Abstract
High-mobility group protein two (HMGA2), a nonhistone nuclear-binding protein and its downregulators; vimentin, matrix metallopeptidase-9 (MMP-9), and E-cadherin are shown to contribute to tumor progression and metastasis. Thus, in this study, we checked simultaneous delivery of HMGA-2 siRNA and the anticancer drug doxorubicin to enhance the anticancer treatment effects. For this purpose, we used MTT assay and real-time polymerase chain reaction (RT-PCR). Our results showed that dual delivery of Dox and HMGA-2 siRNA by trimethyl chitosan (TMC) significantly inhibited breast cancer cells growth. Additionally, the delivery of siRNA significantly silenced HMGA-2, vimentin, and MMP9 mRNAs, but led to overexpression of E-cadherin mRNA.
Keywords:
Introduction
Breast cancer accounts for a large percentage of deaths among all patients with cancer and is the most common cancer among women in Iran and all over the world (Mousavi et al. Citation2008). There are a variety of treatments for patients with breast cancer, but, nowadays, researchers are looking for a certain type of treatment to reduce the side effects of surgery and chemotherapy, called targeted therapy. In this method, we have to pinpoint the genes and proteins that are relevant to diseases and use them as therapeutic targets. They can be implemented with monoclonal antibodies, such as bevacizumab, cetuximab, and panitumumab, or by designing nanoparticles with specific antisense against specific markers. In this project, we are looking for a targeted therapy for breast cancer cells using the nanoparticles that contain siRNA (suppressed expression of HMGA2) and an anticancer drug (doxorubicin). Use of nanoparticles is a new way of designing targeted therapies that make it possible to specifically expose the drug at the right anatomical place at the right time. Chitosan (CH) nanoparticle is one of the biodegradable nanoparticles, which releases the drug in an acidic tumor environment and exposes it to target tumor cells (Jenuwein Citation2006, Kouzarides Citation2002). Some CH modifications have been shown to increase the capacity of CH as a siRNA carrier and promote the escape of the nanoparticles from the endocytic transmission. The parochial quaternization of CH (trimethylchitosan (TMC)) leads to its being soluble at a larger pH range and exhibiting an increased mucoadhesive potential (Kotzé et al. Citation1997).
Epithelial–mesenchymal transition (EMT) is a major process in tumor metastasis which is losing epithelial morphology and getting mesenchymal phenotype (Gao et al. Citation2012, Lim and Thiery Citation2012, Todosi et al. Citation2012). A major feature of EMT is the upregulation of motile mesenchymal proteins, such as vimentin, and downregulation of E-cadherin (Liu et al. Citation2012, Smith et al. Citation2013). High-mobility group protein 2 (HMGA2), a nonhistone nuclear-binding protein, is a major regulator of differentiation and cell growth and belongs to the HMGA protein family (Kumar et al. Citation2014). This protein's high flexibility has an important role in protein–protein and DNA–protein connections. One of the functions of this protein is changing chromatin structure and Promoter’s spatial, which are involved in regulating the expression of many genes. HMGA2 is associated with P120EF4 (transcription inhibiting factor), activation of cyclin A gene, and activation of E2F1 by pRB with a major role in tumorogenicity (Hammond and Sharpless Citation2008). HMGA-2 is an embryonic protein but is also selectively overexpressed in malignant cancers such as lung, breast, ovarian, hepatocellular, and malignant gliomas (Di Cello et al. Citation2008, Kumar et al. Citation2014, Mahajan et al. Citation2010, Yang et al. Citation2011). If overexpressed, HMGA-2 could lead to the epithelial–mesenchymal transition (EMT) process, tumor metastasis, and tumor progression. Additionally, HMGA2 is positively correlated with vimentin and MMP9 expressions and negatively correlated with E-cadherin expression (Xia et al. Citation2015). Vimentin expresses in myoepithelial cells, in which the expression of keratins is a sign of breast tumor cells (Anbazhagan et al. Citation1991). The expression of vimentin in invasive breast tumors is an uncommon, but frequently reported, event which is generally explained by two different hypotheses: epithelial–mesenchymal transition (EMT) and myoepithelial histogenesis (Savagner Citation2001). Vimentin-expressed carcinomas are frequently grade 3: ER-negative carcinomas with high invasion potential and treatment resistance in in vitro (Kesse-Adu and Shousha Citation2004). Matrix metalloproteinases (MMPs) are a large family of zinc-dependent proteinases that play a major role in extracellular matrix degradation and in many pathogenic processes (Leppä et al. Citation2004). Many