Abstract
In this study we report the development and optimization of poly (D, L-lactide-co-glycolide) (PLGA) polymer encapsulated poorly aqueous soluble nonsteroidal antiandrogen drug bicalutamide, to develop a sustained release formulation for the treatment of prostate cancer. The bicalutamide-loaded PLGA nanoparticles were prepared by single emulsion (O/W) solvent evaporation method, and different process parameters like polymer concentration in the organic phase, surfactant concentration in aqueous phase and centrifugation speed for separation of nanoparticles were evaluated to optimize the drug-loaded nanoparticles. The optimum formulation of bicalutamide-loaded PLGA nanoparticles characterized extensively by different analytical techniques like laser light scattering to determine average particle size and size distribution, scanning electron microscopy (SEM) for surface morphology, powder X-ray diffraction (PXRD) for surface chemistry and differential scanning calorimetry (DSC) for thermogram properties. Significant decrease of crystallinity of bicalutamide confirms entrapment of the drug within the PLGA polymer matrix. Further, the drug encapsulation efficiency (EE) and in vitro drug release profile were measured by high-performance liquid chromatography and UV-spectrophotometry. In vitro drug release exhibited biphasic pattern with initial burst release followed by slow and continuous release up to 5 days. Optimum formulation of bicalutamide-loaded PLGA nanoparticles shows significant anti-tumor activity over prostate cancer cell lines (DU 145). The newly developed optimum formulation nanoparticles could be useful for sustained release delivery of bicalutamide.
Introduction
Prostate cancer is one of the most common cancers in men in more developed and developing countries and increases day-to-day. Majority of the middle age people are diagnosed with this neoplasm. The early stage of neoplasm are often asymptomatic and most of the cases they are not detected until the advanced stages. Further, survival rate after diagnosis is also poor, almost around 32%. A handful numbers of chemotherapeutic agents are currently recommended for the treatment of prostate cancer. However, poor aqueous solubility and toxicity due to lack of target specificity are the major hindrance for delivery of the chemotherapeutic agents. Current day development of new formulation using hydrophobic drug is a major challenge faced by the pharmaceuticals (Bolourchian et al. Citation2013). Most of the active molecules already developed or in the developmental stage for the treatment of prostate cancer are poorly aqueous soluble (Saeedi et al. Citation2011). This can lead to issues such as low bioavailability, erratic absorption profiles and reduced patient compliance from having to administer larger or more frequent doses (Aulton Citation1988). Several approaches were attempted to overcome the solubility problem of these poorly aqueous soluble drugs, such as the use of surfactants, inclusion complexation, use of polymorph and amorphous form of drug micronization or solid dispersion techniques, micro emulsion or liposomes (Lawrence and Rees Citation2000, Nakano Citation2000, Schwendener and Schott Citation1996, Vasconcelos et al. Citation2007). For the past few decades, various polymers have been used in the formulation of nanoparticles for drug delivery research to increase the therapeutic benefit, while minimizing the side effects and to improve the pharmacokinetic properties of anticancer drugs (Adams et al. Citation2003, Hoskins et al. Citation2012, Qiu and Bae Citation2006, Svenson Citation2009). Recently, bicalutamide, a popular choice of orally active, nonsteroidal antiandrogen recommended as single agent for the treatment of prostate cancer, is available in different brands in market (Masiello et al. Citation2002). But, the major hindrance for delivery of bicalutamide by oral route is the poor bioavailability because of the limited absorption of drug into the circulation through the intestinal epithelium due to its low aqueous solubility and polymorphism; hence the drug has been classified under BCS class II as per biopharmaceutical classification (Veiga et al. Citation1996). Recently, researchers focus several techniques to develop a successful