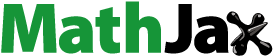
Abstract
The microstructural features of a high-strength body-centered cubic Mg-Li–Al-Ag alloy are investigated and the strengthening mechanisms are discussed. The results show that obvious spinodal decomposition occurs in the alloy. Owing to the diffusion of Al atoms to Ag-vacancy pairs, co-clustering of Al and Ag is observed. The compositional redistribution of Li in the matrix reduces the interfacial lattice misfit and thus lowers the strain energy. Combinatorial spinodal strengthening with coherency strain hardening result in high strength achieved in the Mg-Li–Al-Ag alloy. The dual alloying strategy opens avenues for tuning the microstructure and enhancing mechanical properties of Mg-Li alloys.
GRAPHICAL ABSTRACT
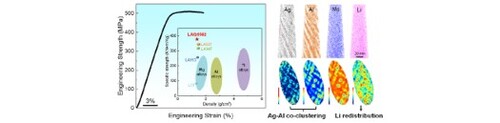
IMPACT STATEMENT
Solutes co-clustering and solvents redistribution are responsible for high strength achieved in ultralight BCC Mg-Li-base alloys, which provide strategy for optimizing mechanical properties in such alloys.
1. Introduction
The development of lightweight materials with high mechanical performance is imperative for the fields of automotive and aerospace industries to increase fuel efficiency and reduce the emissions of greenhouse gases [Citation1–3]. Body-centered cubic (BCC) Mg-Li alloys are among the lightest structural alloys (ρ < 1.5 g/cm3) and possess significantly improved ductility and cold-workability compared to their hexagonal close-packed (HCP) counterparts [Citation4–6]. Although such properties make BCC Mg-Li alloys appealing materials for highly formable and lightweight applications, low strength and microstructural instability become the Achilles’ heel for their diverse use in advanced applications [Citation7].
Aluminium (Al) is the most effective alloying element for increasing the yield strength in BCC Mg-Li based alloys [Citation8]. The strengthening effect due to spinodal decomposition and secondary precipitation of a D03-Mg3Al phase via cluster ordering in BCC Mg-Li–Al alloys has been investigated previously [Citation9,Citation10]. Despite that strength is in direct proportion to Al concentration, there exists a maximum value of Al contents beyond which incoherent AlLi phase forms that cannot provide effective strengthening anymore. Silver (Ag) is not only a precipitation-strengthening phase former in BCC Mg-Li alloys, but also shares the same crystal structure and similar atomic radius with Al [Citation8]. The addition of Ag could strengthen BCC Mg-Li–Al alloy through individual Ag-rich precipitation and/or substitution of Al atoms with Ag in Al-rich clusters/precipitates. Therefore, dual-alloying of Al and Ag in BCC Mg-Li alloys offers the opportunity to solve the above-mentioned problem to further improve mechanical properties. This paper focuses mainly on uncovering the microstructural features and mechanical properties in a BCC Mg-Li–Al-Ag alloy.
2. Material and methods
A Mg-Li based alloy with a composition of Mg-15wt.% Li-6wt.% Al-2wt.%Ag (LAQ1562) was prepared by melting the constituents under inert argon atmosphere in an electrical resistance furnace followed by casting. The as-cast alloys were homogenized for 1 h at 350°C and solution treated for 15 min at 400°C in an Ar-protected tube furnace, followed by rapid quenching. The samples with peak strength are prepared for mechanical testing and microstructural analysis. Cylindrical samples of diameter 10 mm and height 20 mm were prepared from solution-treated alloys. Ambient temperature uniaxial compression testing was carried out on peak-hardness samples using a UTM4304GD electronic universal tensile tester (SUNS, Shenzhen, China) operating at a true strain rate of 1 × 10−3 s−1. Plates of dimensions 5 mm × 5 mm × 3 mm were cut and mechanically polished for X-ray Diffraction (XRD) and Atom Probe Tomography (APT) experiments. Laboratory XRD experiments were carried out on a Bruker-AXS D8 Advance diffractometer, using Ni-filtered Cu Kα radiation to investigate the phases present in the alloys. Each polished sample was adhered to a resin sample holder and aligned with the X-ray beam. The voltage and current were 45 kV and 40 mA, respectively. The 2-theta scan range was 30° to 70°, the scanning resolution was 0.05° per step and the scanning speed was 1° per min. APT samples were prepared by an FEI Helios Plasma focused ion beam (PFIB) with a Xe source. Prior to inserting the samples into the PFIB chamber, they were mechanically polished using an oil-based suspension, then cleaned by pure ethanol. APT measurements were conducted in a Cameca LEAP 5000 XR instrument operating in laser-assisted mode with a pulse rate of 125 kHz, a pulse energy of 40 pJ. The sample temperature during the APT measurements was controlled at 30 K. The commercial software AP suit 6.1 was used for data reconstruction and analyses. As there exists a significant difference of evaporation field between Al/Ag and Li, and Li exhibits much higher probability to be field evaporated outside of the pulses, the deviation of Li concentration is likely induced by preferential evaporation of Li. Before transmission electron microscopic (TEM) testing, thin foils of thickness ∼80 µm were prepared by mechanical polishing followed by ion-milling at −150°C on a Gatan PIPS II Model 695. A Thermo Fisher Scientific TALOS F200S G2 FEG TEM, operating at 200 kV, was used for high-angle annular dark-field scanning transmission electron microscopy (HAADF-STEM) imaging and energy dispersive spectrometry (EDS) mapping.
