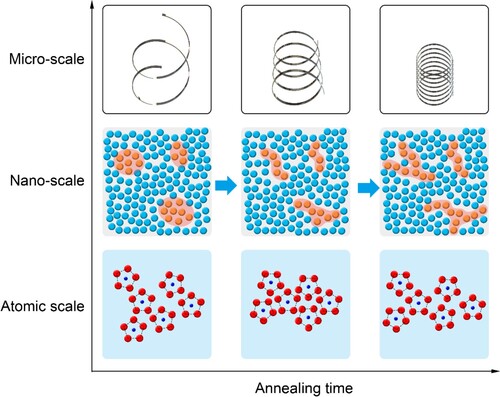
In conclusion, by winding samples into helices and sub-Tg annealing, the flow events in a Zr50Cu40Al10 MG were activated and finely controlled. From simultaneous high energy synchrotron SAXS/WAXS results, we found that the radii of gyration of flow-induced structural heterogeneities were approximately 2.5-3.5 nm, which nondestructively confirmed the scale of dynamic heterogeneities that were reported based on simulations [Citation59], mechanical tests [Citation18–20] and electron microscopy [Citation34,Citation53]. We used the FU perspective to describe the microscopic flow, that is, the collective movement of atomic clusters and their connection from end to end, which resulted in the rod-like morphology of structural heterogeneities. The atomistic structural analysis further determined an abnormal structure change to a state with more disordered regions in samples wound/annealed at around the β-relaxation temperature, which indicated that the MG could be thermally rejuvenated. The transition may have resulted from competition between structural aging and FU accumulation. The observations made in this study will advance our knowledge of the deformation mechanism and flow behavior in MGs and bridge the structure and dynamics of these materials.
Author contributions
S.L., Z.D.W., P.L. X.-L.W., W.H.W. designed the project; S.L. and J.D.A. performed the simultaneous synchrotron SAXS/WAXS experiments; P.L. prepared the samples and performed the mandrel-winding experiments; J.C. G, Z.D.W. and S.L. analyzed the SAXS/WAXS data; all authors reviewed the results, Z.D.W. and S.L. wrote the paper; Z.D.W., S.L., X.L.W. and W.H.W. supervised the overall research activities.
Supplemental Material
Download MS Word (1.7 MB)Acknowledgements
Z.D. Wu and S. Lan acknowledge the support by the Guangdong-Hong Kong-Macao Joint Laboratory for Neutron Scattering Science and Technology. This research used the resources of the Advanced Photon Source, a US Department of Energy (DOE) Office of Science User Facility operated for the DOE Office of Science by Argonne National Laboratory (No. DE-AC02-06CH11357).
Data availability
The data that support the findings of this study are available from the corresponding author on request.
Disclosure statement
No potential conflict of interest was reported by the author(s).
Additional information
Funding
References
- Hufnagel TC, Schuh CA, Falk ML. Deformation of metallic glasses: recent developments in theory, simulations, and experiments. Acta Mater. 2016;109:375–393.
- Leamy H, Wang T, Chen H. Plastic flow and fracture of metallic glass. Metall Mater Trans B. 1972;3(3):699–708.
- Egami T, Iwashita T, Dmowski W. Mechanical properties of metallic glasses. Metals (Basel). 2013;3(1):77–113.
- Greer A, Cheng Y, Ma E. Shear bands in metallic glasses. Mater Sci Eng: R: Rep. 2013;74(4):71–132.
- Schroers J, Johnson WL. Ductile bulk metallic glass. Phys Rev Lett 2004;93(25):255506.
- Yao K, Ruan F, Yang Y, et al. Superductile bulk metallic glass. Appl Phys Lett. 2006;88(12):122106.
- Liu YH, Wang G, Wang RJ, et al. Super plastic bulk metallic glasses at room temperature. Science. 2007;315(5817):1385–1388.
- Demetriou MD, Launey ME, Garrett G, et al. A damage-tolerant glass. Nat Mater. 2011;10(2):123–128.
- Spaepen F. A microscopic mechanism for steady state inhomogeneous flow in metallic glasses. Acta Metall. 1977;25(4):407–415.