formulation using bicalutamide for the treatment of prostate cancer in the form of solid dosage forms (Li et al. Citation2011). But, the current regimen of chemotherapy is far from satisfactory. Contrary to the above, our main objective of present work is to design and develop a new formulation by encapsulation of bicalutamide in novel biodegradable and biocompatible poly (lactic-co-glycolic acid) (PLGA) polymeric vehicle for delivery in parenteral route, can be a foot ahead toward controlling the release of drug, targeting the drug to a tissue with extended clinical effects and reduced dosing frequency there by overcoming the side effects. In our current study, PLGA-bicalutamide nanoparticles prepared by single emulsion (O/W) solvent evaporation techniques, and several process parameters such as polymer concentration in organic phase, surfactant concentration in aqueous phase, and centrifugation force for separation of nanoparticles to receive maximum yield were evaluated; and the optimum formulation was characterized by different analytical techniques such as powder X-ray diffraction (PXRD), thermogravimetric analysis (TGA), Fourier-transform infrared spectroscopy (FT-IR), and scanning electron microscopy (SEM). Drug release study of optimum formulation was performed in phosphate buffer saline (pH 7.4) and analyzed by chromatographic method, confirming successful development sustained release formulation of bicalutamide nanoparticles and release mechanism could be related to the degradation of the polymer. In vitro anticancer activities of optimized nanoparticles were valuated using DU-145 (prostate cancer cell line) cell line that exhibited significant result.
Materials and methods
Materials
Bicalutamide (molecular weight, 430.373 g/mol) was obtained as a gift sample from M/s Cipla Pvt Ltd., Goa, Maharashtra, India. The polymer used in this work, poly (D, L–lactide-coglycolide) (PLGA), with a copolymer ratio of D, L-lactide to glycolide of 50:50 (molecular weight in the range, 24,000–38,000 g/mol purchased from M/s Boehringer Ingelheim Pharma Gmbh & Co., Germany. The nonionic emulsifier poly vinyl alcohol (PVA, average molecular weight, 30,000–70,000) was purchased from M/s Sigma-Aldrich Corporation, St. Louis, MO. The solvent acetone and other chemicals in AR grade were purchased from M/S Sigma Aldrich, St. Louis, MO. Deionized ultrapure water (Milli-Q Millipore Corporation, Billerica, MA, specific resistivity 18.2 MΩ) was used in all the syntheses explained earlier and the chemicals utilized in this study were used as received without further purification.
Preparation of PLGA-bicalutamide nanoparticles by(O/W) single emulsion method
In the present study, initially we developed several numbers of different formulations by varying different manufacturing parameters and only the optimum formulation have been reported here on the basis of physiochemical evaluation of the same. In the present method, PLGA-containing bicalutamide was prepared using oil in water (O/W) single emulsion and solvent emulsification–evaporation method (Jeffery et al. Citation1991). The method in brief, weighed amount of PLGA polymer and bicalutamide active drug () were dissolved in 5 ml of acetone, and then added drop by drop using a 16-gauge needle to 15 ml of PVA aqueous solution, whereas concentration of PVA was varied from 0.5% to 5% (). The emulsion formed was homogenized at specific optimization homogenization speed (14,000) using a high speed homogenizer (T-25 ULTRA-TURRAX- IKA®, Germany) for 5 min. The resulting O/W emulsion formed was stirred in a magnetic stirrer (RH digital white, IKA, Germany) for a period of 8 h for complete evaporation of the solvent. The resulting suspension of nanoparticles were centrifuged (Neofuge 15R, Heal force, China) for 10 min and washed several times with deionized water to remove the excess surfactant (PVA). Finally, the particles were resuspended in a cryoprotectant (0.5% w/v mannitol solution) and freeze dried at −82 °C for 12 h with a vacuum pressure of 20 Pa (Freezers India, New Delhi).
Table 1. Polymer–drug composition of nanoparticles.
Table 2. Effect of PVA concentration (%) in aqueous phase over mean diameter of particle in different formulations where drug and polymer concentration ratio are constant.