3. Results and discussion
Figure (a) shows representative flow curves in uniaxial compression for solution-treated LAQ1562 alloy. The yield stress (YS) reaches ∼ 500 MPa, thereby making LAQ1562 among the highest specific-strength lightweight alloys reported considering its ultra-low density (1.34 g/cm3). This leads us to explore the underlying strengthening mechanism of this Ag-bearing BCC Mg-Li–Al alloys.
Figure 1. (a) Mechanical property and (b) XRD profile of the experimental Mg-Li-Al-Ag alloy. The inset in (a) shows the relation between specific strength and density of LAQ1562 with some high strength BCC Mg-Li-Al alloys [Citation9,Citation10,Citation19,Citation21] and conventional lightweight (Mg, Al, Ti) alloys. All the mechanical property results are obtained from macrosize samples.
![Figure 1. (a) Mechanical property and (b) XRD profile of the experimental Mg-Li-Al-Ag alloy. The inset in (a) shows the relation between specific strength and density of LAQ1562 with some high strength BCC Mg-Li-Al alloys [Citation9,Citation10,Citation19,Citation21] and conventional lightweight (Mg, Al, Ti) alloys. All the mechanical property results are obtained from macrosize samples.](/cms/asset/49759670-5f1f-41f9-86a1-7f23a1fa5b48/tmrl_a_2188909_f0001_oc.jpg)
The entire XRD spectrum in the inset of Figure (b) indicates that LAQ1562 alloy in this state consists of a BCC β-Li phase with an additional minor HCP α-Mg phase that can also be observed. The magnified region indicated by the red dashed rectangle in the inset demonstrates that peak separation can be observed (Figure (b)). The two side maxima adjacent to the (110) diffraction peak of the BCC matrix are a typical feature of spinodal decomposition, which involves the amplification of concentration modulations with long wavelengths inside the supersaturated solution that are initiated by random fluctuations. Previous work proved the spinodal decomposition phenomenon in BCC Mg-Li–Al alloys based on morphological, chemical, structural and thermodynamic aspects [Citation10]. It was proposed that the lattice mismatch at the diffuse transition region between the spinodal clusters with the matrix is the key factor for spinodal strengthening. As the atomic radius of Al (143 pm) is smaller than those of Mg (160 pm) and Li (152 pm), the formation of Al-rich clusters would lead to a negative volume strain and thus the side maximum at higher angles in XRD corresponds to the diffraction of the Al clusters and their immediate vicinity. The wavelength (λ) of composition modulations is a crucial parameter for characterizing spinodal microstructures and can be calculated from XRD data using Daniel-Lipson equation [Citation11,Citation12]:
(1)
(1) where h, k and l are the Miller indices of the Bragg peak, θ is the Bragg angle and a is the lattice parameter of the matrix, Δθ is the angular distance between the centers of the side maximum and the Bragg peak of the matrix. Accordingly, the spinodal wavelength is calculated to be ∼ 17.9 nm.
Figure exhibits the typical microstructure of LAQ1562 characterized by TEM. The HAADF-STEM image exhibits bright well-aligned rod-like clusters that are rich in high atomic-number elements (Figure (a)). As Li cannot be detected from EDS, the corresponding EDS mappings of Mg, Ag and Al are shown in Figure (b–d). The results show that the clusters are rich in Ag and Al.