studies have shown that an elevated expression of MMP-2, MMP-3, and MMP-9 proteins in tumor microenvironment is correlated with poor prognosis (La Rocca et al. Citation2004). MMP-9, the selected member of matrix metalloproteinases family, which is regulated by HMGA-2, profoundly influences the expression of E-cadherin (Villalta et al. Citation2014). E-cadherin protein (encoded by CDH1 gene) is needed for epithelial cell–cell adhesion and epithelial-to-mesenchymal transitions (EMT) (Perl et al. Citation1998). E-cadherin is believed to contribute to tumor suppression, since its loss or inactivation by mutations is mostly seen in invasive epithelial cell cancers and is a major stage in both tumorigenesis and progression (Meiners et al. Citation1998). Decreased cellular adhesion due to loss of E-cadherin leads to the raised invasion of tumor cells and metastases. Doxorubicin hydrochloride (Dox), a ring antibiotic anthracycline, is an antineoplastic factor used in leukemia chemotherapy. Toxic side effects, such as cardiotoxicity, alopecia, vomiting, leucopenia, and stomatitis, are the most important cause of failure of doxorubicin (Dox) chemotherapy. To achieve an efficient delivery system and reduce the side effects, Dox is normally encapsulated into drug delivery systems that selectively target-specific sections without negatively affecting the encompassing tissues (Vashist et al. Citation2011). In addition, sequential specific gene silencing by siRNA offers a significant potential as a therapeutic agent to suppress a broad range of genetic targets.
Recently, trimethyl chitosan nanoparticles have been developed and modified to encapsulate Dox and siRNA and minimize premature drug release. Trimethyl chitosan (TMC) derived from chitosan has aqueous better solubility than chitosan. TMC nanoparticles have an excellent loading capacity for proteins, oligonucleotides, drugs and a positive surface charge, suitable to attach any antibodies or mucosal adhesion (Atyabi et al. Citation2005).
Chitosan nanoparticles are widely used due to their unique properties and biocompatibility (Howard et al. Citation2006, Mao et al. Citation2010, Mittnacht et al. Citation2010). In the present study, we used the dual delivery of the key gene siRNA together with an appropriate anticancer drug to overcome cancer cells growth and progression.
Materials and methods
Oligonucleotides antisense was obtained from Santa Cruz Biotechnology (CA, Lot number: sc-37994). MDA-MB-231 cell line was obtained from Pasteur Institute of Iran (Tehran, Iran). RNA-extraction kit and real-time polymerase chain reaction (RT-PCR) master mix were obtained from Sina Gene (Tehran, Iran, Lot number: 9114054). HMGA2, E-cadherin, MMP-9, and vimentin primers were obtained from Bioneer (Daedeok-gu, Korea). Doxorubicin was obtained from Sigma-Aldrich (St. Louis, MO Lot number: 61501027).
Synthesis of TMC
First, trimethylated chitosan copolymer nanoparticles were prepared as explained in a previous study (Atyabi et al., Citation2005). Briefly, 0.5 g (MW50 kDa) chitosan was diffused in 60 mL NMP and left under stirring for 30 min; then, 1.5 g sodium azide, 5 mL sodium hydroxide (15% w/v), and 3.8 mL methyl iodide were added to the first mixture. This compound was stirred at 60 °C for 3 h. Then, 200 mL deionized water (DW) was added and stirred for 2 h. The mixture was dialyzed against DW using a dialysis tube (12 kDa) for 24 h. The mixture was precipitated by adding an excess amount of acetone. The product was washed with sodium hydroxide (15% w/v) thoroughly. The precipitate was suspended in water and dialyzed against DW for 48 h followed by freeze drying.
Preparing drug- and antisense-loaded TMC nanoparticles
The nanoparticles (NPs) were prepared following the method described in a previous study (Yin et al. Citation2009). Briefly, 10 mg TMC was dissolved in 1 mL deionized water (pH = 5.5) and incubated for 2 h at room temperature with gentle agitation. Next, 100 μL CMD (1 mg/mL), 50 μL doxorubicin (6 mg/mL) and 100 nanomol HMGA2’s antisense were mixed and slowly added to 1 mL TMC solution (10 mg/mL) and the final solution was incubated for 30 min at room temperature.
Evaluation of doxorubicin and siRNA-loading efficiency
To determine the efficiency of doxorubicin loading, Dox–TMC NPs were centrifuged (12,000 g, 20 min) and, then, the optical densities (OD) of the collected supernatant (free Dox) and primary feeding content of Dox used in the manufacturing of Dox–TMC NPs were determined at the wavelength of 480 nm by spectrophotometry (Scinco, Seoul, Korea). Briefly, efficiency of siRNA conjugation was calculated in the siRNA–TMC NPs group through centrifugation (15,000 g, 15 min) and OD absorbance of siRNA at 260 nm in accordance with the method that was used to calculate Dox-loading efficiency.
