ABSTRACT
The cytoskeleton is crucially important for the assembly of cell-cell junctions and the homeostatic regulation of their functions. Junctional proteins act, in turn, as anchors for cytoskeletal filaments, and as regulators of cytoskeletal dynamics and signalling proteins. The cross-talk between junctions and the cytoskeleton is critical for the morphogenesis and physiology of epithelial and other tissues, but is not completely understood. Microtubules are implicated in the delivery of junctional proteins to cell-cell contact sites, in the differentiation and spatial organization of the cytoplasm, and in the stabilization of the barrier and adhesive functions of junctions. Here we focus on the relationships between microtubules and junctions of vertebrate epithelial cells. We highlight recent discoveries on the molecular underpinnings of microtubule-junction interactions, and report new data about the interaction of cingulin and paracingulin with microtubules. We also propose a possible new role of junctions as “molecular sinks” for microtubule-associated signalling proteins.
Introduction
One of the central questions of cell biology is to understand the molecular mechanisms through which cells and tissues achieve their bewildering variety of shapes and functions, and how this relationship is dynamically regulated. How do cells generate, maintain, and alter their shapes? How does form translate into function? How do, in turn, cellular components regulate the shape-generating forces? Decades of research have established that the cytoskeleton, i.e. actin filaments, microtubules (MTs) and intermediate filaments, plays a fundamental role in determining cell shape, and provides cells with resistance to mechanical stresses. This dynamic network of filamentous proteins has many additional functions. It supports whole cell and tissue movement, cell division, precise segregation of genetic material into daughter cells, cytoplasm organization, intracellular vesicular traffic, regulation of gene transcription, and much more. In vertebrates, tissue and organ physiology also require a precise architectural organization and regulation of cell-cell interactions. In epithelial and endothelial tissues, such interactions are largely dependent on highly specialized cell-cell junctions, such as tight junctions (TJ), adherens junctions (AJ), and desmosomes. Importantly, the formation, dynamics and functions of cell-cell junctions crucially depend on their interaction with cytoskeletal filaments, providing an example of cytoskeleton-dependent structure-function relationship. In this review, we discuss the role of a subset of cytoskeletal polymers, e.g. MTs, in the regulation of epithelial junctions. We first briefly describe the composition, organization and distribution of MTs in vertebrate epithelial cells, and introduce a few basic concepts about epithelial junctions. In the second part, we discuss the roles of MTs in the regulation of junctions, and, on the other hand, the roles of specific junctional proteins in regulating MT organization. We also report new data about the interaction of the junctional proteins cingulin and paracingulin with MTs. Finally, we propose the new concept that junctions can function as “molecular sinks” for MT-associated signalling proteins, by focusing on a number of examples of such proteins, that bind to MTs and localize at junctions through known and unknown mechanisms.
Microtubules
Among different types of cytoskeletal polymers, microtubules (MTs), along with actin filaments, are the most evolutionarily conserved, since they are present in all eukaryotes, where they promote the generation of mechanical force and movement through kinesin and dynein (for MTs), and myosin (for actin filaments) motors, respectively. Although proteins similar to tubulin and actin are also found in prokaryotes, the associated protein motors appear to be missing.Citation1 MTs are hollow cylindrical polymers of heterodimeric subunits made of α- and β-tubulin, and are typically made up of 13 parallel protofilaments.Citation2 They are polarized, with plus ends, which are highly dynamic, undergoing either rapid polymerization or rapid depolymerization (catastrophe), and minus ends, which are typically either stabilized or acting as sites of depolymerization.Citation3 Polymerizing MTs are nucleated and stabilized at their minus ends by the γ-tubulin ring complex (γ-TuRC). The γ-TuRC is the main structural unit of microtubule organizing centers (MTOCs), which are found both at centrosomes, and at non-centrosomal sites, such as the Golgi apparatus.Citation4 Tubulins are targets for numerous types of post-translational modifications (PTMs) affecting their C-terminal sequences, including de-tyrosination, Δ2-tubulin generation, polyglutamylation, polyglycylation, and acetylation.Citation5 The functional significance and mechanisms of tubulin PTMs have been investigated in neuronal cells, where PTMs regulate MTs organization and interactions with motors, but their role in epithelial cells is less clear.
MTs have many functions. Besides their role in the formation of the mitotic spindle and chromosome segregation in mitosis, they serve as tracks that facilitate targeted vesicular transport, they interact with different membrane-bound organelles to compartmentalize the cytoplasm, and they underlie the formation of apical cilia in epithelial cells (). The organization of the MT cytoskeleton is cell-type dependent, and is related to the shape, cytoplasmic organization, differentiation and function of each cell. In unpolarized, non-differentiated epithelial cells, that do not have mature junctions, most MTs are nucleated by the centrosomes, the Golgi apparatus and other non-centrosomal sites, and their plus ends are directed towards the cell periphery ()). In contrast, number of mechanisms account for the asymmetric organization of non-centrosomal MTs in differentiated cells.Citation6 In polarized, differentiated epithelial cells with stable junctions, the majority of MTs lose their connection with perinuclear MTOCs, and non-centrosomal MTs align along the apico-basal axis, with the minus ends oriented towards the apical region, and the plus ends oriented towards the basal region, where they form a scattered networkCitation7 ()). Thus, as epithelial cells become polarized and form junctions, the MT nucleating activity becomes more spatially disperse, and MTs become more stable.Citation8 Importantly, the plus-end dynamic instability of MTs is suppressed in cells that form junctions, and individual MTs exhibit an extended state of pause, suggesting that they become capped.Citation9 In stratified epithelia, such as the skin epidermis, keratinocytes drastically reorganize their MT cytoskeleton during differentiation, and radial MTs emanated from the centrosomes become non-centrosomal, and align along the cell cortex.Citation6 In some cell types, such as cultured mouse mammary epithelial cells (Eph4), structured illumination microscopy reveals a new organization of apical non-centrosomal MTs, in the form of planar apical networks (PANs).Citation10
Figure 1. The organization and functional interactions of MTs in polarizing and polarized epithelial cells. (a) Schematic cartoon of two polarizing epithelial cells, with MTs anchored at their minus-ends at centrosomes and at non-centrosomal sites, and kinesin-mediated transport of junctional molecules and membrane vesicles. (b) Schematic cartoon of polarized differentiated cells, each displaying an apical cilium, and MTs oriented with their minus-ends apically, and plus-ends basally. Cell-cell junctions are indicated on the right, and selected junctional molecules are also schematically drawn (out of scale). (c) Graphical legend for structures and proteins implicated in MTs-junctions functional interactions, shown in A and B.
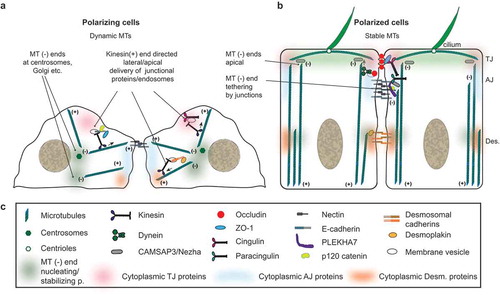
The plus-ends of microtubules are highly dynamic, switching between polymerization and depolymerization cycles, and interact with different plus-end tracking proteins (+TIPs): EB family proteins (e.g. EB1), CAP-Gly proteins (e.g. CLIP170), proteins containing basic and Serine-rich sequences (e.g. APC), HEAT and WD40-repeat proteins (e.g. LIS1), and MT motor proteins (e.g. dynein).Citation4 + TIPs function to link MTs plus-ends to cellular structures, to regulate MT dynamics, to recruit signaling factors, and to generate pushing and pulling forces, via interaction with motors. The minus-ends of MTs are either stabilized by binding to capping proteins, or undergo either depolymerization, or, more rarely, slow polymerization.Citation4 The balance between stabilization and minus-end depolymerization is important to control MT organization both in interphase cells and during mitosis, where minus-end depolymerization at the spindle poles allows poleward MT flux. Two classes of proteins bind to the minus-ends of MTs. The γ-tubulin ring complex (γ-TURC), mentioned above, is present at centrosomes and at Golgi MTOCs.Citation4 However, in differentiated cells most minus-ends are either free in the cytoplasm or tethered to different cytoplasmic structures. In this case, the minus-ends bind to the second class of proteins, belonging to the CAMSAP/Patronin/nezha family, which are exclusive of animal cells.Citation4 While CAMSAP1 exclusively tracks the growing MT minus-ends, CAMSAP2 and CAMSAP3/nezha are not only recruited by the growing minus-ends, but can also stay bound laterally to MTs, as they elongate. CAMSAP2 and Nezha/CAMSAP3 suppress the MT-organizing activity of the centrosome, through unknown mechanisms, so that the γ-TURC is no longer required for MT nucleation.Citation11 Depletion of CAMSAP3/nezha in cultured cells promotes increased MT nucleation from centrosomes, and causes a reduction in MTs with polymerizing plus-ends, as well as inhibition of cell migration.Citation11,Citation12 The disruption of MT organization resulting from depletion of minus-end capping proteins has consequences on cellular morphogenesis. For example, in cultured cells depletion of CAMSAP3/nezha affects junction assembly, Golgi and nucleus positioning and polarization, and lumen formation in 3D.Citation13 In contrast, upon KO of CAMSAP3/nezha in mice, the organization of the MT network and the positioning of the nuclei and Golgi are disrupted in intestinal cells, and some apical markers are mis-localized, but brush border morphology and epithelial junctions are not affected, suggesting redundant mechanism to promote apico-basal polarization.Citation13
Cell-cell junctions
In vertebrate epithelial cells, the junctional complex comprises the tight junction (TJ, also known as zonula occludens or ZO), the adherens junction (AJ), and desmosomes ()).Citation14 TJ seal the apico-lateral borders of polarized cells, to prevent the free diffusion of solutes across the paracellular space (barrier function), and to define the border between the apical and lateral domains of the plasma membrane, which have a different composition (fence function). AJs are primarily involved in cell-cell adhesion and sensing of mechanical forces, and comprise two spatially distinct domains. The apical region, called zonula adhaerens (ZA), is a circumferential continuous junction, which is found immediately basal to the TJ. Together, the TJ and the ZA constitute the “zonular” apical junction (also denoted as “apical junctional complex”-AJC), which forms a continuous belt around the apico-lateral regions of polarized epithelial cells, and is connected to a subcortical bundle of contractile actin filaments. The basal part of epithelial AJ, referred to as “lateral contacts”, is constituted by a looser arrangement of cell-cell adhesive structures, which are uniformly distributed along the lateral surfaces, and are associated with a less contractile cortical actomyosin cytoskeleton.Citation15 Thus, clustering of adhesion receptors distinguishes ZA from lateral contacts, and lateral contacts may be viewed as a “reservoir” of junctional and signaling molecules that can eventually be clustered at zonular junctions during differentiation. Desmosomes are hyper-adhesive button-like structures distributed on the lateral surfaces of epithelial cells, and they provide tissues with a strong resistance to mechanical stress.Citation16 In endothelial cells, since the height of the lateral region is very small, TJ and AJ are intermingled, instead of being spatially separated, as they are in epithelial cells.Citation17 Furthermore, unlike TJ and desmosomes, which are typical of epithelial cells, cadherin-based AJ can be found in most cell types, including fibroblasts, muscle cells and neurons.