- Schall P, Weitz DA, Spaepen F. Structural rearrangements that govern flow in colloidal glasses. Science. 2007;318(5858):1895–1899.
- Argon A. Plastic deformation in metallic glasses. Acta Metall. 1979;27(1):47–58.
- Argon A. Strain avalanches in plasticity. Philos Mag. 2013;93(28-30):3795–3808.
- Falk ML, Langer JS. Dynamics of viscoplastic deformation in amorphous solids. Physical Review E. 1998;57(6):7192.
- Johnson W, Samwer K. A universal criterion for plastic yielding of metallic glasses with a (T/T g) 2/3 temperature dependence. Phys Rev Lett. 2005;95(19):195501.
- Wang WH. Dynamic relaxations and relaxation-property relationships in metallic glasses. Prog Mater Sci. 2019;106:100561.
- Jug G, Loidl A, Tanaka H. On the structural heterogeneity of supercooled liquids and glasses (a). Europhys Lett. 2021;133(5):56002.
- Dmowski W, Iwashita T, Chuang C-P, et al. Elastic heterogeneity in metallic glasses. Phys Rev Lett 2010;105(20):205502.
- Ye J, Lu J, Liu C, et al. Atomistic free-volume zones and inelastic deformation of metallic glasses. Nat Mater. 2010;9(8):619–623.
- Wagner H, Bedorf D, Kuechemann S, et al. Local elastic properties of a metallic glass. Nat Mater. 2011;10(6):439–442.
- Liu Y, Wang D, Nakajima K, et al. Characterization of nanoscale mechanical heterogeneity in a metallic glass by dynamic force microscopy. Phys Rev Lett 2011;106(12):125504.
- Hirata A, Guan P, Fujita T, et al. Direct observation of local atomic order in a metallic glass. Nat Mater. 2011;10(1):28–33.
- Ma D, Stoica AD, Wang X-L. Power-law scaling and fractal nature of medium-range order in metallic glasses. Nat Mater. 2009;8(1):30–34.
- Yang Y, Zhou J, Zhu F, et al. Determining the three-dimensional atomic structure of an amorphous solid. Nature. 2021;592(7852):60–64.
- Lan S, Zhu L, Wu Z, et al. A medium-range structure motif linking amorphous and crystalline states. Nat Mater. 2021;20(10):1347–1352.
- So KP, Stapelberg M, Zhou YR, et al. Observation of dynamical transformation plasticity in metallic nanocomposites through a precompiled machine-learning algorithm. Mater Res Lett. 2022;10(1):14–20.
- Wang Z, Sun B, Bai H, et al. Evolution of hidden localized flow during glass-to-liquid transition in metallic glass. Nat Commun. 2014;5(1):1–7.
- Xue R, Zhao L, Shi C, et al. Enhanced kinetic stability of a bulk metallic glass by high pressure. Appl Phys Lett. 2016;109(22):221904.
- Wang Z, Wang W-H. Flow units as dynamic defects in metallic glassy materials. Natl Sci Rev. 2019;6(2):304–323.
- Liu S, Wang Z, Peng H, et al. The activation energy and volume of flow units of metallic glasses. Scr Mater. 2012;67(1):9–12.
- Luo P, Lu Z, Li Y, et al. Probing the evolution of slow flow dynamics in metallic glasses. Physical Review B. 2016;93(10):104204.
- Yu H, Shen X, Wang Z, et al. Tensile plasticity in metallic glasses with pronounced β relaxations. Phys Rev Lett. 2012;108(1):015504.
- Wang Z, Wen P, Huo L, et al. Signature of viscous flow units in apparent elastic regime of metallic glasses. Appl Phys Lett. 2012;101(12):121906.
- Zhang Z, Ding J, Ma E. Intrinsic shear transformations in metallic glasses. arXiv preprint arXiv:220812661. 2022.
- Zhu F, Song S, Reddy KM, et al. Spatial heterogeneity as the structure feature for structure–property relationship of metallic glasses. Nat Commun. 2018;9(1):1–7.
- Fan Y, Iwashita T, Egami T. How thermally activated deformation starts in metallic glass. Nat Commun. 2014;5(1):1–7.