Scanning electron microscopy
Scanning with an electron microscope (SEM) (XL 30; Philips, Eindhoven, the Netherlands) was carried out to check the morphology of the generated NPs. Briefly, Dox–siRNA–TMC NPs were dried on an aluminum disk at room temperature and vacuity coated with a thin substrate of gold in an argon aerosphere. The prepared samples were evaluated by scanning electron microscope (SEM).
Fourier transform infrared spectroscopy
To check the continuity of TMC with doxorubicin, Fourier transform infrared (FTIR) spectra were analyzed by a Nicolet FT-IR spectrometer (Magna IR 550; Madison, WI) at 4 cm resolution. The sample was accumulated by the KBr pellet method and compressed to a plate to investigate the chemical reactions between TMC and doxorubicin.
Size and zeta potential measurement
The particle surface charge and sizes of the TMC and siRNA complexes were measured by dynamic light scattering using Zetasizer (Nano-ZS; Malvern Instruments, Malvern, UK). All the evaluations were applied with the wavelength of 633 nm at 25 °C and angle detection of 90 °C. Zeta potential evaluations were performed using laser Doppler electrophoresis by Zetasizer (Malvern Instruments). Each sample measurement was repeated for three times.
Assays for serum and heparin stability
To define the stability of siRNA–TMCs versus serum, 400 μL siRNA–TMC NPs was incubated with 200 μL fetal bovine serum (FBS) and gently agitated at 37 °C. At each predefined time (1, 3, 6, 9, 12, 24, 36, 48, and 72 h), 20 μL of the combination was prepared and replete at −20 °C until gel electrophoresis was obtained. Therewith, heparin-mediated instabilization of the siRNA–TMCs was attended using agarose gel electrophoresis. Also, 60 μL siRNA–TMC NPs was incubated with 0, 0.6, 1.5, and 3 μL heparin (2 μg/mL) at 37 °C for 1 h and the stability of TMC NPs was analyzed by gel electrophoresis.
In vitro release of siRNA and drug from trimethyl chitosan–CMD nanoparticles
The in vitro release was performed through the definition of the released siRNA or doxorubicin from NPs by UV-Vis spectrophotometer (U.V-1601; Shimadzu, Japan) at the wavelength of 260 nm and 480 for siRNA and Dox, respectively. Dox–TMC NPs or siRNA–TMC NPs were suspended in a newly prepared phosphate-buffered saline (PBS; pH = 7.4 and pH = 5.5, 4 mL) and redeployed into a dialysis membrane sac (mw cutoff 12 kDa; Sigma Aldrich). The NPs forming the dialysis sac were submerged in a beaker containing 50 mL PBS in the same situation as the release medium. The beaker was placed in a shaking incubator (90–100 rpm) at 37 °C. Subsequently, 2 mL of the release medium was taken out at the outline time intervals and replaced by the same medium in the same situation. Eventually, the amount of the released siRNA or doxorubicin in the release medium was analyzed by UV-Vis spectrometry. Moreover, the release medium collected from unloaded NPs was used as a blank. The siRNA or doxorubicin concentration in the release medium was calculated by comparing the UV absorbance values after the release compared with the initial total siRNA or doxorubicin, which was suspended in 50 mL PBS.
Cell growth inhibition
Cytotoxicity of the NPs forming both doxorubicin and HMGA2 siRNA was determined by computing the viability of human breast cancer cell line (MDA-MB-231) by MTT test. Cells were seeded in 96-well plates at a density of 2 × 104 and kept at 37 °C in 5% CO2. The following day, 100 μL of nanoparticle solution containing different concentrations of doxorubicin (20, 10, 5, and 2.5 μg/mL) and 100 nm HMGA2 siRNA was added. Subsequently, the cytotoxicity of TMC NPs containing both HMGA2 siRNA and doxorubicin (Dox-HMGA2 NPs) along with NPs containing only doxorubicin (doxorubicin NPs), free doxorubicin, and naked HMGA2 antisense was assessed. Cytotoxicity was measured 24 h after the treatment.