From a molecular standpoint, TJ, AJ and desmosomes are organized in a similar fashion ()). Transmembrane molecules, many of which act as cell-cell adhesion molecules, interact in cis to cluster at junctions, and in trans to confer adhesive (TJ, AJ, desmosomes) and barrier (TJ) properties to junctions. These molecules comprise Ig-like adhesion molecules such as JAM-A and CAR at TJ, cadherins and nectins at AJ, and desmogleins and desmocollins (which belong to the cadherin superfamily) at desmosomes. In addition, the 4-pass transmembrane molecules claudins, occludin and tricellulin are critical to set up and regulate the paracellular barrier at the TJ. On the cytoplasmic side, the intracellular domains of the transmembrane junctional proteins interact with complexes of cytoplasmic scaffolding and adaptor proteins. The cytoplasmic proteins (indicated by colour-coded “clouds” in ) have multiple functions. They cluster transmembrane proteins at the junctional sites, thus making it possible, for example, to generate intramembrane continuous fibrils of claudins.Citation18 They can also regulate the turnover and membrane association of transmembrane proteins. They can either directly or indirectly connect the transmembrane proteins to the actin, MT and intermediate filament cytoskeletons, thus stabilizing the respective junction. They can bind to transcription factors, RNA-associated molecules, kinases, GEFs, GAPs and other signaling molecules, thus either sequestering and inactivating them, or directing the site of their function at junctions.Citation19 Among the most prominent cytoplasmic scaffolding/adaptor proteins are ZO proteins (ZO-1, ZO-2 and ZO-3) and cingulin-family proteins (cingulin and paracingulin) at TJ, catenins (p120-catenin, α-catenin, β-catenin), afadin and PLEKHA7 at AJ, and desmoplakin and plakoglobin at desmosomes. In addition, two protein complexes which are involved in signaling to direct the establishment of apico-basal polarity, the Par (Par3-Par6-apKC) and Crumbs (Crumbs-Pals1-PATJ) complexes, are associated apically with the cytoplasmic region of TJ, whereas the Lgl/Scribble/Dlg complex identifies the lateral membrane.Citation20 The actin and intermediate filament cytoskeletons are crucial to allow tissues to adapt to physiological mechanical stresses, and specific junctional adaptor proteins, such as α-catenin, vinculin and ZO-1, have been shown to respond to force with changes in their conformation and interactions,Citation21,Citation22 to transduce mechanical signals. The reader is referred to additional excellent reviews for a more detailed description of the molecular organization of TJ, AJ and desmosomes, and the functional significance of the interaction of these junctions with the cytoskeleton.Citation17,Citation23-Citation31
Regulation of junction assembly and homeostasis by microtubules
Tight junctions
The importance of MTs for TJ physiology and organization was first revealed by studies using colchicine, a drug that disrupts MTs organization. Treatment of MDCK cells with colchicine caused transient changes in trans-epithelial electrical resistance (TER), a measure of TJ barrier function, and in the structure of TJ, as determined by electron microscopy.Citation32 Colchicine also affected TJ ultrastructure and permeability to lanthanum in hepatocytes in vivo.Citation33 These early findings were confirmed by subsequent studies on cultured thyroid epithelial cells, showing that the barrier function of TJ was decreased, and the junctional accumulation of ZO-1 was disrupted, following colchicine treatment.Citation34 Furthermore, treatment of MDCK cells with nocodazole, a drug which interferes with the polymerization of MTs, perturbs the junctional localization of the TJ/AJ protein paracingulin.Citation35 Also, in human intestinal cells TJ barrier formation requires MTs integrity and the minus-end motor protein dynein,Citation36 indicating that plus-end directed transport is involved in delivering TJ proteins to the membrane. Another evidence that MTs are required for TJ formation is the observation that either nocodazole treatment or depletion of Dual leucine zipper-bearing kinase (DLK), which is required for MT reorganization, significantly decreases the levels of ZO-1 and claudins at the cell periphery in keratinocytes.Citation37,Citation38 Conversely, the MT-stabilizing drug paclitaxel promotes keratinocyte differentiation, cortical accumulation of junctional proteins, and barrier formation.Citation38
MTs likely contribute to TJ assembly by functioning as tracks for the delivery of TJ proteins through MT-associated vesicles. During the first division of the Xenopus oocyte, conspicuous ZO-1-stained vesicles are aligned in the cytoplasm, likely along MT tracks, in a plane orthogonal to the plane of cleavage, and appear to fuse with the basolateral membrane.Citation39 In contrast, in dividing Xenopus oocytes occludin-containing granules are found near the tip of oolemma ingression,Citation39 suggesting that at least two types of MT-associated membrane vesicles deliver TJ proteins to the sites of cell-cell contact. In agreement, recent studies confirm that occludin moves along MT tracks.Citation36 The notion that MTs are also involved in epithelial morphogenesis is supported by studies showing that during epithelial lumen formation, which is associated with the formation of new junctions between the apicolateral regions of epithelial cells, the anterograde MT motor kinesin-2 mediates the plus-end directed transport of apical endosomes.Citation40 More recent studies show that the fusion of the apically targeted endosomes with the plasma membrane requires Rab11 and its effector FIP5, as well as the TJ protein cingulin, which interacts with FIP5 and with MTs.Citation41
In addition to the organization and maintenance of junctional integrity, MTs are involved in disassembly of TJ. This role of MTs was first characterized using the calcium depletion model of junction disassembly in intestinal and renal epithelial cells, which affects both TJ and AJ.Citation42 In the absence of nocodazole, calcium depletion leads to disruption of junctions, formation of intracellular contractile actin rigs, and internalization of the TJ proteins occludin and ZO-1, and the AJ proteins E-cadherin and β-catenin, which associate with the actin rings. Depolymerization of MTs with nocodazole prevents the translocation of junctional proteins into these cytosolic ring-like structures.Citation42 Conversely, drug-mediated stabilization of MTs attenuates the disassembly of the apical junctions, and blocks the contraction of the actin rings.Citation42 MT-associated motor activity is implicated in ring formation, since the plus-end directed motor kinesin-1 is localized at junctions, where it associates with E-cadherin and catenins, and treatment with the kinesin inhibitor AMP-PNP blocks the contraction of F-actin rings and the translocation of E-cadherin and occludin into the subapical cytosolic rings.Citation42 Actomyosin contractility is crucial for junction disassembly following calcium depletion.Citation43-Citation46 Indeed, the RhoA-ROCK pathway, mediated by the MT-associated GEF GEF-H1, was shown to be implicated in the formation and contractility of actin rings following calcium depletion, suggesting that MTs control junction disassembly by regulating factors that affect actomyosin contractility.Citation47 GEF-H1 is also implicated in the restoration of functional junctions in adhesion-defective cancer cells, through which inhibition of MT polymerization activates GEF-H1, inducing actomyosin contractility, and a mechanosensitive response which stabilizes junctions.Citation48 Another evidence that MT are implicated in TJ and AJ assembly and stability was the observation that overexpression of the β-tubulin chaperone cofactor D, which acts as a GTPase activating protein for β-tubulin, induces disassembly and prevents assembly of TJ and AJ in MDCK cells.Citation49 In summary, these results indicate that MT dynamics is essential to regulate both assembly and calcium-depletion-mediated disassembly of apical junctions, and that MT operate, at least in part, through mechanisms that affect RhoA and downstream regulation of actomyosin contractility.
Adherens junctions
The first study addressing the role of MTs in the assembly of the AJC reported that colchicine treatment of thyroid epithelial cell monolayers reduced the junctional accumulation of E-cadherin.Citation34 Furthermore, in lung epithelial cells inhibition of MT plus-end growth induced breakage of AJ, loss of F-actin from AJ, and rearrangement of catenins into puncta, and eventually their disappearance from junctions.Citation9 It was proposed that junction breakage was not due to the acute formation of cytoplasmic stress fibers, that is observed following treatment with nocodazole, but rather to the decrease in junctional actin filaments occurring after MT depolymerization.Citation9 These observations reinforce the notion that MT organization and dynamics has multiple effects on actomyosin organization.
Several AJ proteins are delivered to the cell surface through the dynamic plus-ends of MTs and associated motors ()). In fibroblasts, kinesin is required for the formation of N-cadherin-dependent cell-cell contacts,Citation50 and p120-catenin association with kinesin promotes the transport of the catenin-cadherin complex to junctions.Citation51 In agreement, the KO of KAP3, a subunit of kinesin-2, results in decreased levels of N-cadherin and β-catenin in embryonic mouse neural precursors,Citation52 whereas the overexpression of another kinesin, KIF17, promotes the apical and junctional accumulation of actin and E-cadherin, respectively.Citation53 In epithelial Pkt2 cells cytoplasmic dynein transiently tethers MTs at sites of cell-cell contact during junction biogenesis, providing a track for the kinesin-dependent delivery of junctional components.Citation54 Another plus-end binding protein, CLIP170, targets MTs to AJs prior to apico-basal array assembly.Citation55 Either expression of mutant CLIP170, which affects MT dynamics, or depolymerization of dynamic MTs by nocodazole, reduces the accumulation of E-cadherin at junctions, as well as the accumulation of myosin-IIA and phosphorylated myosin light chain.Citation56 Since surface expression of E-cadherin was not affected by nocodazole, it was suggested that dynamic MTs stabilize and regulate the junctional clustering of E-cadherin, rather than its surface expression, by modulating the actomyosin cytoskeleton.Citation56 Cortical MT-associated transfer of junction-associated proteins can also orchestrate the localization of cell fate determinants. For example, β-catenin is transported towards the plus-ends of MTs, and acts as a dorsal determinant in the Xenopus egg.Citation57
In polarized epithelial cells, multiple mechanisms account for the apicolateral anchoring of MTs minus-ends and the formation of non-centrosomal MTOCs ()). First, different centrosomal proteins, including ninein and CAP350, move along MT tracks to reach AJ, where they act as non-centrosomal MT anchoring sites, at least in some cell types.Citation58-Citation60 In MDCK cells CAP350 is recruited to AJ by α-catenin, and its depletion induces an enlargement of the apical domain and a reduction in cell height, and prevents the formation of cell-cell contacts in the calcium switch model of junction assembly.59 Second, MT minus-ends are anchored to AJ by the interaction of CAMSAP3/nezha with PLEKHA7.Citation61 This anchoring has a stabilizing effect on AJ, since depletion of either PLEKHA7 or CAMSAP3/nezha decreases the accumulation of E-cadherin at the ZA.Citation61 The minus-end directed kinesin KIFC3 is targeted to the ZA in a MT-, PLEKHA7- and CAMSAP3-dependent manner, but it does not colocalize with E-cadherin,Citation61 suggesting that it is not involved in E-cadherin transport to the junction. On the other hand, KIFC3 transports to the junction the ubiquitin-specific protease 47 (USP47), which deubiquitinates E-cadherin, and thus prevents its degradation.Citation62 Thus, cadherin stabilization by PLEKHA7/nezha involves its decreased ubiquitination and degradation. As indicated above, the anchoring of MTs minus-ends to AJ and then to the apical membrane plays a role in epithelial morphogenesis, since depletion of CAMSAP3 leads to cell flattening, a wider apical cell surface, although normal cell height is restored after full polarization.Citation13
In summary, these studies show that dynamic MTs are required for the efficient assembly of AJ and delivery of factors that regulate junction dynamics, and multiple mechanisms contribute to tethering MTs to AJ during epithelial differentiation and polarization.