Figure 2. (a) HAADF-STEM image and corresponding EDS mapping of (b) Mg, (c) Ag, (d) Al in the Mg-Li-Al-Ag alloy.
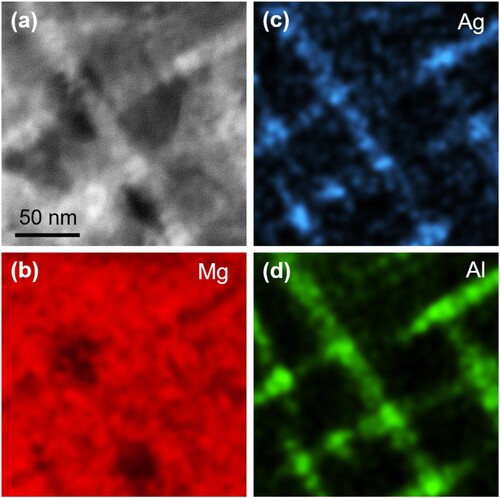
Figure shows a reconstructed APT volume of LAQ1562, indicating the local distributions of elemental Ag, Al, Mg and Li. Modulated solutes with co-clustering of Al and Ag are observed, matching well with the TEM results. The solute clusters are well aligned normal to the elastically soft <100> direction owing to its lowest elastic modulus [Citation9,Citation10]. To obtain a better view of the modulated microstructure, a 2D atomic density contour map is exhibited, which is projected from a 60 nm × 120 nm × 8 nm volume marked by the red dashed rectangle in the reconstructed volume based on the distribution of Al ions. Significant concentration fluctuations with islands of high concentration separated by low-concentration domains can clearly be seen. Moreover, it is intriguing to note that the concentration of Li is higher around the core of Al-Ag co-clusters (black dashed circles in Figure (b1) and Figure (d1)), indicating Li-rich zones that formed in the vicinity of Al-Ag co-clusters.
Figure 3. APT reconstruction of Mg-Li-Al-Ag alloy showing (a) Ag, (b) Al, (c) Mg and (d) Li ions distributions; (a1–d1) 2D atomic density contour maps of Ag, Al, Mg and Li ions projected from a 60 nm × 120 nm × 8 nm volume marked by the red dashed rectangle in (a). The black dashed circles in (b1) represent the core of some Al-Ag co-clusters and the ones in (d1) correspond to the identical positions in (b1).
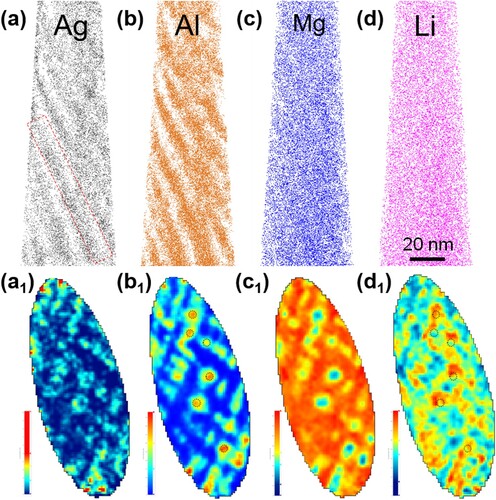
Figure (a) shows the 10 at.% Al iso-composition surface, highlighting the Al-rich clusters. The 1D concentration profile obtained using a 10-nm diameter cylinder volume that extended through the modulated cluster structure (Figure (a)) is exhibited in Figure (b). A near sinusoidal distribution for Ag and Al of average modulation wavelength 10-15 nm can be observed, which matches the calculated results from XRD data. Elemental Al and Ag appear to have almost identical segregation tendencies. The enrichment by elemental Li (marked by pink arrows) around Al-Ag co-clusters was also confirmed.
Figure 4. (a) APT reconstruction exhibiting 10 at.% Al iso-composition surface; (b) 1D concentration profile obtained perpendicular to the modulated clusters in Mg-Li-Al-Ag alloy. The Al-Ag co-clusters are shaded by light yellow. Typical region of elemental Li enrichment around Al-Ag co-clusters are marked by pink arrows; (c) Experimental bulk normalized concentration radial distribution functions (RDFs) of Li with Li.