- Ding J, Patinet S, Falk ML, et al. Soft spots and their structural signature in a metallic glass. Proc Natl Acad Sci USA. 2014;111(39):14052–14056.
- Richard D, Kapteijns G, Giannini JA, et al. Simple and broadly applicable definition of shear transformation zones. Phys Rev Lett 2021;126(1):015501.
- Lu Z, Jiao W, Wang W, et al. Flow unit perspective on room temperature homogeneous plastic deformation in metallic glasses. Phys Rev Lett 2014;113(4):045501.
- Qiao J, Casalini R, Pelletier J-M. Main (α) relaxation and excess wing in Zr50Cu40Al10 bulk metallic glass investigated by mechanical spectroscopy. J Non-Cryst Solids. 2015;407:106–109.
- Wang X-L, Almer J, Liu C, et al. In situ synchrotron study of phase transformation behaviors in bulk metallic glass by simultaneous diffraction and small angle scattering. Phys Rev Lett. 2003;91(26):265501.
- Beaucage G. Approximations leading to a unified exponential/power-law approach to small-angle scattering. J Appl Crystallogr. 1995;28(6):717–728.
- Luo P, Lu Z, Zhu Z, et al. Prominent β-relaxations in yttrium based metallic glasses. Appl Phys Lett. 2015;106(3):031907.
- Guinier A. X-ray diffraction in crystals, imperfect crystals, and amorphous bodies. Courier Corporation; 1994.
- Beaucage G. Small-angle scattering from polymeric mass fractals of arbitrary mass-fractal dimension. J Appl Crystallogr. 1996;29(2):134–146.
- Elliott SR. Origin of the first sharp diffraction peak in the structure factor of covalent glasses. Phys Rev Lett 1991;67(6):711–714.
- Lan S, Blodgett M, Kelton KF, et al. Structural crossover in a supercooled metallic liquid and the link to a liquid-to-liquid phase transition. Appl Phys Lett. 2016;108(21):211907.
- Lan S, Ren Y, Wei XY, et al. Hidden amorphous phase and reentrant supercooled liquid in Pd-Ni-P metallic glasses. Nat Commun. 2017;8(1):14679.
- Dong W, Ge J, Ke Y, et al. In-situ observation of an unusual phase transformation pathway with Guinier-Preston zone-like precipitates in Zr-based bulk metallic glasses. J Alloys Compd. 2020;819:153049.
- Xue RJ, Wang DP, Zhu ZG, et al. Characterization of flow units in metallic glass through density variation. J Appl Phys. 2013;114(12):123514.
- Hodge IM. Physical aging in polymer glasses. Science. 1995;267(5206):1945–1947.
- Tsai P, Kranjc K, Flores KM. Hierarchical heterogeneity and an elastic microstructure observed in a metallic glass alloy. Acta Mater. 2017;139:11–20.
- Cao P, Short MP, Yip S. Understanding the mechanisms of amorphous creep through molecular simulation. Proc Natl Acad Sci USA. 2017;114(52):13631–13636.
- Cao CR, Huang KQ, Shi JA, et al. Liquid-like behaviours of metallic glassy nanoparticles at room temperature. Nat Commun. 2019;10(1):1966.
- Brüning R, Ström-Olsen JO. Atomic displacements during structural relaxation in a metallic glass. Physical Review B. 1990;41(5):2678–2683.
- Deng D, Argon AS, Yip S. Kinetics of structural relaxations in a two-dimensional model atomic glass III. Philos Trans R Soc London Ser A. 1989;329(1608):595–612.
- Ruta B, Baldi G, Monaco G, et al. Compressed correlation functions and fast aging dynamics in metallic glasses. J Chem Phys. 2013;138(5):0054508.
- Ketov SV, Sun YH, Nachum S, et al. Rejuvenation of metallic glasses by non-affine thermal strain. Nature. 2015;524(7564):200–203.
- Meng YH, Zhang SY, Zhou WH, et al. Rejuvenation by enthalpy relaxation in metallic glasses. Acta Mater. 2022;241:118376.
- Krausser J, Samwer KH, Zaccone A. Interatomic repulsion softness directly controls the fragility of supercooled metallic melts. Proc Natl Acad Sci USA. 2015;112(45):13762–13767.