Real-time PCR
Real-time polymerase chain reaction (RT-PCR) was used to quantify gene silencing by siRNA-loaded nanoparticles. Total RNA was extracted and cDNAs were synthesized using the method described in a previously published study (Noori-Daloii et al. Citation2011). RT-PCR was performed using a SYBR Green RT-PCR mastermix (Takara Bio (Ostu, Shiga, Japan), Lot number: AF3P038). The following PCR primers were used: β-actin: (F: 5′- GGA GTC CTGTGG CAT CCA CG-3′), (R: 5′-CTA GAA GCA TTT GCG GTG GA-3′); HMGA2: (F: 5′-TGG GAG GAG CGA AAT CTA AA-3′), (R: 5-TGG TAT TCA GGT CTT TCA TGG-3); E-cadherin: (F: 5′-AGT ACA ACG ACC CAA CCC AAG-3′), (R:5′-GCA AGA ATT CCT CCA AGA ATC C-3′); vimentin: (F: 5′-CAG GCA AAG CAG GAG TCC A-3′), (R: 5′-AAG TTC TCT TCC ATT TCA CGC A-3′), and MMP9: (F: 5′-ATT TCT GCC AGG ACC GCT TCT AC-3′), and (R: 5′-ATC CGG CAA ACT GGC TCC TTC-3′). All the samples were first denatured for 5 min. at 95 °C and amplified for 45 cycles of 15 s at 95 °C and of 60 s at 60 °C. Moreover, gene-specific amplification was examined by melting curve analysis. Relative mRNA levels were specified using ΔCt method based on the relative expression of HMGA2, E-cadherin, vimentin, and MMP9 mRNA to β-actin mRNA level as a housekeeping gene.
Statistical analysis
All experiments were performed three times and the final results were the average of at least three independent experiments. Data were analyzed using analysis of variance (ANOVA) test. *p < 0.05, **p < 0.01, ***p < 0.001 were considered to be statistically significant.
Results
Characterization of nanoparticles
TMC's NP size and surface charge were assessed by dynamic light-scattering microscopy (Zetasizer Nano ZS, Malvern, Worcestershire, UK) and summarized in . The morphology of the TMC NPs was captured via SEM (.
Table 1. Size, charge, and PDI of NPs.
FTIR was used to investigate the conjugation of Dox and TMC. The FTIR spectra of Dox, TMC, and Dox-TMC are presented in . The FTIR spectrum of TMC () showed a broad band at 3420 cm−1 corresponding to the stretching vibration of NAH. The peaks at 2920 and 2840 cm−1 were typical of CAH stretch vibration, while the peaks at 1648, 1596, and 1310 cm−1 were the characteristics of amides I, II, and III, respectively. The sharp peaks at 1310 and 1010 cm−1 were assigned to the CH3 symmetrical deformation mode. The small peak at 920 cm−1 corresponded to the wagging of the saccharide structure of TMC. The FTIR spectrum for pure Dox () demonstrated multiple peaks at 3330, 2990, 1734, 1615, 1416, and 1076 cm−1. These different peaks corresponded to the different quinone and ketone carbonyls of Dox. However, it was difficult to define the different bands for quinine and ketone, because both had carbonyl groups. Comparison of the FTIR spectrum of Dox–TMC with TMC showed that there were additional absorption bands at 1714, 1122, and 810 cm−1, which corresponded to the CAOACH3 stretching bands of Dox and confirmed the successful loading of Dox on the chitosan nanoparticle carrier (). Also, Dox- and siRNA-loading efficiency were 77.4% and 80.2%, respectively.
Serum and heparin stability
The stability of siRNA–TMCs in serum was checked via the incubation of these TMCs with FBS prior to gel electrophoresis. As shown in , partial degradation and complete release of siRNA from nanoformulations was observed at 12 and 96 h, respectively ().
Figure 3. (a) Serum stability of siRNA–TMC NPs incubated in serum over a period of time (1: naked siRNA, 2:1 h, 3:3 h, 4:6 h, 5:9 h, 6:12 h, 7:24 h, 8:48 h, 9:72 hr, 10:96 h). (b) Heparin stability of siRNA–TMC NPs in various volumes of heparin (1: naked siRNA, 2: without heparin, 3:0.6 μL, 4:1.5 μL, 5:3 μL).
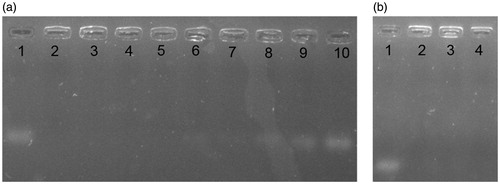
Figure 4. In vitro release profile of drug doxorubicin (A) and siRNA HMGA2 (B) from trimethyl chitosan nanoparticles in pH 7.4 and 5.5 at 37 °C.