Desmosomes
The first evidence for MT-desmosome interaction was the co-localization between desmoglein-1 and MTs in cultured cells.Citation63 The importance of MT organization for desmosome assembly was further demonstrated by the observation that desmoglein-2 and desmocollin-2 depend on MTs for their rapid accumulation at junctions, requiring kinesin-1 and kinesin-2, respectively, for their transport to the surface.Citation64 Desmosome function requires MTs, because depletion of these kinesin motors dramatically weakens cell-cell adhesive strength in confluent epithelial sheets, despite the observation that desmosomal plaque proteins are not affected.Citation64 In addition, the genetic loss of the centrosomal protein LIS1, which re-localizes to desmosomes upon differentiation to reorganize non-centrosomal MTs, leads not only to MT organization defects, but also to severe loss of desmosome stability and epidermal barrier activity, and decreased expression of desmosomal proteins, resulting in embryonic lethality.Citation65 In a tissue-engineered skin model, nocodazole treatment reduces the number and size of desmosomes, and the accumulation of desmoglein-1 at the cell cortex.Citation37 The mitogen-activated protein kinase DLK (dual leucine zipper-bearing kinase) is expressed in granular layer of the epidermis and is required for terminal differentiation of keratinocytes.Citation66 This kinase acts through MTs, since in the epidermis of DLK KO mice, which die soon after birth, MTs fail to align along the cell cortex, and decreased localization of desmoplakin and LIS1 at junctions leads to MT disorganization and to short desmosomes with increased intercellular spaces, similar to nocodazole-treated tissues.Citation37 In summary, in keratinocytes MTs allow trafficking of desmosome transmembrane proteins (desmogleins and desmocollins) to the sites of junction formation, and are critical for keratinocyte differentiation. Finally, MTs are not only involved in desmosome assembly, but also in their disassembly. Desmosomes are internalized following removal of extracellular calcium in MDCK cells, and their intracellular transport is regulated by MTs, leading to lysosomal degradation of internalized desmosomal proteins.Citation67
Regulation of MTs organization by junctional proteins
While MTs are important in the regulation of junction assembly and disassembly and in epithelial differentiation, the cross-talk between MTs and junctions also involves the interaction of several junctional proteins with either MTs or MT-associated proteins, resulting, for example, in the tethering of MTs to TJ, AJ and desmosomes.
Cingulin (CGN), a specific TJ protein, was initially discovered as a protein associated with the actomyosin fraction from intestinal cells lysates,Citation68,Citation69 and was later found to organize the planar apical network (PAN) of MTs in cultured mammary epithelial (Eph4) cells.Citation10 Cingulin controls tethering of the PAN to TJ, based on the observation that depletion of cingulin leads to disruption of the association of organized MT with TJ.Citation10 PANs have not been detected in all epithelial cell types, thus it remains to be seen how cingulin promotes MT organization in other cell types. Phosphorylation of the CGN head domain on Ser 132 and Ser 150 by AMPK is required for cingulin anchoring of MTs and for cyst formation in 3D cultures, implying that the cingulin-MT association is important for epithelial morphogenesis.Citation10 In agreement with this idea, cingulin was found to be involved in the proper establishment of the Apical Membrane Initiation Site (AMIS) and subsequent formation of apical lumen in epithelial cysts.Citation41 The interaction with MTs is relevant, because cingulin mutants that bind less well to tubulin were localized ectopically and failed to rescue the multi-lumen phenotype of cingulin-KO cysts.Citation41 Proteolytic digestion of the C-terminus of tubulin and mutation of the cingulin head domain reduces the MT-cingulin interaction, indicating that a basic patch within the cingulin head binds to an acidic patch within the unstructured C-terminal tail of tubulin.Citation41 Cingulin participates in lumen formation by interacting with FIP5 (Rab11 family interacting protein 5), which is an effector of the Rab11 GTPase, and is required for apical endosome transport and targeting.Citation41 AMPK kinase, which phosphorylates cingulin,Citation10 is in turn activated by phosphorylation by the LKB1 kinase.Citation70 Interestingly, in LKB1-KO mice the level of phosphorylated AMPK is highly reduced, and CGN junctional accumulation in hepatocytes is lost, correlating with changes in cell morphology, and TJ integrity and permeability.Citation71 This suggests that the LKB1-AMPK kinase axis controls the apical accumulation of cingulin through its phosphorylation.
The evidence that cingulin binds to MTs is three-fold. First, a gel overlay assay using tubulin as a ligand binds to cingulin among different proteins present in lysate of a junction-enriched liver membrane fraction.10 Second, the recombinant cingulin head domain co-immunoprecipitates with α-tubulin.Citation10 Third, the same domain co-pellets with microtubules.Citation41 To confirm and extend these studies, we examined the interaction of full-length recombinant cingulin and paracingulin (also known as JACOP or cingulin-like-1, CGNL1), expressed in baculovirus-infected insect cells, with MTs. We found that not only full-length cingulin, but also paracingulin co-pellet specifically with MTs (), thus providing further evidence for cingulin MT-interaction, and reporting for the first time an interaction of paracingulin with MTs.
Figure 2. Full-length cingulin and paracingulin co-sediment with MTs in vitro. Top: Immunoblot analysis, using antibodies against cingulin (CGN, left) and paracingulin (CGNL1, right) of supernatant (S) and pellet (P) fractions following centrifugation (100,000 x g) of cingulin and paracingulin (His-tagged, purified from baculovirus-transduced insect cellsCitation72), either in the absence (-) or in the presence (+) of taxol-stabilized brain MTs (cat n. BK029, Cytoskeleton). Bottom: Coomassie blue staining of corresponding gels, showing sedimented tubulin in pellet fractions. Numbers on the left indicate approximate Mr, based on the migration of pre-stained markers. Densitometric analysis of immunoblots from three experiments show that the presence of MTs promotes the sedimentation of a statistically significant fraction of both cingulin and paracingulin.
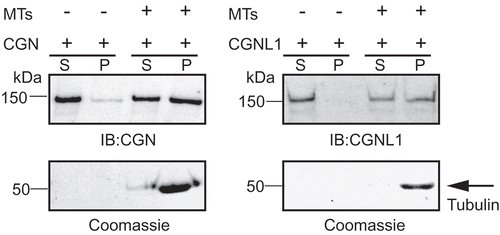
The interaction of paracingulin with MTs suggests that it may also function to tether MTs to junctions. However, no experimental evidence is available so far in this sense, except for the observation that disruption of MTs alters CGNL1 localization.Citation35 Unlike cingulin, which has been detected only at apical TJ, paracingulin has been detected both at TJ and AJ, depending on cell type,Citation73 and both ZO-1 and PLEKHA7 contribute to its junctional recruitment.Citation74 On the other hand, ZO-1 is solely responsible for cingulin recruitment to TJ.Citation75,Citation76 Thus, both cingulin and paracingulin may anchor MTs to TJ and AJ, respectively. Interestingly, paracingulin (e.g. hypothetical protein FLJ14957) was identified among human centrosomal proteins detected through mass-spectrometry-based proteomic analysis.Citation77 Furthermore, antibodies against cingulin and paracingulin label centrosomes in fixed cells.Citation35 Although this labeling could be due to cross-reaction of the antibodies with coiled-coil proteins, we also observed that GFP-tagged forms of cingulin and paracingulin dynamically localize both at centrosomes and junctions in live cultured epithelial cells.Citation35 This evidence, and proteomic data, indicate that cingulin and paracingulin are bona fide centrosomal components. The potential role of cingulin and paracingulin at centrosomes, and their interactors at the centrosome, are not known. However, the centrosomal localization of cingulin and paracingulin suggests that they may interact with centrosomal proteins, and possibly recruit them to junctions in confluent monolayers. As discussed below, cingulin and paracingulin are also implicated in the junctional recruitment of MT-associated signaling proteins (GEFs and GAPs) that regulate the organization and contractility of the actomyosin cytoskeleton. In summary, the identification of both cingulin and paracingulin as MT-binding protein, and their centrosomal localization reinforce the notion of a dynamic re-localization of centrosome-associated proteins to apical junctions, once these are formed following epithelial differentiation ()).
Another potential regulator of MTs at the TJ is the apical polarity complex protein Par3, which in mammalian systems is involved in epithelial apicobasal polarization, and in the junctional recruitment of the exocyst complex.Citation78-Citation81 In invertebrate model systems and in neuronal cells and fibroblasts Par3 regulates MT stability and organization, and forms cortical docking sites for centrosomes, subjected to modulation by Par1 and aPKC.Citation82-Citation84 Par3 associates with the TJ transmembrane protein occludin during polarized migration of MDCK cells, and in this context occludin is required for the organization of the MT network and the orientation of the MTOC during migration.Citation85 However, the specific roles of Par3 and occludin in the regulation of MT organization at epithelial junctions have not been investigated.
The cadherin-catenin complex, which is clustered at AJ of epithelial and non-epithelial cells, has been implicated in MT anchoring through different types of catenin-dependent molecular interactions. Early studies showed that exogenous expression of either E-cadherin or N-cadherin in CHO fibroblasts, which do not express either cadherin, stabilizes non-centrosomal MTs.Citation86 Experiments on astrocytes confirmed that classical cadherins control nucleus and centrosome positioning.Citation87 It was further shown that only membrane targeting of α-catenin, but not β-catenin or p120-catenin (p120-ctn), led to a significant increase in MT length and density in centrosome-free cytoplasts, suggesting that α-catenin can regulate MT dynamics in a centrosome-independent manner.Citation88 This could be due to the fact that α-catenin recruits the centrosomal component CAP350 to the AJ, and can thus bind to MTs through CAP350.Citation59 β-catenin is another AJ protein involved in MT interaction, since it has been identified at centrosomes of non-epithelial cells, where it may anchor MTs,Citation89 and regulate centrosome cohesion.Citation90,Citation91 In addition, β-catenin overexpression disrupts the localization of the minus-end motor dynein, and dramatically perturbs the organization of MT arrays, suggesting that β-catenin may anchor MTs to AJ through dynein.Citation92 Finally, several observations support the idea that p120-ctn interacts and cross-talks with MTs. In COS7 cells, mutated exogenous p120-ctn localizes along MTs in interphase, and recruitment to MT suppresses the ability of p120-ctn to regulate Rho GTPases.Citation93 In MDCK cells p120-ctn binding to either cadherin or MTs is mutually exclusive, and promoting p120-ctn association to MTs by overexpressing its indirect linker kinesin reduces the nuclear pool of p120-ctn.Citation94 MTs plus-ends undergo more frequent rapid depolymerization in p120-ctn-depleted cells, suggesting that p120-ctn can regulate MT dynamics.Citation95 Finally, in mouse primary keratinocytes p120-ctn interacts directly with the plus-end capping protein CLASP2, and recruits it to AJ of basal epidermal progenitors, where CLASP2 functions to promote AJ formation and stability.Citation96
An important mechanism of MTs-AJ interaction was discovered with the identification of PLEKHA7, which binds to p120-ctn and to the minus-end MT-binding protein nezha/CAMSAP3, thus promoting the stabilization of the ZA through the accumulation of E-cadherin.Citation61 In agreement, overexpression of PLEKHA7 attenuates, in a MT-dependent manner, the disruption of junctions and the increase in paracellular TJ-dependent permeability induced by depletion of extracellular calcium.Citation97 PLEKHA7 binds to additional ZA proteins, such as paracingulin,Citation74,Citation98 afadinCitation99 and PDZD11.Citation100 PLEKHA7 is also involved in recruiting mRNAs and miRNA processing factors to the ZA, modulating cellular resistance to staphylococcal α-toxin and controlling the pathogenesis of hypertension and angle closure glaucoma, through mechanisms that remain to be clarified (reviewed inCitation101). However, the role of MT-tethering in all these additional interactions and functions of PLEKHA7 is not clear.
Desmoplakin (DP), which links intermediate filaments to the desmoglein/desmocollin complex ()) (reviewed inCitation102,Citation103), plays a crucial role in MTs rearrangement, through the desmosomal recruitment of MT-binding centrosome proteins, such as ninein, LIS1, Ndel1, and CLIP170.Citation104,Citation105 The recruitment of these MT-binding proteins by desmoplakin during keratinocyte differentiation facilitates MTs reorganization, and provides new nucleation sites, thus promoting MT alignment along the cell cortex.
In summary, several junctional proteins interact either directly or indirectly with MTs, and thus provide cortical sites for the MT-dependent delivery of additional junctional components, as well as tethering MTs during their spatial reorganization of differentiating cells. These interactions are crucial to stabilize junctions, and to establish an architectural framework for the cross-talk between MT and actin cytoskeleton.
