Abstract
Recently, it has been disclosed that silver nanoparticles (AgNPs) have the potential to inhibit infection and cancerous cells and eventually penetrate through injected site into the capillary due to their small size. This study focuses on the effect of size and zeta potential of bare and citrate-coated AgNPs on human umbilical vein endothelial cells (HUVECs) as main capillary cells. AgNPs with high and low concentrations and no citrate coating were synthesized by using simple wet chemical method and named as AgNP/HC, AgNP/LC, and AgNP, respectively. Citrate coated particles showed larger zeta potential of −22 mV and AgNp/HC showed the smallest size of 13.2 nm. UV-Visible spectroscopy and dynamic light scattering (DLS) were performed to evaluate particle size and hydrodynamic diameter of NPs in water and cell culture media. Results indicated that higher concentrations of citrate decreased hydrodynamic diameter and NP agglomeration. reactive oxygen species (ROS) production of all AgNPs was similar at 28 ppm although it was significantly higher than control group. Their effects on cell membrane and chromosomal structure were studied using LDH measurement and 4′,6-diamidino-2-phenylindole (DAPI) staining, as well. Results demonstrated that AgNP/LC was less toxic to cells owing to higher value of IC50, minimum inhibitory concentration (MIC), and less release of LDH. Cancerous (Human Caucasian neuroblastoma) and immortal cells (Mouse embryonic fibroblast cell line) were about twice more sensitive than HUVECs to toxic effects of AgNPs. DAPI staining results showed that AgNP and AgNP/HC induced highest and lowest breaking of chromosome. Overall results suggest that viability of HUVECs will be higher than 90% when viability of cancerous cells is 50% in AgNPs chemotherapy.
Introduction
Infection and cancer are two major problems in healthcare. However, drug resistance has been a major concern especially in nosocomial infection of some bacteria such as Methicillin-Resistant Staphylococcus aureus, extended Spectrum β-Lactamase producer Enterobacteriaceae, Vancomycin resistant Enterococci, Imipenem resistant multi drug Resistant Pseudomons aerginosa, and Imipenem resistant multi drug resistant Acinetobacter baumannii (Bennett and St Geme III Citation1999).
During the last decade, silver nanoparticles (AgNPs) have been widely investigated and used due to their strong antibacterial properties (Batarseh and Smith Citation2012, Hekmat et al. Citation2012, Ma et al. Citation2011, Nirmala et al. Citation2012, Sotiriou and Pratsinis Citation2011, Tavakol et al. Citation2014). The antibacterial potential of AgNPs is influenced by the novel properties of the nanoparticle (NP) and has not been fully understood. Some mechanisms have been suggested for these effects. Increase of cytosolic calcium, impairment of respiratory chain in mitochondria and afterward disturbance in synthesis of ATP, breaking of DNA, protein carbonilation and lipidic membrane peroxidation are likely to be the effective mechanisms of bactericidal properties. Although, use of AgNPs is expected to continue, antibacterial resistance to AgNP via plasmid gene of pMG101 has been suggested (Eckhardt et al. Citation2013, Gupta et al. Citation2001). It is demonstrated that AgNPs through similar mechanisms influence eukaryotic cells and damage proteins etc. However, disruptive effects will decrease at controlled concentrations.
Recently, some anticancer mechanisms of AgNPs have been reported which are different from their effect on normal cells. The anticancer mechanism of AgNPs is related to activation of apoptosis via caspase cascade pathway and increased level of BAX/BCL-XL ratio, p53 and decreased level of NF-κB and AKT. Inhibition of AKT signaling pathway results in decreasing cell survival and vascular permeability and angiogenesis. Cancerous cells via capillary develop cancers and metastasis to other organs (Tavakol Citation2014, Vaidyanathan et al. Citation2009). Based on these findings, if AgNP is considered as a therapeutic agent, its influence on vein endothelial cells will be important.
The first step leading to toxic effects at the cellular level is uptake. During this process, cell membrane undergoes gelation–fluid transition and cell membrane permeability might increase (Cho et al. Citation2009, Seiler et al. Citation1975, Wu et al. Citation2012). To affect on cell organelles or biomolecules, AgNP must either pass through the cell membrane and/or facilitate access of any ionic Ag. There are some key properties of NPs such as size, surface charge and production of reactive oxygen species (ROS) which rule their interaction with cells.
Size provides important control over many of the physical and chemical properties of nanoscale materials and their interaction with biomolecules and cell organelles. NPs with smaller particle size and higher surface/volume ratio can enter into the cells and mitochondria easier. Higher surface area, more exposed atoms and active sites on the surface of small AgNPs plays an important damaging role in cell through interaction with proteins, therefore they can affect on organelles such as mitochondria stronger than larger ones.