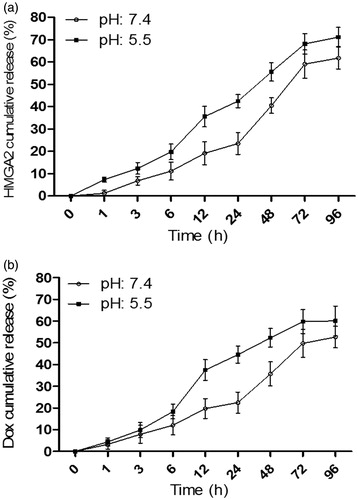
Moreover, siRNA NPs were exposed to heparin to determine the stability of nanoformulation against heparin. Our results revealed that different concentrations of heparin could not affect the degradation of nanoformulations and siRNA release ().
In vitro release studies
The drug and siRNA release of nanoparticles in buffer solutions with the two different pH values of 5.5 and 7.4 were studied at the physiological temperature of 37 °C.
The siRNA release pattern was investigated in TMC NPs and the results showed that siRNA is gradually released in up to 60 h and after 72 h reaches a steady-state phase. There was no difference in the siRNA release pattern in the PBS with pH = 7.4 and pH = 5.5 (). We found that TMC NPs release 50% of their encapsulated siRNA in the first 36 h.
The Dox release pattern from TMC NPs was investigated and our results showed that Dox release after 24 h dropped by more than half at pH 5.5 and, after 72 h, it dropped by more than half at pH 7.4 (). The Dox release at pH 5.5 was significantly greater than at pH 7.4.
Moreover, our results demonstrated that siRNA was gradually released for up to 72 h and after 96 h it reached the steady-state phase. siRNA release was further studied in different pH levels (pH 7.4 and pH 5.5). Our results showed a faster and higher siRNA release at pH 5.5 compared to pH 7.4 (). We found that TMC NPs release 50% of their encapsulated siRNA in the first 12 h.
Cytotoxicity assay
The results of the cell viability MTT assay are presented in . As shown, the growth inhibitory activity of Dox–siRNA–TMC nanoparticle was always higher than Dox–TMC NPs, siRNA–TMC NPs, free Dox and control groups. Such differences were more apparent for the higher doses of complete NPs and 96 ± 3% cell death was obtained at the highest concentration of Dox (20 μg/mL).
Analysis of gene expression in MDA-MB-231 cells
RT-PCR was used to estimate the extent of silencing. The results shown in indicate that Dox–siRNA–TMC NPs and siRNA–TMC NPs could significantly decrease (when compared with control cell values) the expression of HMGA-2, vimentin, and MMP-9 mRNA in moderate (5 μg/mL) and high (10 μg/mL) doses of Dox. Conversely, Dox–siRNA–TMC NPs and siRNA–TMC NPs could significantly increase the expression of E-cadherin in a dose-dependent manner.
Figure 6. (a) HMGA2, (b) Vimentin, (c) MMP9, (d) E-cadherin expression in MDA-MB-231 cells following treatment with different medicinal categories.
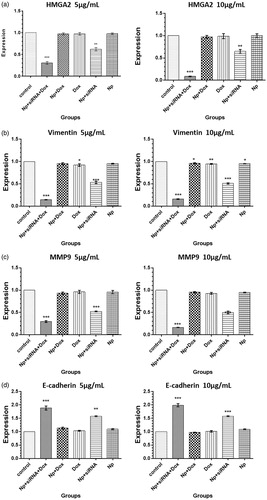
In Dox–siRNA–TMC NPs treated cells, the fold induction of HMGA-2, vimentin, and MMP-9 mRNA decreased from 1.00 to now 0.3 (±0.03), 0.15 (± 0.01), and 0.3 (± 0.04) for 5 μg/mL Dox and from 1.00 to now 0.09 (± 0.01), 0.1 (± 0.01), and 0.18 (± 0.08) for 10 μg/mL Dox, respectively (p < 0.001, p < 0.001, p < 0.001). Moreover, the mRNA expression level of E-cadherin increased from 1.00 to now 1.9 (± 0.14) and 2.0 (± 0.11) for 5 μg/mL and 10 μg/mL Dox, respectively, (p < 0.001, p < 0.001). In siRNA–TMC NPs treated cells, the mRNA level of HMGA-2, vimentin, and MMP-9 decreased from 1.00 to now 0.65 (± 0.03), 0.53 (± 0.05), and 0.51 (± 0.01), respectively, (p < 0.01, p < 0.001, p < 0.001). Conversely, the mRNA level of E-cadherin increased from 1.00 to now 1.6 (± 0.02) (p < 0.01). Furthermore, vimentin mRNA gene expression was significantly inhibited by Dox–TMC NPs and free Dox (p < 0.05, p < 0.01).