Junctions as molecular sinks and regulators for MT-associated signaling proteins
Zonular junctions (TJ and ZA) are sites where transmembrane proteins, cytoplasmic adaptors and signaling proteins are highly concentrated and clustered in a thin circumferential plaque, which is intimately associated with the actin and MT cytoskeletons. Contractility of the actin cytoskeleton affects the conformation of selected TJ and ZA proteins within the cluster, such as ZO-1, α-catenin, and vinculin,Citation21,Citation22 resulting in mechanical reinforcement and enhanced junctional recruitment of signaling and regulatory proteins.Citation21,Citation106 Among proteins found in the cytoplasmic region of zonular junctions there are several proteins that bind either directly or indirectly to MTs, including proteins that redistribute from centrosomes to junctions during epithelial differentiation, and proteins involved in the regulation of Rho family GTPases and actomyosin contractility, such as GEFs and GAPsCitation19,Citation106 (). The recruitment of these signaling, adaptor and regulatory proteins to zonular junctions can result in their functional inhibition, or, alternatively, may spatially restrict their activity at junctions. We propose that zonular junctions act as “sinks”, e.g. either storage, and/or spatial restriction, and/or silencing of signaling and adaptor proteins, in a manner which couples the integrity of cell-cell contacts to the dynamic regulation of the actin and MT cytoskeletons. Indeed, the observation that several junctional proteins, including cingulin, paracingulin,Citation35 β-cateninCitation90,Citation107 and occludinCitation108 are localized at centrosomes suggests that epithelial polarization and junction assembly results in the redistribution of a subset of centrosomal proteins to the zonular “sink”. Below we provide a few examples of proteins that have been functionally and/or spatially linked to both junctions and MTs (), and review the current knowledge about the molecular basis and functional implications of their localization at junctions, and/or association with MTs.
Figure 3. Zonular junctions as sinks for MT-associated signaling proteins. TJ and ZA are shown in a schematic cartoon of an apical junction between epithelial cell, with an apical cilium shown on the right. Cytoplasmic plaque “clusters” of junctional proteins are indicated by diffuse red (TJ) and blue (ZA/AJ) “clouds”, showing only a few of the junctional molecules, for simplicity (see for graphical legend). On the right, the signaling proteins described in the text are indicated, with color-coded arrows (red-TJ, blue-AJ/ZA) linking them to the junctional structures with which they have been associated, based on current literature. For MARKs, only MARK2 and MARK4 have been localized at apical junctions and in cilia basal bodies, respectively. Each protein or group of proteins is also associated with a text, on the right, describing their function in relationship to either MTs or Rho family GTPases.
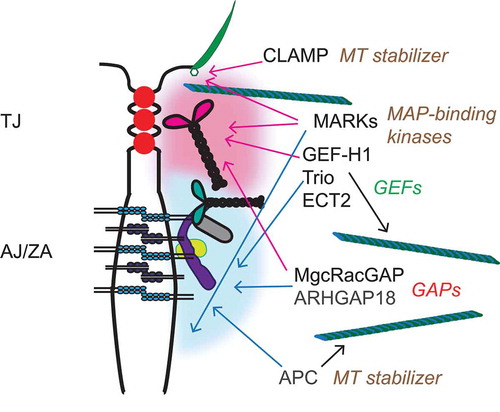
GEF-H1
GEF-H1 (ARHGEF2) is a Dbl family guanidine exchange factor (GEF), which was originally identified as a MT-associated activator of RhoA.Citation109 The activity of GEF-H1 is inhibited when it is bound to MTs, since mutants that do not bind to MTs constitutively activate RhoA.Citation110 GEF-H1 is a key molecule involved in the cross-talk between MTs and the actin cytoskeleton.Citation111 For example, in endothelial cells GEF-H1 plays a critical role in RhoA activation and downstream actomyosin contractility and endothelial barrier disruption caused by MT disassembly, induced either by thrombin or by mechanical strain.Citation112,Citation113 RhoA activation by GEF-H1 is involved not only in promoting the formation of contractile cytoplasmic stress fibers, but also in inducing apical constriction during development,Citation114 establishing epithelial polarity,Citation115 regulating cell proliferation, transcription factors and other signaling molecules.Citation116-Citation118 Because of its multiple roles in disease, it was proposed that GEF-H1 could be a therapeutic target for pathologies where RhoA activity is dysregulated.Citation119
Besides binding to MTs, GEF-H1 is also regulated by phosphorylation and by recruitment to junctions. The interaction with MTs, and hence the inhibition of GEF-H1 catalytic activity, is promoted by phosphorylation at Ser886, either by The Rac1/Cdc42 effector p21-activated kinase (PAK1), or by protein kinase A.120,Citation121 In contrast, GEF-H1 phosphorylation at Ser151 by the MARK3 kinase disrupts the association of GEF-H1 with MTs, and thus activates it, resulting in development of epithelial polarity and cyst lumen formation.Citation115 During mitosis the catalytic activity of GEF-H1 is inhibited, prior to cytokinesis, through phosphorylation by the mitotic kinases Aurora A/B and Cdk1/Cyclin B.Citation122 Another mechanism through which GEF-H1 activity is inhibited is its recruitment to junctions, which depends on cingulinCitation123 (). Upon either depletion of cingulin in cultured cells, or KO of cingulin in either embryoid bodies or in mice, GEF-H1 loses its junctional localization, resulting in RhoA activation, and RhoA-dependent transcriptional changes, such as increased expression in the TJ transmembrane protein claudin-2.Citation123-Citation126 Thus, the junctional sequestration of GEF-H1 by cingulin provides a simple mechanism to couple cell confluency to downregulation of RhoA activity, both in epithelial and endothelial cells.Citation127 However, it is not clear whether direct binding to cingulin, which has been proposed based on in vitro experiments,Citation123 functions as a regulator of either the catalytic activity or the phosphorylation of GEF-H1. Another TJ protein, ZO-2, was recently shown to have a role in promoting GEF-H1 inhibition through phosphorylation.Citation128
MgcRacGAP and ECT2
MgcRacGAP, together with the kinesin-6-family member MKLP1 (mitotic kinesin-like protein), forms the centralspindlin complex, which organizes antiparallel arrays of MTs at the spindle midzone and midbody, controls the activity of RhoA and Rac1 during furrow ingression, and links the mitotic spindle to the plasma membrane during cytokinesis.Citation129 ECT2 is a Rho GEF, which concentrates on the central spindle by binding to centralspindlin, and is essential for RhoA activation in the cleavage furrow.Citation130,Citation131 MgcRacGAP localizes to MT plus-ends at the equatorial cortex as cytokinesis initiates, and it tracks with EB3 at growing MT plus-ends.Citation132 In contrast, in interphase confluent epithelial cells, MgcRacGAP accumulates at apical junctions, where it is recruited by cingulin, paracingulin and α-cateninCitation133,Citation134 (). In some but not all cell types the centralspindlin complex recruits the ECT2 to the ZACitation133,Citation134 (). MgcRacGAP regulates AJ but not TJ structure, through its function as a GTPase activating protein (GAP) activity, and signaling via the RhoA pathway.Citation135 The C-terminal region of MgcRacGAP is involved to its targeting both to AJ, via a SxIP motif,Citation132 and to TJ, via its interaction with cingulin and paracingulin.Citation134 These observations suggest that initially MgcRacGAP is delivered to AJ through plus-end directed motors, and then is redistributed at both TJ and AJ through multiple interactions.
ARHGAP18
The RhoGAP ARHGAP18/SENEX was identified in a screen for genes involved in angiogenesis, and is highly expressed in endothelial cells, where it is downregulated in the early migration phase, and upregulated in the stabilization phase of tube formation, when junctions are formed.Citation136 Indeed, ARHGAP18 translocates to and stabilizes junctions during angiogenesisCitation137 (). Consistent with its role in stabilizing endothelial junctions, loss of ARHGAP18 promotes tumor angiogenesis,Citation137 and, conversely, its expression is associated with good breast cancer prognosis.Citation138 In HUVEC endothelial cells, ARHGAP18 is associated with MTs, and ARHGAP18 depletion decreases total MT network density and acetylation levels,Citation139 which regulate the ability of the MT lattice to cope with mechanical stress and self-repair.Citation140 However, nothing is known about the mechanisms through which ARHGAP18 associates with junctions, and how it may affect tubulin acetylation.
Trio
Trio (ARHGEF23) is a RhoGEF, which promotes localized Rac1 activation, and is targeted to the plus-ends of MTs during neurite extension, through its interaction with the +TIPs NAV and EB1.Citation141 In endothelial cells Trio is involved in the stabilization of VE-cadherin.Citation142 In epithelial cells it interacts with E-cadherin and mediates the junctional recruitment of Tara, which regulates E-cadherin transcriptionCitation143 (). However, it is not known whether Trio activity as a GEF is modulated by its MT versus junctional localizations.
APC
APC is a gene mutated in familial colorectal cancer, which encodes a large protein involved in the regulation of the cytoplasmic pool of β-catenin.Citation144 APC binds to MTs, either directly or through the +TIPs EB1 or CLIP170, and its binding to MTs is regulated by phosphorylation, and results in MT polymerization and stabilizationCitation145-Citation149 (). Studies on cultured cells show that two spatially separate populations of APC exist at the cell periphery, one which is highly dynamic and associates with MTs near free edges, and a second comparatively static and closely associated with actin at sites of cell-cell contactCitation150,Citation151 (). However, the molecular basis for APC association with junctions, and how the junctional localization of APC is related to its signaling activity is not clear.
MARK proteins
Microtubule affinity-regulating kinases (MARKs) are an evolutionarily conserved family of kinases that comprise MARK1(PAR-1c), MARK2 (PAR-1b/EMK), MARK3 (PAR-1a/C-TAK1) and MARK4 (PAR-1d/MARKL1).Citation152 Mammalian MARKs are homologous to the invertebrate partitioning gene product Par-1, which regulates development of cell polarity,Citation153 and belong to the same family of energy sensing kinases as AMPK.Citation154 MARKs are indirectly associated with MTs, since they were identified as kinases that phosphorylate the MT-regulating proteins tau and MAPs, to regulate MT dynamics and MT-dependent transport.Citation155 There is evidence that MARK proteins are involved in different aspects of epithelial differentiation. For example, MARK4 localizes to the basal body and promotes ciliogenesis in ciliated cells,Citation156 and MARK2 functions downstream of aPKC in the establishment and maintenance of epithelial cell polarity in mammalian cellsCitation157 (). Importantly, MARK2 (PAR1b) has been localized both at apical and lateral epithelial junctions,Citation157 and is required for the formation of apical lumens in epithelial cells grown in 3D, by promoting the apicobasal alignment of MTs, and by modulating myosin-II and E-cadherin dependent signalingCitation158,Citation159 (). MARKs are involved in regulation of different signaling pathways, for example through the phosphorylation of proteins involved in membrane recycling,Citation160 RhoA/Rac1 signaling,Citation115,Citation161,Citation162 inflammation,Citation163 and the Hippo pathway.Citation164 However, although MARK2 has been localized at junctions,Citation157,Citation158 little is known about the junctional localization of other MARKs (MARK1, MARK3 and MARK4), the mechanisms that regulate the subcellular localizations of MARKs, and the effect of junctional localization on the enzymatic activity and signaling functions of MARK proteins.
CLAMP
CLAMP/Spef1 is a MT-associated protein which is highly expressed in ciliated epithelia, stabilizes MTs, and has been implicated in the apical accumulation of stable acetylated MTs.Citation165 Recently, it was shown that CLAMP/Spef1 accumulates not only at MT-rich structures, but also at junctions, and it controls planar polarity and MT asymmetry in Xenopus ciliated epitheliaCitation166 (). CLAMP co-localizes with components of the Par complex and interacts with aPKC, suggesting that the Par complex directs the junctional localization of CLAMP.Citation165 However, it is not clear how the junctional localization of CLAMP affects the PTMs and dynamics of MTs.