Zeta potential of the NPs is another critical factor leading to different cell responses as compared to particle size. Zeta potential is electrical charge on the surface of material surrounded in the medium. Higher zeta potential results in higher stability and assimilation to the body because substances with high zeta potential do not tend to adhere or clump to each other (Clogston and Patri Citation2011). Zeta potential values less than −14 mv usually represent the onset of agglomeration. Charge of NP also is an important factor in cell membrane damage. Fluid–gelation or gelation–fluid transition in cell membrane local phase is a key factor of NP internalization. NPs with significantly weaker negative charge could induce gelation–fluid transition (biphasic) and could enter into the cell easier. Particles with higher negative charge can induce gelation phase and decreased internalization (Cho et al. Citation2009, Nel et al. Citation2009, Seiler et al. Citation1975).
ROS have the absence of one electron on the chemical structure and when materials of negative charge interact with ROS, they neutralize free radicals and increase cell viability. Restoring of oxidizing function of organelles in cancerous cells is ideal for cancer therapy (Gerson Citation2002).
By regards to this fact that vascular endothelial cells are critical cells in angiogenesis, tissue engineering as well as in tumor treatment, and also treatment with AgNPs in infection and tumor sites may pass them to the vein and capillary so in the present work, to the best of our knowledge for the first time we aimed at parallely investigating the effects of particle size, zeta potential, and ROS production of bare AgNP, AgNP with low and high citrate coatings on viability, membrane damage and changes in chromosomal structure of human umbilical vein endothelial cells (HUVEC) and compare HUVEC viability with two cancerous and immortal cells at different concentrations and zeta potential.
Materials and methods
Synthesis of AgNPs
Three samples of AgNPs were synthesized by using a simple wet chemical method by chemical reduction of Ag+ ions in water, in presence and absence of tri sodium citrate as stabilizer. Since tri sodium citrate is an ionic surfactant, by varying the concentration of the surfactant, the surface charge on the particles can be manipulated. For preparation of bare AgNPs, O(0).5 ml, 0.1 M ice cooled solution of NaBH4 (99.0%, Merck, Darmstadt, Germany) was injected into 12 ml, 0.5 mM solution of AgNO3·2H2O (99.9%, Sigma). These particles were named as AgNP. For synthesis of citrate coated particles, 0.5 ml of 0.01 and 0.2 M solutions of tri sodium citrate (99.0%, Merck, Darmstadt, Germany) were initially mixed and stirred with AgNO3 solution. Then, 0.5 ml, 0.04 M solution of ice cooled NaBH4 was injected into this. These samples were named as AgNP/LC and AgNP/HC, respectively. The synthesis parameters for all three samples are listed in .
Table 1. Characteristics of synthesized AgNPs.
Characterization of AgNPs
Determination of particle size and zeta potential
Particle size of three AgNPs was estimated triplicate by means of UV-Visible absorption spectra (CARY-100-Bio mod) and origin software. Then, to investigate hydrodynamic diameter of particles in face to media, dynamic light scattering (DLS) was performed at different time point of 2, 10, 20, and 30 min post-treatment with media.
Measurement of zeta potential of three AgNPs was carried out using dynamic light scattering (DLS) (Brookhaven Instruments Corporation, Holtsville, NY) at adjusted pH. The average values were calculated based on the data from six runs.
Study of minimum inhibitory concentration (MIC) of bacteria
MIC was determined by using microdilution method. The mixtures containing AgNPs of concentrations of 2, 4, 6, 8, 10, 12, 14, 16, 18, 20, 22, 24, and 26 ppm in LB medium and Escherichia coli (ATCC5922) and Pseudomonas aeruginosa (ATCC27853) at 105–106 CFU/ml were prepared and incubated in triplicate into separate 96 well plates at 37 °C for 24 h. After incubation, the MIC of AgNP, AgNP/LC, and AgNP/HC were determined using optical density of incubated mixtures.
Determination of free radical scavenger property
1,1-Diphenyl-2-picryl-hydrazyl (DPPH) with an unpaired valence electron has free radical scavenger potential and was applied as antioxidant assay. It was purchased from Sigma-Aldrich (St. Louis, MO) and diluted in methanol (100 μM). AgNP, AgNP/LC, and AgNP/HC were prepared at concentrations of 2 and 28 ppm in methanol. After the incubation time of 24 h, 100 μl of 100 μM solution of DPPH in methanol was added to 100 μl of NP solution and was kept in 30 °C for 30 min and the absorbance was measured using Eliza reader at 517 nm (Fisher Lifesciences, Germany).