Percentage of yield
The lyophilized formulation from each batch was weighed and the respective percentage yield was calculated using the following formula (Sun et al. Citation2015).
Percentage yield = Amount (mg) of lyophilized nanoparticles obtained ×100/Total amount of PLGA and bicalutamide used to prepare nanoparticles (mg).
Determination of drug content
From each batch of above said formulations, 10 mg of freeze-dried PLGA-encapsulated bicalutamide nanoparticles were dispersed separately in 2 ml of acetone and was kept for 1–2 h in an incubator shaker at 37 °C. Drug from nanoparticles was extracted in phosphate buffer saline of pH 7.4. The extracts were centrifuged at 3315 × g rcf (∼5000 rpm) and filtered through membrane filter (0.22 μm) (Maji et al. Citation2014). Finally, 5 ml of supernatant was taken using micropipette (eppendrof, United States of America) and absorbance was measured at 270 nm using a spectrophotometer (Swamivelmanickam et al. Citation2009). The drug entrapment efficiency was determined from the calibration curve prepared earlier using the following formula (Maji et al. Citation2014).
All experiments were carried out in triplicate and the error was expressed as SD.
Determination of PVA Content
The amount of PVA present in bicalutamide-loaded PLGA nanoparticles was determined by colorimetric method. The method is based on the formation of a colored complex between two adjacent hydroxyl groups of PVA and an iodine molecule (Lee et al. Citation1999). Briefly, 5 mg of lyophilized drug-loaded PLGA nanoparticles from each batch was treated with 5 ml of 0.5M of NaOH solution for 10 minutes at 60° C. The above said solution was then neutralized with 1 ml of 1N HCl and volume was adjusted to 10 ml with distilled water. Further, 5 ml of 0.65 M solution of boric acid, 2 ml of 0.05 M of KI solution, and distilled water were added to this solution after neutralization. Finally, the absorbance of the resulting solution was measured at 690 nm after incubating for 20 min. A standard calibration plot was also prepared under same conditions.
Determination of hydrophobicity of bicalutamide-loaded nanoparticles
The surface hydrophobicity of different batch of bicalutamide-loaded PLGA polymeric nanoparticles prepared by varied ranges of surfactant (PVA) concentrations as shown in were measured using Rose Bengal dye (Müller Citation1991). In this method, drug-loaded nanoparticles of all formulations were incubated with different concentrations of Rose Bengal dye for 5 h at room temperature. The samples were centrifuged at 15,000 × g rcf for 20 min to spin down the nanoparticles. Finally, the supernatant of each sample was analyzed spectrophotometrically at 540 nm to determine the unbound dye, which might bind to centrifuge tubes. The dye solution without polymeric nanoparticles was run as a control under similar conditions to determine the dye bound to centrifuge tubes. The binding constant was calculated using Scatchard plot according to the equation:
where, r is the amount of Rose Bengal absorbed per milligrams of nanoparticles (μg/ml); a is equilibrium concentration of Rose Bengal (μg/ml); K is the binding constant (ml/μg); and N is the maximum amount bound (μg/mg).
In vitro drug release study
The in vitro drug release study was carried out in phosphate buffer saline solution (PBS) of pH 7.4 with some modification in dissolution process as described in literature (Herrero-Vanrell et al. Citation2000). The optimized formulation was the only one chosen for the in vitro drug release study. The method in brief is as follows: optimized (PLGA-BCL-5E-LS) batch of 100 mg of freeze-dried and cryoprotected bicalutamide-loaded PLGA nanoparticles were placed in a screw capped glass vials and suspended in 5 ml of isotonic phosphate buffer saline (PBS) solution of pH 7.4 containing 1% of polysorbate 80. The glass vials were then placed in a mechanical shaking bath (100 cycles/min), at a temperature adjusted to 37 °C. One milliliter of sample was removed at predetermined time intervals and replaced with an equal quantity of fresh PBS. The samples were then ultracentrifuged at 15,778 × g rcf (∼12,000 rpm) for 20 min and appropriately diluted. Finally, it was filtered and quantitatively analyzed using a UV spectrophotometer (UV-2600, Shimadzu, Japan) at 270 nm (Clarke Citation1994, Hsu and Chien Citation1994). Calibration curve of pure bicalutamide in PBS was plotted in concentration range of 5–100 mcg/ml.