Conclusions
The dynamics and the reorganization of the MT network are fundamentally important in the formation of cell-cell junctions and in the biogenesis of the epithelial phenotype. MTs serve as tracks for the delivery of vesicles containing junctional and signaling proteins to the cell periphery, contributing to generation of apical lumens, efficient assembly of junctions, apico-basal polarization, and differentiation. In a feed-forward loop, the formation of junctions promotes the local clustering of cytoplasmic plaque proteins, several of which interact either with MT or MT-binding proteins, and help to redundantly orient, tether, and stabilize MTs along the apicobasal axis of polarized cells. Among these proteins, the MT-binding proteins cingulin and paracingulin may form docking sites for apical MT structures, such as the planar apical network. Other junctional proteins, such as PLEKHA7, can represent transient docking sites for MT-binding proteins, such as CAMSAP3/nezha, which redistribute apically in fully differentiated cells. The junctional localization of several centrosome-associated proteins underlines the crucial role of junctions as sites that orchestrate MT organization in polarizing cells. Besides nucleating the formation of a molecular environment rich in MT-binding proteins, cytoplasmic junctional proteins also promote the junctional recruitment and storage of MT-associated signaling proteins, several of which play crucial roles in the regulation of the actomyosin cytoskeleton. The release of these signaling proteins during junction disassembly contributes to the reorganization of both actin and MT cytoskeleton, leading to changes in cell motility and behavior. Thus, junctions act both as organizers of MTs, as “molecular sinks” for MT-associated proteins, and as signaling hubs, which modulate the assembly and dynamics of both MT and actin cytoskeletons locally and globally in the cell.
Disclosure of potential conflicts of interest
No potential conflicts of interest were disclosed.
Acknowledgments
We thank members of the Citi laboratory and Paul Guichard for their comments on the manuscript, and the Swiss National Foundation (n. 31003A_172809, SC), and the Novartis Foundation for Medical and Biological Research (#17B072, SC) for financial support.
Additional information
Funding
References
- Pollard TD, Goldman RD. Overview of the cytoskeleton from an evolutionary perspective. Cold Spring Harb Perspect Biol. 2018;10(7):a030288. doi:10.1101/cshperspect.a030288.
- Tilney LG, Bryan J, Bush DJ, Fujiwara K, Mooseker MS, Murphy DB, Snyder DH. Microtubules: evidence for 13 protofilaments. J Cell Biol. 1973;59(2 Pt 1):267–275.
- Desai A, Mitchison TJ. Microtubule polymerization dynamics. Annu Rev Cell Dev Biol. 1997;13:83–117. doi:10.1146/annurev.cellbio.13.1.83.
- Martin M, Akhmanova A. Coming into Focus: mechanisms of Microtubule Minus-End Organization. Trends Cell Biol. 2018;28(7):574–588. doi:10.1016/j.tcb.2018.02.011.
- Gadadhar S, Bodakuntla S, Natarajan K, Janke C. The tubulin code at a glance. J Cell Sci. 2017;130(8):1347–1353. doi:10.1242/jcs.199471.
- Muroyama A, Lechler T. Microtubule organization, dynamics and functions in differentiated cells. Development. 2017;144(17):3012–3021. doi:10.1242/dev.153171.
- Bacallao R, Antony C, Dotti C, Karsenti E, Stelzer EH, Simons K. The subcellular organization of Madin-Darby Canine Kidney cells during the formation of a polarized epithelium. J Cell Biol. 1989;109:2817–2832.
- Bré MH, Pepperkok R, Hill AM, Levilliers N, Ansorge W, Stelzer EH, Karsenti E. Regulation of microtubule dynamics and nucleation during polarization in MDCK II cells. J Cell Biol. 1990;111(6 Pt 2):3013–3021.
- Waterman-Storer CM, Salmon WC, Salmon ED. Feedback interactions between cell-cell adherens junctions and cytoskeletal dynamics in newt lung epithelial cells. Mol Biol Cell. 2000;11(7):2471–2483. doi:10.1091/mbc.11.7.2471.
- Yano T, Matsui T, Tamura A, Uji M, Tsukita S. The association of microtubules with tight junctions is promoted by cingulin phosphorylation by AMPK. J Cell Biol. 2013;203(4):605–614. doi:10.1083/jcb.201304194.
- Tanaka N, Meng W, Nagae S, Takeichi M. Nezha/CAMSAP3 and CAMSAP2 cooperate in epithelial-specific organization of noncentrosomal microtubules. Proc Natl Acad Sci U S A. 2012;109(49):20029–20034. doi:10.1073/pnas.1218017109.
- Jiang K, Hua S, Mohan R, Grigoriev I, Yau KW, Liu Q, Katrukha EA, Altelaar AFM, Heck AJR, Hoogenraad CC, et al. Microtubule minus-end stabilization by polymerization-driven CAMSAP deposition. Dev Cell. 2014;28(3):295–309. doi:10.1016/j.devcel.2014.01.001.
- Toya M, Kobayashi S, Kawasaki M, Shioi G, Kaneko M, Ishiuchi T, Misaki K, Meng W, Takeichi M. CAMSAP3 orients the apical-to-basal polarity of microtubule arrays in epithelial cells. Proc Natl Acad Sci U S A. 2016;113(2):332–337. doi:10.1073/pnas.1520638113.
- Farquhar MG, Palade GE. Junctional complexes in various epithelia. J Cell Biol. 1963;17:375–412.
- Takeichi M. Dynamic contacts: rearranging adherens junctions to drive epithelial remodelling. Nat Rev Mol Cell Biol. 2014;15(6):397–410. doi:10.1038/nrm3802.
- Godsel, L.M., et al. The molecular composition and function of desmosomes. Handb Exp Pharmacol. 2004;165:137–193.
- Giannotta M, Trani M, Dejana E. VE-cadherin and endothelial adherens junctions: active guardians of vascular integrity. Dev Cell. 2013;26(5):441–454. doi:10.1016/j.devcel.2013.08.020.
- Umeda K, Ikenouchi J, Katahira-Tayama S, Furuse K, Sasaki H, Nakayama M, Matsui T, Tsukita S, Furuse M, Tsukita S. ZO-1 and ZO-2 independently determine where claudins are polymerized in tight-junction strand formation. Cell. 2006;126(4):741–754. doi:10.1016/j.cell.2006.06.043.
- Citi S, Guerrera D, Spadaro D, Shah J. Epithelial junctions and Rho family GTPases: the zonular signalosome. Small GTPases. 2014;5(4):1–15. doi:10.4161/21541248.2014.973760.
- Wen W, Zhang M. Protein complex assemblies in epithelial cell polarity and asymmetric cell division. J Mol Biol. 2017;430:3504–3520.
- Charras G, Yap AS. Tensile forces and mechanotransduction at cell-cell junctions. Curr Biol. 2018;28(8):R445–R457. doi:10.1016/j.cub.2018.02.003.
- Spadaro D, Le S, Laroche T, Mean I, Jond L, Yan J, Citi S. Tension-dependent stretching activates ZO-1 to control the junctional localization of its interactors. Curr Biol. 2017;27(24):3783–3795.e8. doi:10.1016/j.cub.2017.11.014.
- Hatzfeld M, Keil R, Magin TM. Desmosomes and intermediate filaments: their consequences for tissue mechanics. Cold Spring Harb Perspect Biol. 2017;9(6):a029157. doi:10.1101/cshperspect.a029157.
- Guillemot L, Paschoud S, Pulimeno P, Foglia A, Citi S. The cytoplasmic plaque of tight junctions: a scaffolding and signalling center. Biochim Biophys Acta Biomembr. 2008;1778(3):601–613. doi:10.1016/j.bbamem.2007.09.032.
- Green KJ, Getsios S, Troyanovsky S, Godsel LM. Intercellular junction assembly, dynamics, and homeostasis. Cold Spring Harb Perspect Biol. 2010;2(2):a000125. doi:10.1101/cshperspect.a000125.
- Van Itallie CM, Anderson JM. Architecture of tight junctions and principles of molecular composition. Semin Cell Dev Biol. 2014;36:157–165. doi:10.1016/j.semcdb.2014.08.011.
- Meng W, Takeichi M. Adherens junction: molecular architecture and regulation. Cold Spring Harb Perspect Biol. 2009;1:a002899–a002899. doi:10.1101/cshperspect.a002899.
- Niessen CM, Gottardi CJ. Molecular components of the adherens junction. Biochim Biophys Acta. 2008;1778(3):562–571. doi:10.1016/j.bbamem.2007.12.015.
- Mandai K, Rikitake Y, Mori M, Takai Y. Nectins and nectin-like molecules in development and disease. Curr Top Dev Biol. 2015;112:197–231. doi:10.1016/bs.ctdb.2014.11.019.
- Furuse M, Tsukita S. Claudins in occluding junctions of humans and flies. Trends Cell Biol. 2006;16(4):181–188. doi:10.1016/j.tcb.2006.02.006.
- Lecuit T, Yap AS. E-cadherin junctions as active mechanical integrators in tissue dynamics. Nat Cell Biol. 2015;17(5):533–539. doi:10.1038/ncb3136.
- Meza I, Ibarra G, Sabanero M, Martínez-Palomo A, Cereijido M. Occluding junctions and cytoskeletal components in a cultured transporting epithelium. J Cell Biol. 1980;87:746–754.
- Rassat J, Robenek H, Themann H. Alterations of tight and gap junctions in mouse hepatocytes following administration of colchicine. Cell Tissue Res. 1982;223(1):187–200.
- Yap AS, Stevenson BR, Abel KC, Cragoe EJ, Manley SW. Microtubule integrity is necessary for the epithelial barrier function of cultured thyroid cell monolayers. Exp Cell Res. 1995;218(2):540–550. doi:10.1006/excr.1995.1189.
- Paschoud S, Yu D, Pulimeno P, Jond L, Turner JR, Citi S. Cingulin and paracingulin show similar dynamic behaviour, but are recruited independently to junctions. Mol Membr Biol. 2011;28(2):123–135. doi:10.3109/09687688.2010.538937.
- Glotfelty LG, Zahs A, Iancu C, Shen L, Hecht GA. Microtubules are required for efficient epithelial tight junction homeostasis and restoration. Am J Physiol Cell Physiol. 2014;307(3):C245–54. doi:10.1152/ajpcell.00336.2013.
- Simard-Bisson C, Bidoggia J, Larouche D, Guérin SL, Blouin R, Hirai S-I, Germain L. A role for DLK in microtubule reorganization to the cell periphery and in the maintenance of desmosomal and tight junction integrity. J Invest Dermatol. 2017;137(1):132–141. doi:10.1016/j.jid.2016.07.035.
- Hsu C-Y, Lecland N, Pendaries V, Viodé C, Redoulès D, Paul C, Merdes A, Simon M, Bierkamp C. Stabilization of microtubules restores barrier function after cytokine-induced defects in reconstructed human epidermis. J Dermatol Sci. 2018;91(1):87–96. doi:10.1016/j.jdermsci.2018.04.008.
- Fesenko I, Kurth T, Sheth B, Fleming TP, Citi S, Hausen P. Tight junction biogenesis in the early Xenopus embryo. Mech Dev. 2000;96:51–65.
- Li D, Kuehn EW, Prekeris R. Kinesin-2 mediates apical endosome transport during epithelial lumen formation. Cell Logist. 2014;4(1):e28928. doi:10.4161/cl.28928.
- Mangan AJ, Sietsema DV, Li D, Moore JK, Citi S, Prekeris R. Cingulin and actin mediate midbody-dependent apical lumen formation during polarization of epithelial cells. Nat Commun. 2016;7:12426. doi:10.1038/ncomms12426.
- Ivanov AI, McCall IC, Babbin B, Samarin SN, Nusrat A, Parkos CA. Microtubules regulate disassembly of epithelial apical junctions. BMC Cell Biol. 2006;7:12. doi:10.1186/1471-2121-7-12.