In vitro studies
HUVEC isolation
HUVEC were obtained within 24 h of normal deliveries in cold Dulbecco’s phosphate-buffered saline (DPBS) containing antibiotics. The umbilical vein was rinsed free of blood, filled with 1 mg/ml collagenase type IA dissolved in hanks media and incubated for 15 min at 37 °C. At the end of the incubation period of the vein, digestive juice was collected and centrifuged (1200 rpm for 10 min), then washed with M199 and centrifuged again (1200 rpm for 10 min) and cultured with 20% EGM in DMEM with 4 mM l-glutamine. The content were adjusted to 1.5 g/L sodium bicarbonate, 150 mg/ml endothelial cell growth factor, 20 U/ml heparin, 4.5 g/L glucose, 1.0 mM sodium pyruvate, 100 IU/ml penicillin, 50 ng/ml streptomycin, and 90% DMEM +20% FBS. Culture flasks were maintained at 37 °C in a humidified atmosphere of 5% CO2.
Cell culture purity was characterized by using endothelial cell markers (PECAM1 and von Willebrand Factor; Abcam, Germany) and by means of lack of smooth muscle actin and cell surface fibroblast markers. All assays were performed between passages 4–6.
Study of cell membrane damage
LDH assay was performed to study the cell membrane damage. HUVEC (1 × 104 cell/ml) was seeded in triplicate into 96 well plate for 24 h in RPMI medium poor of pyruvate (As pyruvate induces false positive in LDH assay) supplemented with 1% antibiotic penicillin/streptomycin (Gibco, Gaithersburg, MD). NPs were prepared in concentration of 2 and 28 ppm in serum free and poor pyruvate RPMI medium (Gibco, Gaithersburg, MD). Media in 96-well-plate were changed to the AgNP solutions and then incubated at 37 °C in 5% CO2 for 24 h. Then, 100 μl of supernatant from each well were removed. This output was transferred into corresponding wells, and then 100 μL/well reaction mixture was added and incubated about 30 min at 15–25 °C. Absorbance was read in triplicate at 490 nm in 96-well format using ELIZA reader.
Study of cell viability
Human Caucasian neuroblastoma (BE (2)M17) (NB) and Mouse embryonic fibroblast cell line (STO) as immortal cells and HUVEC as normal cell (1 × 104 cell/ml) were seeded in triplicate 96 well plate and incubated for 24 h in RPMI medium supplemented with 15% fetal bovin serum (FBS). AgNP, AgNP/LC and AgNP/HC were prepared in concentrations of 2, 4, 6, 8, 10, 12, 14, 16, 18, 20, 22, 24, and 26 ppm in RPMI medium (Gibco, Gaithersburg, MD) containing 15% FBS (Gibco, Gaithersburg, MD) and 1% antibiotic penicillin/streptomycin (Gibco, Gaithersburg, MD). Media in 96 well plate were changed to the NP solutions and were then incubated at 37 °C in 5% CO2 for 24 h. The MTT solution 3-(4,5-dimethylthiazol-2-yl)-2,5 diphenyltetrazolium bromide was added into wells and was incubated for 4 h and then DMSO was added. Absorbance was read at 570 nm using ELIZA reader. Based on results of MTT assay, the half maximal inhibitory concentration (IC50) was determined.
Morphology observation and counting of nucleolus
4′,6-Diamidino-2-phenylindole (DAPI) staining was carried out to investigate chromatin nucleus staining as DNA fragmentation and nucleus number in HUVEC treated with three types of NPs in 2, 12, and 24 ppm concentrations after 48 h. Cells were washed in cold PBS. They were fixed in 4% paraformaldehyde, then DAPI (1 mg/ml) was added and left for 15 s and was then washed in PBS. Images were recorded using an inverted fluorescence microscope (Olympus AX-800, Hamburg, Germany) equipped with digital camera (Leica, DC200, Wetzlar, Germany).
Statistical analysis
The Graph pad software was applied to calculate and analyze ROS production, MIC, LDH release, MTT assay data using One-way ANOVA tests. P < 0.05 was found statistically significant.
Results
Particle size and zeta potential
UV-Visible spectroscopy was performed to determine average particle size in three different AgNPs. In this study, citrate was added as surfactant in two reaction mixture of NPs at different concentrations. Particle size of AgNP (17.8 nm) was close to AgNP/LC (17.2 nm), but larger than AgNP/HC (13.2 nm).