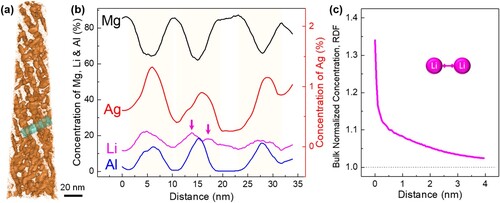
Radial distribution functions (RDF) were calculated to analyse the existence of compositional segregation from the reconstructed APT data. Under this circumstance, each neighborhood solute surrounding a specific elemental species is evaluated and an average composition of each solute type as a function of the radial distance to this particular elemental species is calculated. Compositional segregation occurs when the value of the bulk normalized concentration is larger than unity. The RDF of Li with Li is exhibited in Figure (c) and the Li-Li bulk normalized concentration is mildly larger than unity, proving the aggregation of Li atoms. The RDFs of Al (Figure (a)) and Ag (Figure (b)) with Ag, Al, Mg and Li are exhibited in Figure . Both, the value of the Al-Al and the Ag–Ag bulk normalized concentration are larger than 2 within the distance of 1 nm, indicating a strong compositional segregation of Al and Ag with the same species. Similarly, the Al-Ag/Ag-Al RDFs demonstrate that Ag and Al atoms tend to prefer concurrent segregation, creating regions of high Al-Ag content. Conversely, matrix Mg atoms appear to be repelled by Al and Ag atoms. It is worth noting that the value of Al–Li and Ag-Li bulk normalized concentrations are slightly larger than unity once over a certain distance, which agrees with the preferential decoration of the interface between matrix and Al-Ag co-clusters by Li.
Figure 5. Experimental bulk normalized radial distribution functions (RDFs) of (a) Al and (b) Ag with Ag, Al, Mg and Li, indicating a compositional segregation in Mg-Li-Al-Ag alloy. The regions with the value of the bulk normalized concentration larger than unity is shaded by light green in (a4) and (b4).
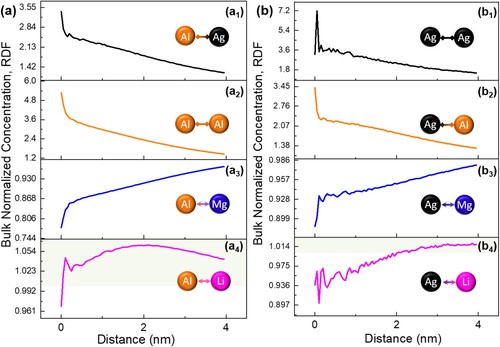
Subtle Ag addition (2 wt.% /0.3 at.%) visibly induced co-clustering of Ag and Al. It is essential to understand the microstructural origin of this phenomenon. XRD results (Figure (b)) showed that the alloy exhibited a similar spinodal scenario as found for BCC Mg-Li–Al alloys [Citation9]. Neither evenly distributed as solid-solution solute nor forming secondary precipitates solely, Ag atoms aggregate to form well-aligned spinodal clusters coupled with Al atoms (Figure and 3). There are two possible pathways for the formation of co-clusters. In the first scenario, spinodal decomposition induces Al clusters [Citation10], followed by the diffusion of Ag atoms towards Al-enriched clusters to generate the observed co-clusters. The diffusion coefficient of Ag is calculated to be at least one order of magnitude lower than that of Al in Mg [Citation13]. Although the diffusivity of Ag and Al in BCC Mg-Li alloys is unknown, it can be deduced that Ag can barely diffuse towards the Al clusters without long-term or high temperature ageing. In the second scenario, Ag clusters are formed via spinodal decomposition. Non-equilibrium quenched-in vacancies for precipitation-hardenable alloys are known to play a vital role in clustering/precipitation at lower temperatures. As the Ag-vacancy binding energy is almost twice higher than the binding energy between an Al atom and a vacancy in Mg [Citation13], Ag-vacancy pairs are bound to form first at low temperature. Al atoms are likely to diffuse to Ag-vacancy pairs to reduce the strain energy, which matches our RDF results indicating the Al-Ag co-segregation tendency. Consequently, spinodal decomposition proceeds heterogeneously on these precursors to form Al-Ag co-clusters. Further investigations are required to use first-principles density functional theory calculations to examine the Gibbs free energy of BCC Mg-Ag solid solutions in order to rigorously analyse the initial stage of co-cluster formation.