- Citi S. Protein kinase inhibitors prevent junction dissociation induced by low extracellular calcium in MDCK epithelial cells. J Cell Biol. 1992;117(1):169–178.
- Citi S, Volberg T, Bershadsky AD, Denisenko N, Geiger B. Cytoskeletal involvement in the modulation of cell-cell junctions by the protein kinase inhibitor H-7. J Cell Sci. 1994;107(3):683–692.
- Ivanov AI, McCall IC, Parkos CA, Nusrat A. Role for actin filament turnover and a myosin II motor in cytoskeleton-driven disassembly of the epithelial apical junctional complex. Mol Biol Cell. 2004;15(6):2639–2651. doi:10.1091/mbc.e04-02-0163.
- Ivanov AI, Bachar M, Babbin BA, Adelstein RS, Nusrat A, Parkos CA, Cordes N. A unique role for nonmuscle myosin heavy chain IIA in regulation of epithelial apical junctions. PLoS One. 2007;2(7):e658. doi:10.1371/journal.pone.0000658.
- Samarin SN, Ivanov AI, Flatau G, Parkos CA, Nusrat A. Rho/Rho-associated kinase-II signaling mediates disassembly of epithelial apical junctions. Mol Biol Cell. 2007;18(9):3429–3439. doi:10.1091/mbc.e07-04-0315.
- Ito S, Okuda S, Abe M, Fujimoto M, Onuki T, Nishimura T, Takeichi M. Induced cortical tension restores functional junctions in adhesion-defective carcinoma cells. Nat Commun. 2017;8(1):1834. doi:10.1038/s41467-017-01945-y.
- Shultz T, Shmuel M, Hyman T, Altschuler Y. Beta-tubulin cofactor D and ARL2 take part in apical junctional complex disassembly and abrogate epithelial structure. FASEB J. 2008;22(1):168–182. doi:10.1096/fj.06-7786com.
- Mary S, Charrasse S, Meriane M, Comunale F, Travo P, Blangy A, Gauthier-Rouvière C. Biogenesis of N-cadherin-dependent cell-cell contacts in living fibroblasts is a microtubule-dependent kinesin-driven mechanism. Mol Biol Cell. 2002;13(1):285–301. doi:10.1091/mbc.01-07-0337.
- Chen X, Kojima S-I, Borisy GG, Green KJ. p120 catenin associates with kinesin and facilitates the transport of cadherin-catenin complexes to intercellular junctions. J Cell Biol. 2003;163(3):547–557. doi:10.1083/jcb.200305137.
- Teng J, Rai T, Tanaka Y, Takei Y, Nakata T, Hirasawa M, Kulkarni AB, Hirokawa N. The KIF3 motor transports N-cadherin and organizes the developing neuroepithelium. Nat Cell Biol. 2005;7(5):474–482. doi:10.1038/ncb1249.
- Acharya BR, Espenel C, Libanje F, Raingeaud J, Morgan J, Jaulin F, Kreitzer G. KIF17 regulates RhoA-dependent actin remodeling at epithelial cell-cell adhesions. J Cell Sci. 2016;129(5):957–970. doi:10.1242/jcs.173674.
- Ligon LA, Holzbaur ELF. Microtubules tethered at epithelial cell junctions by dynein facilitate efficient junction assembly. Traffic. 2007;8(7):808–819. doi:10.1111/j.1600-0854.2007.00574.x.
- Bellett G, Carter JM, Keynton J, Goldspink D, James C, Moss DK, Mogensen MM. Microtubule plus-end and minus-end capture at adherens junctions is involved in the assembly of apico-basal arrays in polarised epithelial cells. Cell Motil Cytoskeleton. 2009;66(10):893–908. doi:10.1002/cm.20393.
- Stehbens SJ, Paterson AD, Crampton MS, Shewan AM, Ferguson C, Akhmanova A, Parton RG, Yap AS. Dynamic microtubules regulate the local concentration of E-cadherin at cell-cell contacts. J Cell Sci. 2006;119(Pt 9):1801–1811. doi:10.1242/jcs.02903.
- Rowning BA, Wells J, Wu M, Gerhart JC, Moon RT, Larabell CA. Microtubule-mediated transport of organelles and localization of beta-catenin to the future dorsal side of Xenopus eggs. Proc Natl Acad Sci U S A. 1997;94(4):1224–1229.
- Moss DK, Bellett G, Carter JM, Liovic M, Keynton J, Prescott AR, Lane EB, Mogensen MM. Ninein is released from the centrosome and moves bi-directionally along microtubules. J Cell Sci. 2007;120(Pt 17):3064–3074. doi:10.1242/jcs.010322.
- Gavilan MP, Arjona M, Zurbano A, Formstecher E, Martinez-Morales JR, Bornens M, Rios RM, Gumbiner BM. Alpha-catenin-dependent recruitment of the centrosomal protein CAP350 to adherens junctions allows epithelial cells to acquire a columnar shape. PLoS Biol. 2015;13(3):e1002087. doi:10.1371/journal.pbio.1002087.
- Goldspink DA, Rookyard C, Tyrrell BJ, Gadsby J, Perkins J, Lund EK, Galjart N, Thomas P, Wileman T, Mogensen MM. Ninein is essential for apico-basal microtubule formation and CLIP-170 facilitates its redeployment to non-centrosomal microtubule organizing centres. Open Biol. 2017;7(2):160274. doi:10.1098/rsob.160274.
- Meng W, Mushika Y, Ichii T, Takeichi M. Anchorage of microtubule minus ends to adherens junctions regulates epithelial cell-cell contacts. Cell. 2008;135(5):948–959. doi:10.1016/j.cell.2008.09.040.
- Sako-Kubota K, Tanaka N, Nagae S, Meng W, Takeichi M. Minus end-directed motor KIFC3 suppresses E-cadherin degradation by recruiting USP47 to adherens junctions. Mol Biol Cell. 2014;25(24):3851–3860. doi:10.1091/mbc.E14-07-1245.
- Pasdar M, Krzeminski KA, Nelson WJ. Regulation of desmosome assembly in MDCK epithelial cells: coordination of membrane core and cytoplasmic plaque domain assembly at the plasma membrane. J Cell Biol. 1991;113:645–655.
- Nekrasova OE, Amargo EV, Smith WO, Chen J, Kreitzer GE, Green KJ. Desmosomal cadherins utilize distinct kinesins for assembly into desmosomes. J Cell Biol. 2011;195(7):1185–1203. doi:10.1083/jcb.201106057.
- Sumigray KD, Chen H, Lechler T. Lis1 is essential for cortical microtubule organization and desmosome stability in the epidermis. J Cell Biol. 2011;194(4):631–642. doi:10.1083/jcb.201104009.
- Robitaille H, Proulx R, Robitaille K, Blouin R, Germain L. The mitogen-activated protein kinase kinase kinase dual leucine zipper-bearing kinase (DLK) acts as a key regulator of keratinocyte terminal differentiation. J Biol Chem. 2005;280(13):12732–12741. doi:10.1074/jbc.M411619200.
- McHarg S, Hopkins G, Lim L, Garrod D, Klymkowsky M. Down-regulation of desmosomes in cultured cells: the roles of PKC, microtubules and lysosomal/proteasomal degradation. PLoS One. 2014;9(10):e108570. doi:10.1371/journal.pone.0108570.
- Citi S, Sabanay H, Jakes R, Geiger B, Kendrick-Jones J. Cingulin, a new peripheral component of tight junctions. Nature. 1988;333:272–276. doi:10.1038/333272a0.
- Citi S, Sabanay H, Kendrick-Jones J, Geiger B. Cingulin: characterization and localization. J Cell Sci. 1989;93(1):107–122.
- Lizcano JM, Göransson O, Toth R, Deak M, Morrice NA, Boudeau J, Hawley SA, Udd L, Mäkelä TP, Hardie DG, et al. LKB1 is a master kinase that activates 13 kinases of the AMPK subfamily, including MARK/PAR-1. Embo J. 2004;23(4):833–843. doi:10.1038/sj.emboj.7600110.
- Porat-Shliom N, Tietgens AJ, Van Itallie CM, Vitale-Cross L, Jarnik M, Harding OJ, Anderson JM, Gutkind JS, Weigert R, Arias IM. Liver kinase B1 regulates hepatocellular tight junction distribution and function in vivo. Hepatology. 2016;64(4):1317–1329. doi:10.1002/hep.28724.
- D’Atri F, Citi S. Cingulin interacts with F-actin in vitro. FEBS Lett. 2001;507:21–24.
- Ohnishi H, Nakahara T, Furuse K, Sasaki H, Tsukita S, Furuse M. JACOP, a novel plaque protein localizing at the apical junctional complex with sequence similarity to cingulin. J Biol Chem. 2004;279(44):46014–46022. doi:10.1074/jbc.M402616200.
- Pulimeno P, Paschoud S, Citi S. A role for ZO-1 and PLEKHA7 in recruiting paracingulin to tight and adherens junctions of epithelial cells. J Biol Chem. 2011;286(19):16743–16750. doi:10.1074/jbc.M111.230862.
- Umeda K, Matsui T, Nakayama M, Furuse K, Sasaki H, Furuse M, Tsukita S. Establishment and characterization of cultured epithelial cells lacking expression of ZO-1. J Biol Chem. 2004;279(43):44785–44794. doi:10.1074/jbc.M406563200.
- D’Atri F, Nadalutti F, Citi S. Evidence for a functional interaction between cingulin and ZO-1 in cultured cells. J Biol Chem. 2002;277(31):27757–27764. doi:10.1074/jbc.M203717200.
- Andersen JS, Wilkinson CJ, Mayor T, Mortensen P, Nigg EA, Mann M. Proteomic characterization of the human centrosome by protein correlation profiling. Nature. 2003;426(6966):570–574. doi:10.1038/nature02166.
- Lin D, Edwards AS, Fawcett JP, Mbamalu G, Scott JD, Pawson T. A mammalian PAR-3-PAR-6 complex implicated in Cdc42/Rac1 and aPKC signalling and cell polarity. Nat Cell Biol. 2000;2(8):540–547. doi:10.1038/35019582.
- Joberty G, Petersen C, Gao L, Macara IG. The cell-polarity protein Par6 links Par3 and atypical protein kinase C to Cdc42. Nat Cell Biol. 2000;2(8):531–539. doi:10.1038/35019573.
- Mizuno K, Suzuki A, Hirose T, Kitamura K, Kutsuzawa K, Futaki M, Amano Y, Ohno S. Self-association of PAR-3-mediated by the conserved N-terminal domain contributes to the development of epithelial tight junctions. J Biol Chem. 2003;278(33):31240–31250. doi:10.1074/jbc.M303593200.
- Ahmed SM, Macara IG. The Par3 polarity protein is an exocyst receptor essential for mammary cell survival. Nat Commun. 2017;8:14867. doi:10.1038/ncomms14867.
- Schmoranzer J, Fawcett JP, Segura M, Tan S, Vallee RB, Pawson T, Gundersen GG. Par3 and dynein associate to regulate local microtubule dynamics and centrosome orientation during migration. Curr Biol. 2009;19(13):1065–1074. doi:10.1016/j.cub.2009.05.065.
- Chen S, Chen J, Shi H, Wei M, Castaneda-Castellanos DR, Bultje RS, Pei X, Kriegstein AR, Zhang M, Shi S-H. Regulation of microtubule stability and organization by mammalian Par3 in specifying neuronal polarity. Dev Cell. 2013;24(1):26–40. doi:10.1016/j.devcel.2012.11.014.
- Jiang T, McKinley RFA, McGill MA, Angers S, Harris TJC. A Par-1-Par-3-Centrosome cell polarity pathway and its tuning for isotropic cell adhesion. Curr Biol. 2015;25(20):2701–2708. doi:10.1016/j.cub.2015.08.063.