Measurement of zeta potential showed that AgNP (−9 mV) had weaker negative zeta potential than others and citrated AgNPs had similar zeta potential and there was no significant difference between them.
DLS was performed to investigate hydrodynamic diameter of NPs in face to media at different time points. Results showed that although, AgNP/HC had smaller hydrodynamic diameter as compared to others at different time points but eventually due to media compartment–NPs interaction, all NPs had ascending order of size with time. AgNP/LC had the highest difference of particle size in presence and absence of cell culture media. However, based on UV-Visible spectroscopy and DLS data, AgNP/HC has the smallest size and the lowest potential of cell agglomeration. Data are presented in .
Minimum inhibitory concentration
Minimum inhibitory concentration (MIC) of three NPs against E. coli and S. aeurogenosa strains was determined by microdilution method. Results indicated that AgNPs coated with tri-sodium citrate were ineffective against S. aeurogenosa at all concentrations. Lowest and highest MIC values were related to AgNP at 14 ppm and AgNP/HC at 26 ppm against E. coli, respectively ().
Determination of free radical scavenger property
DPPH assay was performed to evaluate free radical scavenging property of particles at 2 and 28 ppm. Results showed that bare AgNPs induced significant free radical values as compared to citrate-coated and control group at 2 ppm, however there was no significant difference between productions of free radical among citrate coated NPs. At 28 ppm, there was a significant increase of free radical of coated and bare particles as compared to control group but this difference was not significant among samples ().
Figure 2. (a) Determination of free radical scavenger property at 2 and 28 ppm. Bare AgNPs induced significant free radical values as compared to low and high citrate coated AgNPs at 2 ppm (p <0.05 and p <0.01, respectively). At 28 ppm concentration the p values was 0.6681, considered not significant difference between ROS production of AgNPs. (b) LDH release from HUVECs treated with NPs. AgNPs induced non-significant free radical values as compared to each others at 2 ppm (p values was 0.0602). At 28 ppm concentration, although there was no significant difference between ROS production of bare and high citrate coated AgNPs, but bare AgNP and AgNP/HC induced higher LDH release as compared to AgNP/LC (p values was <0.001).
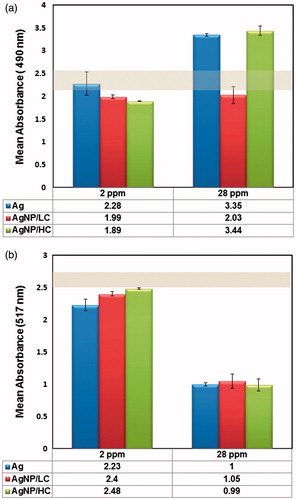
LDH release
LDH release was assessed as a sign of damage to cell membrane. Results showed that LDH release from HUVEC cells treated with NPs was not significantly higher than the control group at 2 ppm. However, damage to cell membrane, even at high concentrations of AgNP/LC, was not significant as compared to negative control. AgNP and AgNP/HC induced higher LDH release as compared to AgNP/LC and control group at 28 ppm ().
Cell viability
MTT was done to investigate cell viability and maximum half inhibitory concentration (IC50) of three different NPs with three types of cells (normal and immortal cells) using effect of material on reduction in the mitochondrial metabolic activity (cell respiratory system). Results showed that AgNP/HC induced higher cell viability as compared to others and AgNP induced more mortality in fibroblast cells in a similar concentration. In the other word, highest IC50s were related to AgNP/HC and AgNP/LC in fibroblast and NB, respectively. IC50 values of AgNP in NB and STO cells were 8 ppm and IC50s of AgNP/LC in NB and STO cells were 10 ppm. Normal cells showed about twice viability as compared to immortal cells in three NPs and highest and lowest IC50 of HUVEC belonged to AgNP/LC and AgNP/HC group. AgNP/LC had higher IC50 values in normal and immortal cells ().
Figure 3. (a) Maximum half inhibitory concentration (IC50) of three different NPs with Human Caucasian neuroblastoma [BE (2)M17] (NB) and Mouse embryonic fibroblast cell line (STO) as immortal cells and HUVEC. There was significant difference between IC50 values of AgNP/HC and AgNP/LC (p< 0.05). (b) DAPI staining of HUVEC at 2, 12, and 24 ppm concentrations of AgNPs. AgNP/HC exhibited less light blue chromatin and damage to nucleus as compared to others. (c) Nucleus numbers of of HUVECs at 2, 12, and 24 ppm concentrations of AgNPs. Cells treated by AgNP/LC exhibited higher nucleus number as compared to others. At 2 ppm, the p values was 0.0001, considered extremely significant. There was not significant difference between Bare and AgNP/LC. At 12 ppm, p values was 0.0017, considered very significant. There was no significant difference between Bare and AgNP/HC.