Discussion
Nowadays, different treatments are used for suppressing cancers, which include silencing oncogenes by siRNAs, anticancer drugs, radiation. But they all have some side effects. Therefore, there is a need to develop novel methods for targeted therapies that could lead to personalized medicine with fewer side effects. New advances in nanomaterials have contributed to the development of targeting strategies for elevating intratumoral drug concentrations while limiting the undesirable toxicity to healthy tissues (Kamaly et al. Citation2012). Nanoparticles are considered as “escort” molecules that deliver drug agents in tumor tissues. Partially deacetylated chitin (chitosan) and carboxymethyl derivatives have been widely used as nanovehicles due to their antitumor effects and biocompatibility (Tsukada et al. Citation1990). Ueno et al. demonstrated the efficacy of chitosan to mediate its antitumor effects by the activation of macrophages (Ueno et al. Citation2001). Moreover, chitosan nanoparticles offer a relatively safe and efficient drug delivery system and reduce premature drug release (Janes et al. Citation2001).
In the present study, we used simultaneous codelivery of the anticancer drug doxorubicin and siRNA of HMGA2 using TMC NPs. Subsequently, cell viability and gene expression of breast cancer cell line (MDA-MB-231) were evaluated using MTT assay and RT-PCR, respectively.
The first main finding of this study is Dox–siRNA–TMC NPs significantly reduce the viability of breast cancer cells in a dose-dependent manner at all concentrations except 2.5 μg/mL compared to the control cells. It seems that changes in sensitivity to Dox are caused by the silencing of HMGA-2 mRNA. Indeed, the cell viability significantly decreased when breast cancer cells were treated with HMGA-2 siRNA together with Dox. These results show the advantages of simultaneously targeting different pathways in tumors.
Several studies have already shown that dual codelivery of the key gene siRNA and appropriate anticancer drugs lead to tumor cell death; for example, Saad et al. demonstrate that an efficient codelivery of Dox and BCL2 mRNA siRNA can ultimately lead to sensitivity to chemotherapy and cell death in lung cancer cells (Saad et al. Citation2008).
In addition, using RT-PCR, we documented that HMGA-2, vimentin, and MMP-9 were significantly silenced after transfection with Dox–siRNA–TMC NPs and siRNA–TMC NPs. Interestingly, the higher Dox concentration induced greater knockdown with the highest knockdown of 90%, 83%, 82% for HMGA-2, vimentin, and MMP-9, respectively, (compared to control cells) at 10 μg/mL of Dox. Many mechanisms have been suggested to describe the transforming capability of the HMGA2 protein. For its main act of regulating gene transcription, mostly, these mechanisms are based on the capability of the HMGA2 protein to upregulate or downregulate the expression of genes that have a major role in the control of cell invasion and proliferation, like vimentin, MMP-9, and E-cadherin (Fusco and Fedele Citation2007). Vimentin has been identified as extremely significant as a canonical marker of EMT (Thiery Citation2002). EMT is determined by the expression of vimentin intracellular filaments in epithelial cells. So, pending the inverse process of EMT, known as mesenchymal–epithelial transition (MET), the cells start getting epithelial phenotype and represent a reduced vimentin expression with less motility rates (Chaffer et al. Citation2006). MMP-9 is known to play a role in tumor angiogenesis via its degradative potetntial (John and Tuszynski Citation2001, Nguyen et al. Citation2001, Stetler-Stevenson Citation1999). Degradation of extracellular matrix (ECM) can result in tumor cells and endothelial cell migration due to a lack of cell–cell and cell–matrix connectivity.
Another interesting finding of our study was that downregulation of HMGA-2 led to overexpression of E-cadherin with the highest upregulation at 10 μg/mL of Dox. Cell–cell adhesion mediated by E-cadherin has been found to decrease in many cancers. More recently, it has been shown that lack of E-cadherin causes the necessary signals that actively enforce tumor-cell metastasis and invasion (Bullions and Levine Citation1998, Christofori and Semb Citation1999).
In conclusion, our results demonstrated that Dox and HMGA-2 were successfully encapsulated in TMC NPs and had a great potential for HMGA-2 and its downstream-signaling molecules silencing. Moreover, the HMGA-2 mRNA gene silencing in MDA-MB-231 was closely related to the drug sensitivity to Dox and led to a significant cytotoxic effect. The results of the present study also confirm that Dox–siRNA–TMC NPs are always more efficient in inducing tumor cell death and HMGA-2 gene silencing.
Acknowledgement
This work was financially supported by Iran National Science Fundation (INSF).