- Du D, Xu F, Yu L, Zhang C, Lu X, Yuan H, Huang Q, Zhang F, Bao H, Jia L, et al. The tight junction protein, occludin, regulates the directional migration of epithelial cells. Dev Cell. 2010;18(1):52–63. doi:10.1016/j.devcel.2009.12.008.
- Chausovsky A, Bershadsky AD, Borisy GG. Cadherin-mediated regulation of microtubule dynamics. Nat Cell Biol. 2000;2(11):797–804. doi:10.1038/35041037.
- Dupin I, Camand E, Etienne-Manneville S. Classical cadherins control nucleus and centrosome position and cell polarity. J Cell Biol. 2009;185(5):779–786. doi:10.1083/jcb.200812034.
- Shtutman M, Chausovsky A, Prager-Khoutorsky M, Schiefermeier N, Boguslavsky S, Kam Z, Fuchs E, Geiger B, Borisy GG, Bershadsky AD. Signaling function of alpha-catenin in microtubule regulation. Cell Cycle. 2008;7(15):2377–2383. doi:10.4161/cc.6362.
- Huang P, Senga T, Hamaguchi M. A novel role of phospho-beta-catenin in microtubule regrowth at centrosome. Oncogene. 2007;26(30):4357–4371. doi:10.1038/sj.onc.1210217.
- Bahmanyar S, Kaplan DD, Deluca JG, Giddings TH, O’Toole ET, Winey M, Salmon ED, Casey PJ, Nelson WJ, Barth AIM. beta-Catenin is a Nek2 substrate involved in centrosome separation. Genes Dev. 2008;22(1):91–105. doi:10.1101/gad.1596308.
- Bahmanyar S, Guiney EL, Hatch EM, Nelson WJ, Barth AIM. Formation of extra centrosomal structures is dependent on beta-catenin. J Cell Sci. 2010;123(Pt 18):3125–3135. doi:10.1242/jcs.064782.
- Ligon LA, Karki S, Tokito M, Holzbaur EL. Dynein binds to beta-catenin and may tether microtubules at adherens junctions. Nat Cell Biol. 2001;3(10):913–917. doi:10.1038/ncb1001-913.
- Franz CM, Ridley AJ. p120 catenin associates with microtubules: inverse relationship between microtubule binding and Rho GTPase regulation. J Biol Chem. 2004;279(8):6588–6594. doi:10.1074/jbc.M312812200.
- Yanagisawa M, Kaverina IN, Wang A, Fujita Y, Reynolds AB, Anastasiadis PZ. A novel interaction between kinesin and p120 modulates p120 localization and function. J Biol Chem. 2004;279(10):9512–9521. doi:10.1074/jbc.M310895200.
- Ichii T, Takeichi M. p120-catenin regulates microtubule dynamics and cell migration in a cadherin-independent manner. Genes Cells. 2007;12(7):827–839. doi:10.1111/j.1365-2443.2007.01095.x.
- Shahbazi MN, Megias D, Epifano C, Akhmanova A, Gundersen GG, Fuchs E, Perez-Moreno M. CLASP2 interacts with p120-catenin and governs microtubule dynamics at adherens junctions. J Cell Biol. 2013;203(6):1043–1061.
- Paschoud S, Jond L, Guerrera D, Citi S. PLEKHA7 modulates epithelial tight junction barrier function. Tissue Barriers. 2014;2(2):e28755. doi:10.4161/tisb.28755.
- Pulimeno P, Bauer C, Stutz J, Citi S, Arkowitz RA. PLEKHA7 is an adherens junction protein with a tissue distribution and subcellular localization distinct from ZO-1 and E-Cadherin. PLoS One. 2010;5(8):e12207. doi:10.1371/journal.pone.0012207.
- Kurita S, Yamada T, Rikitsu E, Ikeda W, Takai Y. Binding between the junctional proteins afadin and PLEKHA7 and implication in the formation of adherens junction in epithelial cells. J Biol Chem. 2013;288(41):29356–29368. doi:10.1074/jbc.M113.453464.
- Guerrera D, Shah J, Vasileva E, Sluysmans S, Méan I, Jond L, Poser I, Mann M, Hyman AA, Citi S. PLEKHA7 recruits PDZD11 to adherens junctions to stabilize nectins. J Biol Chem. 2016;291(21):11016–11029. doi:10.1074/jbc.M115.712935.
- Shah J, Guerrera D, Vasileva E, Sluysmans S, Bertels E, Citi S. PLEKHA7: cytoskeletal adaptor protein at center stage in junctional organization and signaling. Int J Biochem Cell Biol. 2016;75:112–116. doi:10.1016/j.biocel.2016.04.001.
- Franke WW, Cowin P, Schmelz M, Kapprell HP. The desmosomal plaque and the cytoskeleton. Ciba Found Symp. 1987;125:26–48.
- Garrod D, Chidgey M. Desmosome structure, composition and function. Biochim Biophys Acta. 2008;1778(3):572–587. doi:10.1016/j.bbamem.2007.07.014.
- Lechler T, Fuchs E. Desmoplakin: an unexpected regulator of microtubule organization in the epidermis. J Cell Biol. 2007;176(2):147–154. doi:10.1083/jcb.200609109.
- Sumigray KD, Lechler T. Control of cortical microtubule organization and desmosome stability by centrosomal proteins. Bioarchitecture. 2011;1(5):221–224. doi:10.4161/bioa.18403.
- Sluysmans S, Vasileva E, Spadaro D, Shah J, Rouaud F, Citi S. The role of apical cell-cell junctions and associated cytoskeleton in mechanotransduction. Biol Cell. 2017;109(4):139–161. doi:10.1111/boc.201600075.
- Mbom BC, Nelson WJ, Barth A. beta-catenin at the centrosome: discrete pools of beta-catenin communicate during mitosis and may co-ordinate centrosome functions and cell cycle progression. Bioessays. 2013;35(9):804–809. doi:10.1002/bies.201300045.
- Runkle EA, Sundstrom JM, Runkle KB, Liu X, Antonetti DA. Occludin localizes to centrosomes and modifies mitotic entry. J Biol Chem. 2011;286(35):30847–30858. doi:10.1074/jbc.M111.262857.
- Ren Y, Li R, Zheng Y, Busch H. Cloning and characterization of GEF-H1, a microtubule-associated guanine nucleotide exchange factor for Rac and Rho GTPases. J Biol Chem. 1998;273(52):34954–34960.
- Krendel M, Zenke FT, Bokoch GM. Nucleotide exchange factor GEF-H1 mediates cross-talk between microtubules and the actin cytoskeleton. Nat Cell Biol. 2002;4(4):294–301. doi:10.1038/ncb773.
- Chang Y-C, Nalbant P, Birkenfeld J, Chang Z-F, Bokoch GM. GEF-H1 couples nocodazole-induced microtubule disassembly to cell contractility via RhoA. Mol Biol Cell. 2008;19(5):2147–2153. doi:10.1091/mbc.e07-12-1269.
- Birukova AA, Adyshev D, Gorshkov B, Bokoch GM, Birukov KG, Verin AD. GEF-H1 is involved in agonist-induced human pulmonary endothelial barrier dysfunction. Am J Physiol Lung Cell Mol Physiol. 2006;290(3):L540–8. doi:10.1152/ajplung.00259.2005.
- Birukova AA, Fu P, Xing J, Yakubov B, Cokic I, Birukov KG. Mechanotransduction by GEF-H1 as a novel mechanism of ventilator-induced vascular endothelial permeability. Am J Physiol Lung Cell Mol Physiol. 2010;298(6):L837–48. doi:10.1152/ajplung.00263.2009.
- Itoh K, Ossipova O, Sokol SY. GEF-H1 functions in apical constriction and cell intercalations and is essential for vertebrate neural tube closure. J Cell Sci. 2014;127(Pt 11):2542–2553. doi:10.1242/jcs.146811.
- Sandí M-J, Marshall CB, Balan M, Coyaud É, Zhou M, Monson DM, Ishiyama N, Chandrakumar AA, La Rose J, Couzens AL, et al. MARK3-mediated phosphorylation of ARHGEF2 couples microtubules to the actin cytoskeleton to establish cell polarity. Sci Signal. 2017;10(503):eaan3286. doi:10.1126/scisignal.aan3286.
- Nie M, Aijaz S, Leefa Chong San IV, Balda MS, Matter K. The Y-box factor ZONAB/DbpA associates with GEF-H1/Lfc and mediates Rho-stimulated transcription. EMBO Rep. 2009;10(10):1125–1131. doi:10.1038/embor.2009.182.
- Cullis J, Meiri D, Sandi MJ, Radulovich N, Kent OA, Medrano M, Mokady D, Normand J, Larose J, Marcotte R, et al. The RhoGEF GEF-H1 is required for oncogenic RAS signaling via KSR-1. Cancer Cell. 2014;25(2):181–195. doi:10.1016/j.ccr.2014.01.025.
- Dubois F, Keller M, Calvayrac O, Soncin F, Hoa L, Hergovich A, Parrini M-C, Mazières J, Vaisse-Lesteven M, Camonis J, et al. RASSF1A suppresses the invasion and metastatic potential of human non-small cell lung cancer cells by inhibiting YAP activation through the GEF-H1/RhoB pathway. Cancer Res. 2016;76(6):1627–1640. doi:10.1158/0008-5472.CAN-15-1008.
- Birkenfeld J, Nalbant P, Yoon S-H, Bokoch GM. Cellular functions of GEF-H1, a microtubule-regulated Rho-GEF: is altered GEF-H1 activity a crucial determinant of disease pathogenesis? Trends Cell Biol. 2008;18:210–219. doi:10.1016/j.tcb.2008.02.006.
- Zenke FT, Krendel M, DerMardirossian C, King CC, Bohl BP, Bokoch GM. p21-activated kinase 1 phosphorylates and regulates 14-3-3 binding to GEF-H1, a microtubule-localized Rho exchange factor. J Biol Chem. 2004;279(18):18392–18400. doi:10.1074/jbc.M400084200.
- Meiri D, Greeve MA, Brunet A, Finan D, Wells CD, LaRose J, Rottapel R. Modulation of Rho guanine exchange factor Lfc activity by protein kinase A-mediated phosphorylation. Mol Cell Biol. 2009;29(21):5963–5973. doi:10.1128/MCB.01268-08.
- Birkenfeld J, Nalbant P, Bohl BP, Pertz O, Hahn KM, Bokoch GM. GEF-H1 modulates localized RhoA activation during cytokinesis under the control of mitotic kinases. Dev Cell. 2007;12(5):699–712. doi:10.1016/j.devcel.2007.03.014.
- Aijaz S, D’Atri F, Citi S, Balda MS, Matter K. Binding of GEF-H1 to the tight junction-associated adaptor cingulin results in inhibition of Rho signaling and G1/S phase transition. Dev Cell. 2005;8(5):777–786. doi:10.1016/j.devcel.2005.03.003.
- Guillemot L, Hammar E, Kaister C, Ritz J, Caille D, Jond L, Bauer C, Meda P, Citi S. Disruption of the cingulin gene does not prevent tight junction formation but alters gene expression. J Cell Sci. 2004;117(Pt 22):5245–5256. doi:10.1242/jcs.01399.
- Guillemot L, Citi S. Cingulin regulates claudin-2 expression and cell proliferation through the small GTPase RhoA. Mol Biol Cell. 2006;17(8):3569–3577. doi:10.1091/mbc.e06-02-0122.
- Guillemot L, Schneider Y, Brun P, Castagliuolo I, Pizzuti D, Martines D, Jond L, Bongiovanni M, Citi S. Cingulin is dispensable for epithelial barrier function and tight junction structure, and plays a role in the control of claudin-2 expression and response to duodenal mucosa injury. J Cell Sci. 2012;125:5005–5012. doi:10.1242/jcs.101261.