![Figure 3. (a) Maximum half inhibitory concentration (IC50) of three different NPs with Human Caucasian neuroblastoma [BE (2)M17] (NB) and Mouse embryonic fibroblast cell line (STO) as immortal cells and HUVEC. There was significant difference between IC50 values of AgNP/HC and AgNP/LC (p< 0.05). (b) DAPI staining of HUVEC at 2, 12, and 24 ppm concentrations of AgNPs. AgNP/HC exhibited less light blue chromatin and damage to nucleus as compared to others. (c) Nucleus numbers of of HUVECs at 2, 12, and 24 ppm concentrations of AgNPs. Cells treated by AgNP/LC exhibited higher nucleus number as compared to others. At 2 ppm, the p values was 0.0001, considered extremely significant. There was not significant difference between Bare and AgNP/LC. At 12 ppm, p values was 0.0017, considered very significant. There was no significant difference between Bare and AgNP/HC.](/cms/asset/3f8b57ec-e446-4a8b-bad7-fd225b2ee171/ianb_a_1178132_f0003_c.jpg)
Observation of chromosome of nucleus
DAPI staining was carried out to evaluate fragmented nucleus via lighter blue chromatin at 2, 12, and 24 ppm concentrations of AgNP. Results from DAPI staining showed that AgNP and AgNP/LC induced apoptotic cells with lighter blue chromatin staining and AgNP/HC rarely influenced chromatin even at high concentration. 12 ppm induced higher fragmented nucleus as compared to 2 ppm in HUVEC cells treated by NPs. However, nucleus number is in accordance to MTT data and the nucleus of cells treated with AgNP/LC were more alive than others’ ().
Discussion
The aim of this study was to investigate the effect of size, zeta potential and ROS production of bare and citrate coated AgNPs on viability of normal, immortal and cancerous cells and LDH release of treated normal cells, MIC of resistant bacteria, and chromatin nucleus (Lash Citation2006).
Results of DLS measurements of zeta potential of particles showed that addition of citrate coating elevated the zeta potential (−19.26 and −21.82 mV) and resulted in more stable colloids, while bare AgNP (−7.07 mV) was more susceptible to aggregation. Citrate ions interact with silver seeds in the reaction mixture and form a complex which can slow down transformation and result in decrease of agglomeration and more stable particles as compared to poor citrate particles (Kaur and Tikoo Citation2012, Pillai and Kamat Citation2004). During the time, bare NPs with lower zeta potential may aggregate, resulting in formation of larger particles. This leads to decreasing surface area/volume and finally affects biological behavior and ROS production.
At 2 ppm concentrations, AgNP induced more ROS. This might be related to the poor coating on this NP. At higher concentrations, this difference was not significant due to high concentration of ROS. It is demonstrated that charge of NPs might influence on ROS production. Positively and neutrally charged NPs can induce more intracellular ROS production than negatively charged ones. It is even thought that positively charged NPs damage the morphology of mitochondria while negatively charged ones do not (Bhattacharjee et al. Citation2010).
LDH release data were in good agreement with results of ROS production by NPs in DPPH test. It could be said that ROS production is an important factor of cell membrane damage and increase of LDH level. LDH releases from HUVECs were higher for AgNP and AgNP/HC as compared to AgNP/LC at 28 ppm and LDH release from cells treated by AgNP/LC was lower than negative control. It is demonstrated that the key internalization route of AgNP is endocytosis and clathrine-mediated endocytosis is the preferred route for positive charged particles rather than negatively charged ones. However citrate, as a substrate for energy production in mitochondria, can internalize via specific citrate transporters. Since Ag-specific transport proteins exist in the lysosome, instability and rupture of lysosome membrane happens and results in apoptosis (García-Alonso et al. Citation2011, Mycielska et al. Citation2009). Besides highly negative charge of cell membrane there are some positively charged sites in the membrane that can facilitate internalization of negatively charged particles (Cho et al. Citation2009, Ghinea and Simionescu Citation1985). In addition to charge, size of particles plays a critical role in cell membrane disruption, too. It is demonstrated that NPs with average size between 1.2 and 22 nm can form holes in cell membrane and induce leaking of membrane and increased level of LDH release and get internalized (Wu et al. Citation2012). Earlier studies reported that for AgNPs smaller than 10 nm mode of toxicity is more related to Ag+ ion and in the larger ones particle-cell interaction is dominant (Choi and Hu Citation2008). Based on these studies, since particle size of AgNP/HC is 13.2 nm, mechanism of action could be attributed to Ag+ ions and cell membrane damage potential of Ag+ ions is higher than NPs, the induced LDH release due to smaller particles is higher than larger ones. It seems that AgNP releases more Ag+ ions than citrate coated AgNP/LC of equal size and owing to higher level of Ag+ ions released by AgNP, damage to cell membrane is higher than AgNP/LC. Ag+ ions interact with sulfhydryl groups on the cells and disrupt eukaryote and prokaryote cell membrane. Another disruptive mechanism of silver NPs is effect on ion channels and their conformational changes.