Disclosure statement
The authors report no conflicts of interest. The authors alone are responsible for the content and writing of this article.
References
- Anbazhagan R, Bartek J, Monaghan P, Gusterson B. 1991. Growth and development of the human infant breast. Am J Anat. 192:407–417.
- Atyabi F, Majzoob S, Iman M, Salehi M, Dorkoosh F. 2005. In vitro evaluation and modification of pectinate gel beads containing trimethyl chitosan, as a multi-particulate system for delivery of water-soluble macromolecules to colon. Carbohyd Polym. 61:39–51.
- Bullions LC, Levine AJ. 1998. The role of beta-catenin in cell adhesion, signal transduction, and cancer. Curr Opin Oncol. 10:81–87.
- Chaffer CL, Brennan JP, Slavin JL, Blick T, Thompson EW, Williams ED. 2006. Mesenchymal-to-epithelial transition facilitates bladder cancer metastasis: role of fibroblast growth factor receptor-2. Cancer Res. 66:11271–11278.
- Christofori G, Semb H. 1999. The role of the cell-adhesion molecule E-cadherin as a tumour-suppressor gene. Trends Biochem Sci. 24:73–76.
- Di Cello F, Hillion J, Hristov A, Wood LJ, Mukherjee M, Schuldenfrei A, et al. 2008. HMGA2 participates in transformation in human lung cancer. Mol. Cancer Res. 6:743–750.
- Fusco A, Fedele M. 2007. Roles of HMGA proteins in cancer. Nat Rev Cancer. 7:899–910.
- Gao D, Vahdat LT, Wong S, Chang JC, Mittal V. 2012. Microenvironmental regulation of epithelial–mesenchymal transitions in cancer. Cancer Res. 72:4883–4889.
- Hammond SM, Sharpless NE. 2008. HMGA2, microRNAs, and stem cell aging. Cell. 135:1013–1016.
- Howard KA, Rahbek UL, Liu X, Damgaard CK, Glud SZ, Andersen MØ, et al. 2006. RNA interference in vitro and in vivo using a chitosan/siRNA nanoparticle system. Mol Ther. 14:476–484.
- Janes K, Calvo P, Alonso M. 2001. Polysaccharide colloidal particles as delivery systems for macromolecules. Adv Drug Deliv Rev. 47:83–97.
- Jenuwein T. 2006. The epigenetic magic of histone lysine methylation. FEBS J. 273:3121–3135.
- John A, Tuszynski G. 2001. The role of matrix metalloproteinases in tumor angiogenesis and tumor metastasis. Pathol Oncol Res. 7:14–23.
- Kamaly N, Xiao Z, Valencia PM, Radovic-Moreno AF, Farokhzad OC. 2012. Targeted polymeric therapeutic nanoparticles: design, development and clinical translation. Chem Soc Rev. 41:2971–3010.
- Kesse-Adu R, Shousha S. 2004. Myoepithelial markers are expressed in at least 29% of oestrogen receptor negative invasive breast carcinoma. Mod Pathol. 17:646–652.
- Kotzé AR, Lueβen HL, De Leeuw BJ, Verhoef JC, Junginger HE. 1997. N-trimethyl chitosan chloride as a potential absorption enhancer across mucosal surfaces: in vitro evaluation in intestinal epithelial cells (Caco-2). Pharm Res. 14:1197–1202.
- Kouzarides T. 2002. Histone methylation in transcriptional control. Curr Opin Genet Dev. 12:198–209.
- Kumar MS, Armenteros-Monterroso E, East P, Chakravorty P, Matthews N, Winslow MM, Downward J. 2014. HMGA2 functions as a competing endogenous RNA to promote lung cancer progression. Nature. 505:212–217.
- La Rocca G, Pucci-Minafra I, Marrazzo A, Taormina P, Minafra S. 2004. Zymographic detection and clinical correlations of MMP-2 and MMP-9 in breast cancer sera. Br J Cancer. 90:1414–1421.
- Leppä S, Saarto T, Vehmanen L, Blomqvist C, Elomaa I. 2004. A high serum matrix metalloproteinase-2 level is associated with an adverse prognosis in node-positive breast carcinoma. Clin Cancer Res. 10:1057–1063.
- Lim J, Thiery JP. 2012. Epithelial-mesenchymal transitions: insights from development. Development. 139:3471–3486.
- Liu AN, Zhu ZH, Chang SJ, Hang XS. 2012. Twist expression associated with the epithelial-mesenchymal transition in gastric cancer. Mol Cell Biochem. 367:195–203.