- Tian Y, Gawlak G, Tian X, Shah AS, Sarich N, Citi S, Birukova AA. Cingulin role in agonist-induced vascular endothelial permeability. J Biol Chem. 2016;291:23681–23692. doi:10.1074/jbc.M116.720763.
- Raya-Sandino A, Castillo-Kauil A, Domínguez-Calderón A, Alarcón L, Flores-Benitez D, Cuellar-Perez F, López-Bayghen B, Chávez-Munguía B, Vázquez-Prado J, González-Mariscal L. Zonula occludens-2 regulates Rho proteins activity and the development of epithelial cytoarchitecture and barrier function. Biochim Biophys Acta. 2017;1864(10):1714–1733. doi:10.1016/j.bbamcr.2017.05.016.
- White EA, Glotzer M. Centralspindlin: at the heart of cytokinesis. Cytoskeleton (Hoboken). 2012;69(11):882–892. doi:10.1002/cm.21065.
- Yuce O, Piekny A, Glotzer M. An ECT2-centralspindlin complex regulates the localization and function of RhoA. J Cell Biol. 2005;170(4):571–582. doi:10.1083/jcb.200501097.
- Su KC, Takaki T, Petronczki M. Targeting of the RhoGEF Ect2 to the equatorial membrane controls cleavage furrow formation during cytokinesis. Dev Cell. 2011;21(6):1104–1115. doi:10.1016/j.devcel.2011.11.003.
- Breznau EB, Murt M, Blasius TL, Verhey KJ, Miller AL. The MgcRacGAP SxIP motif tethers Centralspindlin to microtubule plus ends in Xenopus laevis. J Cell Sci. 2017;130(10):1809–1821. doi:10.1242/jcs.195891.
- Ratheesh A, Gomez GA, Priya R, Verma S, Kovacs EM, Jiang K, Brown NH, Akhmanova A, Stehbens SJ, Yap AS. Centralspindlin and alpha-catenin regulate Rho signalling at the epithelial zonula adherens. Nat Cell Biol. 2012;14(8):818–828. doi:10.1038/ncb2532.
- Guillemot L, Guerrera D, Spadaro D, Tapia R, Jond L, Citi S. MgcRacGAP interacts with cingulin and paracingulin to regulate Rac1 activation and development of the tight junction barrier during epithelial junction assembly. Mol Biol Cell. 2014;25(13):1995–2005. doi:10.1091/mbc.E13-11-0680.
- Breznau EB, Semack AC, Higashi T, Miller AL. MgcRacGAP restricts active RhoA at the cytokinetic furrow and both RhoA and Rac1 at cell-cell junctions in epithelial cells. Mol Biol Cell. 2015;26(13):2439–2455. doi:10.1091/mbc.E14-11-1553.
- Coleman PR, Hahn CN, Grimshaw M, Lu Y, Li X, Brautigan PJ, Beck K, Stocker R, Vadas MA, Gamble JR. Stress-induced premature senescence mediated by a novel gene, SENEX, results in an anti-inflammatory phenotype in endothelial cells. Blood. 2010;116(19):4016–4024. doi:10.1182/blood-2009-11-252700.
- Chang GHK, Lay AJ, Ting KK, Zhao Y, Coleman PR, Powter EE, Formaz-Preston A, Jolly CJ, Bower NI, Hogan BM, et al. ARHGAP18: an endogenous inhibitor of angiogenesis, limiting tip formation and stabilizing junctions. Small GTPases. 2014;5(3):1–15. doi:10.4161/21541248.2014.975002.
- Aleskandarany MA, Sonbul S, Surridge R, Mukherjee A, Caldas C, Diez-Rodriguez M, Ashankyty I, Albrahim KI, Elmouna AM, Aneja R, et al. Rho-GTPase activating-protein 18: a biomarker associated with good prognosis in invasive breast cancer. Br J Cancer. 2017;117(8):1176–1184. doi:10.1038/bjc.2017.261.
- Lovelace MD, Powter EE, Coleman PR, Zhao Y, Parker A, Chang GH, Lay AJ, Hunter J, McGrath AP, Jormakka M, et al. The RhoGAP protein ARHGAP18/SENEX localizes to microtubules and regulates their stability in endothelial cells. Mol Biol Cell. 2017;28(8):1066–1078. doi:10.1091/mbc.E16-05-0285.
- Janke C, Montagnac G. Causes and consequences of microtubule acetylation. Curr Biol. 2017;27(23):R1287–R1292. doi:10.1016/j.cub.2017.10.044.
- van Haren J, Boudeau J, Schmidt S, Basu S, Liu Z, Lammers D, Demmers J, Benhari J, Grosveld F, Debant A, et al. Dynamic microtubules catalyze formation of navigator-TRIO complexes to regulate neurite extension. Curr Biol. 2014;24(15):1778–1785. doi:10.1016/j.cub.2014.06.037.
- Timmerman I, Heemskerk N, Kroon J, Schaefer A, van Rijssel J, Hoogenboezem M, van Unen J, Goedhart J, Gadella TWJ, Yin T, et al. A local VE-cadherin and Trio-based signaling complex stabilizes endothelial junctions through Rac1. J Cell Sci. 2015;128(18):3514. doi:10.1242/jcs.179424.
- Yano T, Yamazaki Y, Adachi M, Okawa K, Fort P, Uji M, Tsukita S, Tsukita S. Tara up-regulates E-cadherin transcription by binding to the Trio RhoGEF and inhibiting Rac signaling. J Cell Biol. 2011;193(2):319–332. doi:10.1083/jcb.201009100.
- Gumbiner BM. Carcinogenesis: a balance between beta-catenin and APC. Curr Biol. 1997;7(7):R443–6.
- Munemitsu S, Souza B, Müller O, Albert I, Rubinfeld B, Polakis P. The APC gene product associates with microtubules in vivo and promotes their assembly in vitro. Cancer Res. 1994;54(14):3676–3681.
- Zumbrunn J, Kinoshita K, Hyman AA, Näthke IS. Binding of the adenomatous polyposis coli protein to microtubules increases microtubule stability and is regulated by GSK3 beta phosphorylation. Curr Biol. 2001;11(1):44–49.
- Wen Y, Eng CH, Schmoranzer J, Cabrera-Poch N, Morris EJS, Chen M, Wallar BJ, Alberts AS, Gundersen GG. EB1 and APC bind to mDia to stabilize microtubules downstream of Rho and promote cell migration. Nat Cell Biol. 2004;6(9):820–830. doi:10.1038/ncb1160.
- Morrison EE. The APC-EB1 interaction. Adv Exp Med Biol. 2009;656:41–50.
- Watanabe T, Wang S, Noritake J, Sato K, Fukata M, Takefuji M, Nakagawa M, Izumi N, Akiyama T, Kaibuchi K. Interaction with IQGAP1 links APC to Rac1, Cdc42, and actin filaments during cell polarization and migration. Dev Cell. 2004;7(6):871–883. doi:10.1016/j.devcel.2004.10.017.
- Rosin-Arbesfeld R, Ihrke G, Bienz M. Actin-dependent membrane association of the APC tumour suppressor in polarized mammalian epithelial cells. Embo J. 2001;20(21):5929–5939. doi:10.1093/emboj/20.21.5929.
- Langford KJ, Askham J, Lee T, Adams M, Morrison E. Examination of actin and microtubule dependent APC localisations in living mammalian cells. BMC Cell Biol. 2006;7:3. doi:10.1186/1471-2121-7-3.
- Wu Y, Griffin EE. Regulation of Cell Polarity by PAR-1/MARK Kinase. Curr Top Dev Biol. 2017;123:365–397. doi:10.1016/bs.ctdb.2016.11.001.
- Guo S, Kemphues KJ. par-1, a gene required for establishing polarity in C. elegans embryos, encodes a putative Ser/Thr kinase that is asymmetrically distributed. Cell. 1995;81(4):611–620.
- Hurov J, Piwnica-Worms H. The Par-1/MARK family of protein kinases: from polarity to metabolism. Cell Cycle. 2007;6(16):1966–1969. doi:10.4161/cc.6.16.4576.
- Drewes G, Ebneth A, Preuss U, Mandelkow EM, Mandelkow E. MARK, a novel family of protein kinases that phosphorylate microtubule-associated proteins and trigger microtubule disruption. Cell. 1997;89(2):297–308.
- Kuhns S, Schmidt KN, Reymann J, Gilbert DF, Neuner A, Hub B, Carvalho R, Wiedemann P, Zentgraf H, Erfle H, et al. The microtubule affinity regulating kinase MARK4 promotes axoneme extension during early ciliogenesis. J Cell Biol. 2013;200(4):505–522. doi:10.1083/jcb.201206013.
- Suzuki A, Hirata M, Kamimura K, Maniwa R, Yamanaka T, Mizuno K, Kishikawa M, Hirose H, Amano Y, Izumi N, et al. aPKC acts upstream of PAR-1b in both the establishment and maintenance of mammalian epithelial polarity. Curr Biol. 2004;14(16):1425–1435. doi:10.1016/j.cub.2004.08.021.
- Cohen D, Brennwald PJ, Rodriguez-Boulan E, Müsch A. Mammalian PAR-1 determines epithelial lumen polarity by organizing the microtubule cytoskeleton. J Cell Biol. 2004;164(5):717–727. doi:10.1083/jcb.200308104.
- Cohen D, Tian Y, Musch A. Par1b promotes hepatic-type lumen polarity in Madin Darby canine kidney cells via myosin II- and E-cadherin-dependent signaling. Mol Biol Cell. 2007;18(6):2203–2215. doi:10.1091/mbc.e07-02-0095.
- Ducharme NA, Hales CM, Lapierre LA, Ham A-JL, Oztan A, Apodaca G, Goldenring JR. MARK2/EMK1/Par-1Balpha phosphorylation of Rab11-family interacting protein 2 is necessary for the timely establishment of polarity in Madin-Darby canine kidney cells. Mol Biol Cell. 2006;17(8):3625–3637. doi:10.1091/mbc.e05-08-0736.
- Cohen D, Fernandez D, Lázaro-Diéguez F, Müsch A. The serine/threonine kinase Par1b regulates epithelial lumen polarity via IRSp53-mediated cell-ECM signaling. J Cell Biol. 2011;192(3):525–540. doi:10.1083/jcb.201007002.
- Yamahashi Y, Saito Y, Murata-Kamiya N, Hatakeyama M. Polarity-regulating kinase partitioning-defective 1b (PAR1b) phosphorylates guanine nucleotide exchange factor H1 (GEF-H1) to regulate RhoA-dependent actin cytoskeletal reorganization. J Biol Chem. 2011;286(52):44576–44584. doi:10.1074/jbc.M111.267021.
- Li X, Thome S, Ma X, Amrute-Nayak M, Finigan A, Kitt L, Masters L, James JR, Shi Y, Meng G, et al. MARK4 regulates NLRP3 positioning and inflammasome activation through a microtubule-dependent mechanism. Nat Commun. 2017;8:15986. doi:10.1038/ncomms15986.
- Heidary Arash E, Shiban A, Song S, Attisano L. MARK4 inhibits Hippo signaling to promote proliferation and migration of breast cancer cells. EMBO Rep. 2017;18(3):420–436. doi:10.15252/embr.201642455.
- Werner ME, Mitchell JW, Putzbach W, Bacon E, Kim SK, Mitchell BJ. Radial intercalation is regulated by the Par complex and the microtubule-stabilizing protein CLAMP/Spef1. J Cell Biol. 2014;206(3):367–376. doi:10.1083/jcb.201312045.
- Kim SK, Zhang S, Werner ME, Brotslaw EJ, Mitchell JW, Altabbaa MM, Mitchell BJ. CLAMP/Spef1 regulates planar cell polarity signaling and asymmetric microtubule accumulation in the Xenopus ciliated epithelia. J Cell Biol. 2018;217(5):1633–1641. doi:10.1083/jcb.201706058.