Data from MTT assay indicated that normal cells are less sensitive to the damaging potential of AgNPs as compared to immortal cells and might be used as cancer cell therapy and NPs with approximately equal zeta potential and larger size induced higher viability and bacterial inhibitory values. Also, NPs of higher negative zeta potential among particles of similar size exhibited less mortality and lower bacterial inhibitory.
Net charge of mitochondrial outer membrane is negative and NPs with lower negative charge such as AgNP can adsorb easier onto membrane than higher negative charged particles such as AgNP/LC and thus disruptive effect on mitochondria membrane decreases. Earlier studies showed that AgNPs interact with thiol groups in inner mitochondria membrane and mitochondria dysfunction is an important mechanism towards apoptosis (Heit et al. Citation2011). Since charge affinity of AgNP/LC is less than AgNP to mitochondria, interaction with thiol groups’ enzymes at the surface and inner site of mitochondria membrane decreased and cell viability more was seen using MTT assay test.
Other key factor might be Ag+ ions and it seems that level of Ag+ ion released from AgNPs is higher than coated particles because degradation rate of bare NPs is faster than coated ones and citrate could trap silver particles and result in lower free Ag+ ions to damage the cells. Additionally, data from the DPPH showed higher free radical derived from AgNP as compared to AgNP/HC that might be a satisfactory reason for difference in cell mortality and bacterial inhibitory potential of NPs.
Another reason for higher cell viability of normal cells as compared to cancerous cells treated with capped and uncapped AgNPs is that cancerous cells have different cell morphology and pore size of plasma membrane than normal cells. AgNPs can directly inhibit and activate PI3/Act, caspase nine and three signaling pathways, respectively which result in inhibition of vascular permeability, cell survival and activation of apoptosis (Vaidyanathan et al. Citation2009). All of these events make a cancerous cell more sensitive than normal cell to AgNPs, as seen in this study. This finding can be useful to design of local anticancer drug without targeting with antibodies and other ligands. It could be concluded that among particles of equal size the one with negative zeta potential showed lower cell toxicity.
Study on cell viability and bacterial inhibitory potential showed that larger NPs with equal zeta potential induced higher cell viability and bacterial inhibitory effects on normal HUVEC cells and E. coli than smaller ones, respectively. In this case, IC50 and MIC values of cells treated by AgNP/LC had the highest difference together. It is of interest to note that IC50 of normal cells treated with three AgNPs were seen in higher concentration than of MIC values, respectively. In the other words, at MIC values, the most of normal HUVEC cells remain alive. However, Ivask et al. (Citation2014) revealed that higher intracellular bioavailability of AgNPs with 10 nm in diameter than larger ones is related to cell-particle contact that resulted in higher cell toxic efficacy.