- Mahajan A, Liu Z, Gellert L, Zou X, Yang G, Lee P, Yang X, Wei JJ. 2010. HMGA2: a biomarker significantly overexpressed in high-grade ovarian serous carcinoma. Mod Pathol. 23:673–681.
- Mao S, Sun W, Kissel T. 2010. Chitosan-based formulations for delivery of DNA and siRNA. Adv Drug Deliv Rev. 62:12–27.
- Meiners S, Brinkmann V, Naundorf H, Birchmeier W. 1998. Role of morphogenetic factors in metastasis of mammary carcinoma cells. Oncogene. 16:9–20.
- Mittnacht U, Hartmann H, Hein S, Oliveira H, Dong MP, Peêgo A, et al. 2010. Chitosan/siRNA nanoparticles biofunctionalize nerve implants and enable neurite outgrowth. Nano Lett. 10:3933–3939.
- Mousavi S, Alamolhoda A, Gouya M, Lickiss N. 2008. Implementation of comprehensive national cancer control program in Iran: an experience in a developing country. Ann Oncol. 19:398–400.
- Nguyen M, Arkell J, Jackson CJ. 2001. Human endothelial gelatinases and angiogenesis. Int J Biochem Cell Biol. 33:960–970.
- Noori-Daloii MR, Momeny M, Yousefi M, Shirazi FG, Yaseri M, Motamed N, Kazemialiakbar N, Hashemi S. 2011. Multifaceted preventive effects of single agent quercetin on a human prostate adenocarcinoma cell line (PC-3): implications for nutritional transcriptomics and multi-target therapy. Med Oncol. 28:1395–1404.
- Perl AK, Wilgenbus P, Dahl U, Semb H, Christofori G. 1998. A causal role for E-cadherin in the transition from adenoma to carcinoma. Nature. 392:190–193.
- Saad M, Garbuzenko O, Minko T. 2008. Co-delivery of siRNA and an anticancer drug for treatment of multidrug-resistant cancer. Nanomedicine (London, England). 3:761–76
- Savagner P. 2001. Leaving the neighborhood: molecular mechanisms involved during epithelial‐mesenchymal transition. Bioessays. 23:912–923.
- Smith A, Teknos TN, Pan Q. 2013. Epithelial to mesenchymal transition in head and neck squamous cell carcinoma. Oral Oncol. 49:287–292.
- Stetler-Stevenson WG. 1999. Matrix metalloproteinases in angiogenesis: a moving target for therapeutic intervention. J Clin Invest. 103:1237.
- Thiery JP. 2002. Epithelial-mesenchymal transitions in tumour progression. Nat Rev Cancer. 2:442–454.
- Todosi AM, Gavrilescu MM, Aniţei GM, Filip B, Scripcariu V. 2012. Colon cancer at molecular level-usefulness of epithelial-mesenchymal transition analysis.
- Tsukada K, Matsumoto T, Aizawa K, Tokoro A, Naruse RS, Suzuki S, Suzuki M. 1990. Antimetastatic and growth-inhibitory effects of N-acetylchitohexaose in mice bearing Lewis lung carcinoma. Jpn J Cancer Res. 81:259–265.
- Ueno H, Murakami M, Okumura M, Kadosawa T, Uede T, Fujinaga T. 2001. Chitosan accelerates the production of osteopontin from polymorphonuclear leukocytes. Biomaterials. 22:1667–1673.
- Vashist SK, Zheng D, Pastorin G, Al-Rubeaan K, Luong JH, Sheu FS. 2011. Delivery of drugs and biomolecules using carbon nanotubes. Carbon. 49:4077–4097.
- Villalta PC, Rocic P, Townsley MI. 2014. Role of MMP2 and MMP9 in TRPV4-induced lung injury. Am J Physiol Lung Cell Mol Physiol. 307:L652–L659.
- Xia YY, Yin L, Tian H, Guo WJ, Jiang N, Jiang XS, et al. 2015. hMga2 is associated with epithelial–mesenchymal transition and can predict poor prognosis in nasopharyngeal carcinoma. Onco Targets Ther. 8:169.
- Yang G, Zhang L, Bo J, Hou K, Cai X, Chen Y, et al. 2011. Overexpression of HMGA2 in bladder cancer and its association with clinicopathologic features and prognosis: HMGA2 as a prognostic marker of bladder cancer. Eur J Surg Oncol. 37:265–271.
- Yin L, Ding J, He C, Cui L, Tang C, Yin C. 2009. Drug permeability and mucoadhesion properties of thiolated trimethyl chitosan nanoparticles in oral insulin delivery. Biomaterials. 30:5691–5700.