Cell culture
The human prostate cells (DU 145) were obtained from ATCC (Rockville, MD), were cultured in Dulbecco’s Modified Eagle’s Medium (DMEM) supplemented with 10% fetal bovine serum (FBS) and 1% penicillin-streptomycin. DU 145 cells were cultivated in a 5% CO2-humidified atmosphere at 37 °C. The cells were subcultured using trypsin–EDTA when they were 85–90% confluent. All experiments were performed with the cells when they were within four passages after revival from cryopreservation.
Determination of IC50 of bicalutamide on DU 145 prostate cancer cell line
To determine IC50 value of active drug bicalutamide, five different (10–100 μg/ml) concentrations of drug solution were prepared from a stock solution of bicalutamide (1 mg/ml) in dimethyl sulfoxide (DMSO, GR grade, Merck, India). At first, 5 × 103 DU 145 cells/ml per well were placed in a 96-well plate (McGowen et al. Citation2000, Okamoto et al. Citation2012). The cells were then incubated at 37 °C in the CO2 incubator (Thermo Scientific™, Thermo Fisher Scientific, Waltham, MA) for 24 h to allow sufficient cell adhesion, and the incubation was continued for a total duration of 72 h after addition of bicalutamide to the extent 10, 20, 40, 80, and 100 μg/ml (in triplicate). Cultured DU 145 cells (5 × 103cells/ml) in DMEM medium was taken as control. After 72 h, the MTT assay was performed by adding 10 μl 3–(4, 5-dimethylthiazole-2-yl)-2, 5-phenyltetrazolium bromide (MTT) reagent at 1 mg/ml (Sigma-Aldrich, St. Louis, MO), in 1:9 ratio (MTT reagent:DMEM medium) to all the wells with subsequent incubation in dark for a period of 4 h at 37 °C. The reaction was stopped by adding 200 μl DMSO to each well of the plate after removal of the MTT and DMEM medium. The absorbance was then measured at 550 nm in an ELISA reader (iMark™ Microplate Absorbance Reader, Bio-Rad, Hercules, CA), the dose response curves were generated and the DU 145 cell sensitivity to bicalutamide was expressed as IC50.
In vitro cell inhibitory assay of optimized formulation bicalutamide-loaded PLGA nanoparticles (PLGA-BCL-5ALS) over human prostate cancer cell lines
Cytotoxicity study of optimized formulation of the bicalutamide-loaded PLGA nanoparticles was evaluated by MTT assay. DU 145 (prostate cancer cells) cells were seeded at a density of 5 × 103 cells per well into 96-well plates with their specific cell culture medium (Schleich et al. Citation2013). The cells were incubated at 37 °C in humidified atmosphere containing 5% CO2 for 24 h. The medium was then replaced with fresh medium, and the drug-loaded optimized nanopaticles formulations (PLGA-BCL-5E-LS) containing 40 μg of bicalutamide were added and incubated with the cells for 24 , 48 and 72 h in above mentioned condition. After each time point the plate was removed from the incubator and the medium was removed; each well was rinsed with PBS; and 20 μl of MTT solution was added followed by incubation for 4 h. Then, the metabolized product MTT formazan was dissolved by adding 200 μl of DMSO to each well. Finally, the plate was shaken for 2 min, and the absorbance of the formazan product was measured at 550 nm in a microplate reader (iMark™ Microplate Absorbance Reader, Bio-Rad, Hercules, CA).