Data from DAPI staining indicated the lower effects of AgNP/HC with smaller particle size and higher negative zeta potential on chromatin nucleus. An interesting finding was that it could be observed a bit higher fragmented chromatin in cells treated with NPs as compared to control group at 2 ppm when cell viability was about 100%. This indicated that although particles at this concentration did not alter cell viability but they can affect biomolecules and use of these materials chronically will show adverse side effects even at concentration that provides 100% cell viability. Small particles have more exposed atoms on the surface and more active sites to interact with DNA. Owing to its weaker electronegativity and lower stability properties, NPs can strongly get adsorbed to DNA and bind to phosphate groups. This performance decreases with increase in the citrate concentration, and therefore zeta potential can be considered responsible for this result. In fact, when a particle is chemically stable, it does not strongly tend to interact with other materials. NPs with equal size but stronger electronegativity and higher negative zeta potential, were readily assimilated to control group and resulted in lesser influence on chromatin structure. Nuclear membrane pore (NMP) of cells has distinct internal channel diameter, some reports indicated that 25 nm particles can pass through them and enter into the nucleus and affect proteins and chromosome (Feldherr and Akin Citation1991, Liu et al. Citation2003). Every nucleus membrane has definite pore diameter. It is obvious that particles with lower particle size, even if they do not undergo aggregation, will pass through the pores and induce positive or negative effects on chromosome and other sub-cellular biomolecules in the nucleus. NMP has nuclear pore complexes (NPCs) that are close to the pore’ s wall and the pore’s center have negative and positive charges, respectively (Colwell et al. Citation2010, Serdiuk et al. Citation2012, Tagliazucchi et al. Citation2013). Owing to higher negative charge on the pore’s wall it might be speculated that negative particles (AgNP/LC and AgNP/HC) will repel from the pore’s wall as compared to weaker negative charged particle (AgNP). Even if negative charged AgNP/HC can enter into nucleus, negatively charged DNA tends to interact with positive charged materials and its influence on DNA will not be significant.
Overall, it could be suggested that variation of particle size and zeta potential affect cell organelles and biomolecules. Also, biomolecules have undergone structural and chemical changes at the concentration when the functioning of cell membrane and mitochondrial is normal. It is notable that vein endothelia cells are more resistant to the lethal concentrations of AgNPs on above mentioned cancer and immortal cells. Although, it needs more investigation to prove our assumption but the present study disclosed that eventually vein endothelial cells as a cell candidate of capillary will tolerate AgNPs with higher IC50 concentrations of immortal cells in cancer chemotherapy
Funding information
This work was supported by grant (91-04-61-18748) from Student’s Scientific Research Center, Tehran University of Medical Science, Tehran, Iran.
Disclosure statement
The authors report no conflicts of interest. The authors alone are responsible for the content and writing of this article.
References
- Bennett J, St Geme JW III. 1999. Bacterial resistance and antibiotic use in the emergency department. Pediatr Clin North Am. 46:1125–1143.
- Bhattacharjee S, de Haan LH, Evers NM, Jiang X, Marcelis AT, Zuilhof H, Rietjens IM, Alink GM. 2010. Role of surface charge and oxidative stress in cytotoxicity of organic monolayer-coated silicon nanoparticles towards macrophage NR8383 cells. Part Fibre Toxicol. 7:25.
- Cho EC, Xie J, Wurm PA, Xia Y. 2009. Understanding the role of surface charges in cellular adsorption versus internalization by selectively removing gold nanoparticles on the cell surface with a I2/KI etchant. Nano Lett. 9:1080–1084.
- Choi O, Hu Z. 2008. Size dependent and reactive oxygen species related nanosilver toxicity to nitrifying bacteria. Environ Sci Technol. 42:4583–4588.
- Clogston JD. Patri AK. 2011. Zeta potential measurement. In: McNeil SE, Ed. Characterization of Nanoparticles Intended for Drug Delivery. New York: Humana Press, pp. 63–70.
- Colwell LJ, Brenner MP, Ribbeck K. 2010. Charge as a selection criterion for translocation through the nuclear pore complex. PLoS Comput Biol. 6:e1000747.
- Eckhardt S, Brunetto PS, Gagnon J, Priebe M, Giese B, Fromm KM. 2013. Nanobio silver: its interactions with peptides and bacteria, and its uses in medicine. Chem Rev. 113:4708–4754.
- Feldherr CM, Akin D. 1991. Signal-mediated nuclear transport in proliferating and growth-arrested BALB/c 3T3 cells. J Cell Biol. 115:933–939.
- García-Alonso J, Khan FR, Misra SK, Turmaine M, Smith BD, Rainbow PS, Luoma SN, Valsami-Jones E. 2011. Cellular internalization of silver nanoparticles in gut epithelia of the estuarine polychaete Nereis diversicolor. Environ Sci Technol. 45:4630–4636.
- Gerson M. 2002. A Cancer Therapy. 6th ed. Gerson Institute. ISBN-13: 978-0961152628.
- Ghinea N, Simionescu N. 1985. Anionized and cationized hemeundecapeptides as probes for cell surface charge and permeability studies: differentiated labeling of endothelial plasmalemmal vesicles. J Cell Biol. 100:606–612.
- Gupta A, Phung LT, Taylor DE, Silver S. 2001. Diversity of silver resistance genes in IncH incompatibility group plasmids. Microbiology (Reading, Engl.). 147:3393–3402.