The reduction of the free energy (strain energy and/or interfacial energy) constitutes the driving force for the redistribution of Li atoms. Generally speaking, interfacial segregation can decrease the strain energy and/or the interfacial energy. However, diffuse Li-rich zones around the clusters can be observed rather than conventional thin-layered segregation at well-defined precipitate/matrix interfaces. Although diffuse interfaces between spinodal clusters and matrix can be observed in Figure (b), the sizes of the Li-enriched zones (Figure (d1)) are almost identical or even larger than that of the co-clusters, which is beyond the scale of interfaces. The strain excess energy of interfaces is known to be proportional to the interfacial lattice misfit [Citation14]. Different from interfacial segregation, it is proposed in this paper that the reduction of interfacial lattice misfit is achieved by a compositional redistribution in the matrix of the present alloy. As the atomic radius of Li is smaller than that of Mg and the diffusivity of Li is fast, even at ambient temperature [Citation9], the aggregation of Li atoms around Al-Ag co-clusters can easily occur to reduce the lattice parameter of the matrix. Hence, the interfacial lattice misfit is decreased to lower the excess energy of the system.
High strength generated in LAQ1562 is bound to be the result of spinodal-induced Al-Ag co-clustering and Li redistribution. Two main factors determine the magnitude of incremental yield stress in spinodally modulated BCC alloys: first, lattice misfit effect by the coherent internal stress on account of decomposition; second, modulus effect owing to spatial variation of elastic modulus [Citation15,Citation16]. As the atomic radius of Al and Ag (144 pm) is smaller than that of Mg and Li with evident sidebands observed in XRD profile (Figure (b)), lattice misfit effect caused by Al-Ag co-clustering are critical for strengthening. Modulus improvement by aggregation of Al and Ag are also expected, and thus modulus effect will contribute to yield stress increment undoubtedly. Moreover, Li redistribution in the matrix will induce coherency strain field. Dislocations tend to lie in low-energy positions with respect to the strain fields and the atomic displacements associated with dislocations interacting with the coherency strain fields will provide certain strengthening effect [Citation17,Citation18]. In a word, through proper chemical engineering, combinatorial spinodal strengthening and coherency strain hardening give rise to high strength achieved in LAQ1562 alloy.
Quantitative estimations of different strengthening mechanisms are vital to evaluate the contributions of them. The yield strength of LAQ1562 is ∼500 MPa, leading to ∼400 MPa increment compared to binary Mg-Li counterparts [Citation19]. For spinodal structure in Mg-Li–Al-Ag alloys, dislocations will be trapped by (Al, Ag)-rich clusters with diffuse interfaces. The evaluation of lattice misfit strengthening can be expressed as [Citation17,Citation20] Δσ = M·ks·(AηY)5/3 ·(λ/Gb)2/3, where M = 3 is the Taylor factor, ks = 0.122·[π(1 − υ)/(1 − 2υ)]2/3 is a material constant, υ is the Poisson ratio, A is the amplitude of modulation (in at.%), λ is the wavelength of the composition modulation, and η = a−1·da/dC is the lattice strains caused by spinodal decomposition, in which a is the lattice constant for the matrix, da/dC is the variation in the lattice constant with the composition C. As our alloy only contains 0.3 at.% Ag and the concentration of Li and Al are close to those of LA147 alloy, elastic modulus of LA147 are used here for calculation [Citation10]. E = 52.91 GPa is the elastic modulus, Y = E/(1 − υ) = 66.14 GPa is an elastic dependent parameter, G = 22.06 GPa is the shear modulus, and b = √3·a/2 is the Burgers vector. λ is evaluated to be ∼10 nm from APT results (Figure (b)), whereas this value calculated by Daniel-Lipson equation approximates to 17.9 nm based on XRD result (Figure (b)). As the value evaluated based on APT data is pretty local and that calculated from XRD results is more reliable, we take 15 nm as the λ value. Accordingly, Δσ by lattice misfit is calculated to be ∼320 MPa. Previous study indicated that a 22% increase of Al content only results in a 3.6% increment in shear modulus, which substantially weakens modulus hardening effect [Citation10]. Indeed, the combined effects of modulus and coherency strain can be predicted to be Δσ ∼80 MPa considering the total yield strength increment being ∼400 MPa. It is reasonable to conclude that lattice misfit effect contributes the most to strengthening effect. First-principles calculation of elastic constants/modulus and more detailed quantitative analysis of individual strengthening mechanism in Ag-containing Mg-Li–Al alloys will be further investigated.