In-vitro cellular uptake study of bicalutamide-loaded PLGA nanoparticles (PLGA-BCL-5E-LS) over human prostate cancer cell lines
In-vitro cellular uptake study of optimized formulation of nanoparticles was performed in human prostate cancer cell line (DU 145) (Sahoo et al. Citation2002). The method in brief, six well plates were seeded with 5 × 104 cells per well density and incubated at 37 °C in humidified atmosphere containing 5% CO2 for 24 h to attach the cells. After 24 h the medium of each plate was replaced with 1 ml of fresh medium containing optimized formulation PLGA-BCL-5E-LS (100 μg/ml of bicalutamide) and incubated further in the same condition for 30 min (0.5 h), 1 and 2 h. At each time points, the cells were then washed with PBS to remove the nanoparticles that were not internalized. The cells were then lysed by incubating them with 0.5 ml of RIPA lysis buffer system (RIPA Buffer, Product Number R0278, St. Louis, MO) for 10 min at 4 °C in ice-cold water. A 500 μl of each cell lysate aliquot was used immediately for the cell protein determination and the remaining portion was preserved in nitrogen at −70 °C for further use. Carefully, the lysate was centrifuged at 7168 × g rcf for 5 min at 4 °C to pellet the cell debris. The supernatant was collected and centrifuged further at 8092 × g rcf for 10 min in a micro centrifuge to remove unwanted cell debris. The supernatant was then analyzed for bicalutamide using HPLC method. A standard plot with different concentration of nanoparticles was constructed simultaneously under similar conditions to determine the amount of nanoparticles in cell lysates. The data were normalized to per milligram of cell protein. In order to determine whether the uptake mechanism of optimized nanoparticles entry into cells (DU145) was mediated by endocytosis, we again performed the cellular uptake study at a temperature of 4 °C. Several literature reported that an uptake mechanism of nanoparticles by endocytotic mechanism is driven by an energy-dependent, temperature-sensitive process. So, in order to prevent this internalization mechanism, the cells were incubated at 4 °C and treated with optimized formulation at the same condition (Mo and Lim 2004). To perform the experiment, 5 × 104 cells were pre-incubated in DMEM at 4° C and after 30 min the medium of each plate was replaced with 1 ml of fresh medium containing optimized formulation PLGA-BCL-5E-LS (100 μg/ml of bicalutamide) and incubated further in the same (4 °C) condition for 30 min, 1 and 2 h. Uptake was terminated with washing the cells thrice with ice-cold phosphate buffer saline and cells were then lysed by incubating it with 0.5 ml of RIPA lysis buffer system (RIPA Buffer, Product Number R0278, St. Louis, MO) for 5 min at 4 °C in ice-cold water and the amount of bicalutamide internalized was measured using the method described earlier. A calibration plot with different concentration of nanoparticles was constructed simultaneously under similar conditions (4° C) to determine the amount of nanoparticles in cell lysates.
Stability study
Following the ICH guidelines, optimized formulation (PLGA-BCL-5E-LS) containing bicalutamide were stored at elevated temperatures and relative humidity (25 ± 2 °C/60% RH, 45 ± 2 °C/75% RH, 60 ± 2 °C/75% RH) in a stability analysis chamber (Darwin Chambers Company, St Louis, MO) over a period of 3 months. Samples stored at 2–8 °C were used as control. Samples were then kept for 3 months for stability analysis and after 3 months drug content studies of optimized formulation of the nanoparticles were compared with those of the control formulations (Jain et al. Citation2010).