- Heit B, Yeung T, Grinstein S. 2011. Changes in mitochondrial surface charge mediate recruitment of signaling molecules during apoptosis. AmJ Physiol Cell Physiol. 300:C33–C41.
- Hekmat A, Saboury AA, Divsalar A. 2012. The effects of silver nanoparticles and doxorubicin combination on DNA structure and its antiproliferative effect against T47D and MCF7 cell lines. J Biomed Nanotechnol. 8:968–982.
- I Batarseh K, A Smith M. 2012. Synergistic activities of a silver (I) glutamic acid complex and reactive oxygen species (ROS): a novel antimicrobial and chemotherapeutic agent. Curr Med Chem. 19:3635–3640.
- Ivask A, Kurvet I, Kasemets K, Blinova I, Aruoja V, Suppi S, et al. 2014. Size-dependent toxicity of silver nanoparticles to bacteria, yeast, algae, crustaceans and mammalian cells in vitro. PLoS One. 9:e102108.
- Kaur J, Tikoo K. 2012. Evaluating cell specific cytotoxicity of differentially charged silver nanoparticles. Food Chem Toxicol. 51:1–14.
- Lash LH. 2006. Mitochondrial glutathione transport: physiological, pathological and toxicological implications. Chem-Biol Interact. 163:54–67.
- Liu G, Li D, Pasumarthy MK, Kowalczyk TH, Gedeon CR, Hyatt SL, et al. 2003. Nanoparticles of compacted DNA transfect postmitotic cells. J Biol Chem. 278:32578–32586.
- Ma J, Lu X, Huang Y. 2011. Genomic analysis of cytotoxicity response to nanosilver in human dermal fibroblasts. J Biomed Nanotechnol. 7:263–275.
- Mycielska ME, Patel A, Rizaner N, Mazurek MP, Keun H, Patel A, Ganapathy V, Djamgoz M. 2009. Citrate transport and metabolism in mammalian cells: prostate epithelial cells and prostate cancer. Bioessays. 31:10–20.
- Nel AE, Mädler L, Velegol D, Xia T, Hoek EM, Somasundaran P, et al. 2009. Understanding biophysicochemical interactions at the nano-bio interface. Nat Mater. 8:543–557.
- Nirmala R, Kang HS, Park HM, Navamathavan R, Jeong IS, Kim HY. 2012. Silver-loaded biomimetic hydroxyapatite grafted poly (ɛ-caprolactone) composite nanofibers: a cytotoxicity study. J Biomed Nanotechnol. 8:125–132.
- Pillai ZS, Kamat PV. 2004. What factors control the size and shape of silver nanoparticles in the citrate ion reduction method? J Phys Chem B. 108:945–951.
- Seiler M, Venkatachalam M, Cotran R. 1975. Glomerular epithelium: structural alterations induced by polycations. Science. 189:390–393.
- Serdiuk T, Alekseev S, Lysenko V, Skryshevsky V, Géloën A. 2012. Charge-driven selective localization of fluorescent nanoparticles in live cells. Nanotechnology. 23:315101.
- Sotiriou GA, Pratsinis SE. 2011. Engineering nanosilver as an antibacterial, biosensor and bioimaging material. Curr Opin Chem Eng. 1:3–10.
- Tagliazucchi M, Peleg O, Kröger M, Rabin Y, Szleifer I. 2013. Effect of charge, hydrophobicity, and sequence of nucleoporins on the translocation of model particles through the nuclear pore complex. Proc Natl Acad Sci. 110:3363–3368.
- Tavakol S. 2014. Acidic pH derived from cancer cells may induce failed reprogramming of normal differentiated cells adjacent tumor cells and turn them into cancer cells. Med Hypotheses. 83:668–672.
- Tavakol S, Nikpour MR, Hoveizi E, Tavakol B, Rezayat SM, Adabi M, Abokheili SS, Jahanshahi M. 2014. Investigating the effects of particle size and chemical structure on cytotoxicity and bacteriostatic potential of nano hydroxyapatite/chitosan/silica and nano hydroxyapatite/chitosan/silver; as antibacterial bone substitutes. J Nanoparticle Res. 16:1–13.
- Vaidyanathan R, Kalishwaralal K, Gopalram S, Gurunathan S. 2009. Nanosilver-the burgeoning therapeutic molecule and its green synthesis. Biotechnol Adv. 27:924–937.
- Wu YL, Putcha N, Ng KW, Leong DT, Lim CT, Loo SCJ, Chen X. 2012. Biophysical responses upon the interaction of nanomaterials with cellular interfaces. Acc Chem Res. 46:782–791.