4. Conclusion
In summary, co-clustering and solute redistribution is observed in a high-strength BCC Mg-Li–Al-Ag alloy. The phenomenon of spinodal decomposition is obvious, which indicates the occurrence of solute clustering. Owing to the diffusion of Al atoms to Ag-vacancy pairs, co-clustering of Al and Ag occurs. The compositional redistribution of Li in the matrix close to the interface between matrix and co-clusters reduces the interfacial lattice misfit and hence lowers the strain energy. Spinodal strengthening and coherency strain hardening give rise to the high strength in LAQ1562 alloy, with lattice misfit effect contributing the most to strengthening. Dual alloying of Al and Ag in BCC Mg-Li alloys paves the road for designing ultralightweight alloys with unique microstructures and improved mechanical performance.
Acknowledgements
T.L. acknowledges the financial support from the Alexander von Humboldt Foundation and the fruitful discussion with Dr. Baptiste Gualt.
Disclosure statement
No potential conflict of interest was reported by the author(s).
Additional information
Funding
References
- Polmear I, StJohn D, Nie J-F, et al. Light alloys: metallurgy of the light metals, Butterworth-Heinemann. 2017.
- Pollock TM. Weight loss with magnesium alloys. Science. 2010;328(5981):986–987.
- Easton M, Beer A, Barnett M, et al. Magnesium alloy applications in automotive structures. JOM. 2008;60(11):57–62.
- Li C, Xu D, Wang B, et al. Natural ageing responses of duplex structured Mg-Li based alloys. Sci Rep. 2017;7:40078.
- Guo F, Jiang L, Ma Y, et al. Strengthening a dual-phase Mg-Li alloy by strain-induced phase transformation at room temperature. Scripta Mater. 2020;179:16–19.
- Tang S, Xin T, Xu W, et al. The composition-dependent oxidation film formation in Mg-Li-Al alloys. Corros Sci. 2021;187:109508.
- Wu R, Yan Y, Wang G, et al. Recent progress in magnesium-lithium alloys. Int Mater Rev. 2015;60(2):65–100.
- Jackson J, Frost P, Loonam AC, et al. Magnesium-lithium base alloys—preparation, fabrication, and general characteristics. JOM. 1949;1(2):149–168.
- Tang S, Xin T, Xu W, et al. Precipitation strengthening in an ultralight magnesium alloy. Nat Commun. 2019;10(1):1003.
- Xin T, Zhao Y, Mahjoub R, et al. Ultrahigh specific strength in a magnesium alloy strengthened by spinodal decomposition. Sci Adv. 2021;7(23):eabf3039.
- Ditchek B, Schwartz LH. Diffraction study of spinodal decomposition in Cu-10 w/o Ni-6 w/o SN. Acta Metall. 1980;28(6):807–822.
- Wang LD, Chen CL, Kang MK, et al. On the daniel-lipson’s wavelength formula of spinodal decomposition. Scripta Mater. 2000;42(7):725–730.
- Zhou B-C, Shang S-L, Wang Y, et al. Diffusion coefficients of alloying elements in dilute Mg alloys: A comprehensive first-principles study. Acta Mater. 2016;103:573–586.
- Wen H, Zhao B, Dong X, et al. Preferential growth of coherent precipitates at grain boundary. Mater Lett. 2020;261:126984.
- Kato M. Hardening by spinodally modulated structure in b.c.c. alloys. Acta Metall. 1981;29(1):79–87.
- Kato M, Mori T, Schwartz LH. Hardening by spinodal modulated structure. Acta Metall. 1980;28(3):285–290.
- Ardell A. Precipitation hardening. Metall Trans A. 1985;16(12):2131–2165.
- Gladman T. Precipitation hardening in metals. Mater Sci technol. 1999;15(1):30–36.
- Park GH, Kim JT, Park HJ, et al. Development of lightweight Mg Li Al alloys with high specific strength. J Alloys Compd. 2016;680:116–120.
- Liang Y-J, Wang L, Wen Y, et al. High-content ductile coherent nanoprecipitates achieve ultrastrong high-entropy alloys. Nat Commun. 2018;9(1.
- Hagihara K, Mori K, Nakano T. Enhancement of plastic anisotropy and drastic increase in yield stress of Mg-Li single crystals by Al-addition followed by quenching. Scripta Mater. 2019;172:93–97.