Characterization
PXRD analysis of PLGA, active pharmaceutical ingredients bicalutamide and PLGA-encapsulated bicalutamide nanopaticles formulation (PLGA-BCL-5E-LS) were performed using X'Pert Pro MPD diffractometer (Panalytical, Almelo, The Netherlands) with Cu Kα1 (λ = 1.5418 Å) radiation at 40 mA, 40 kV. PLGA, bicalutamide and drug-loaded nanoparticles formulations were scanned in the 2θ range of 5–60°, with 0.03° step size and count time of 2s at each point. Particle morphology and size of bicalutamide-loaded PLGA nanoparticles were analyzed using Carl Zeiss SMT AG SUPRA® 40 VP (a Carl Zeiss SMT AG Company, Oberkochen, Germany) field emission scanning electron microscope (FE-SEM). FTIR spectra of the prepared powders were recorded at room temperature using the KBr (Sigma-Aldrich, St. Louis, MO, ≥99%) pellet method (sample: KBr =1:100) on a Perkin–Elmer Spectrum 100 spectrophotometer (PerkinElmer Inc., Waltham, MA) at 400–4000 cm−1 range with average of 50 scans to evaluate the encapsulation of the drug in polymeric nanostructure of PLGA. Differential scanning calorimetry (DSC) study was performed with Polymer PLGA, pure drug bicalutamide, and drug-loaded nanoparticles samples, were separately sealed in aluminum cells and set at temperature from 30 °C to 500 °C, and thermal analysis was performed at a heating rate maintained at 10 °C per minute in a nitrogen atmosphere.
Figure 1. FTIR spectroscopy of (a) optimized formulation PLGA-BCL-5E-LS (b) Bicalutamide, and (c) PLGA.
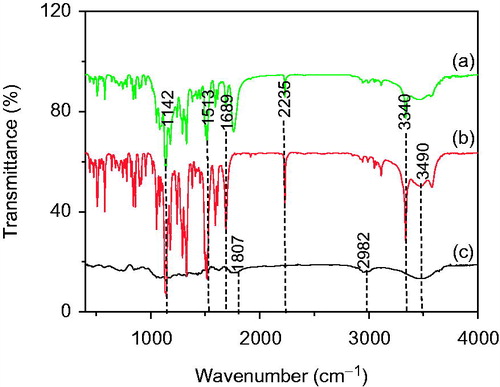
Figure 2. Powder X-ray diffraction patterns of (a) Bicalutamide (b) PLGA and (c) PLGA-BCL-5E-LS nanoparticles powders.
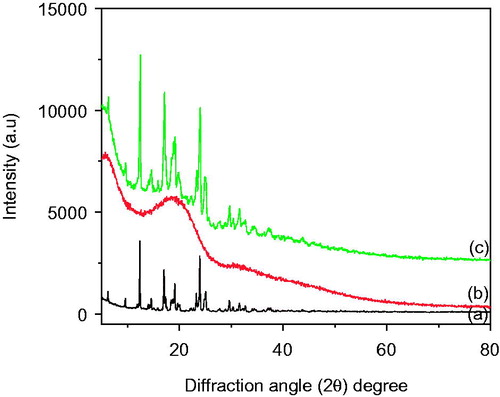
Results and discussions
In the present study, the simple lab-based techniques, such as single emulsion-solvent evaporation method, was used for formulating PLGA-encapsulated bicalutamide nanoparticles with homogeneous uniform size distribution. At the time of synthesis of bicalutamide-loaded PLGA nanoparticles some of the process parameters such as polymer content in the formulation, surfactant concentration in continuous phase, and centrifugation speed for separation to get maximum yield has been evaluated in order to achieve optimal formulation with our desired requisite. Only one parameter was changed in each series of experiments whereas other was constant. In our study we prepared several formulations by single emulsion techniques, and PLGA concentrations on the size of the obtained nanoparticles were studied. In order to study the influence the polymer (PLGA) content on nanoparticles size, PLGA was varied from 100 to 500 mg (20 mg/ml–100 mg/ml of acetone) where amount of bicalutamide (drug) was fixed (100 mg). The concentration of polymer in organic phase might affect the size of the formed nanoparticles. The results listed in clearly exhibit that when the concentration of polymer increased in the acetone solution from 20 to 100 mg/ml, the particle size increased from 127 to 289 nm. These results are in agreement with the previous work reported by Budhina et al. This may be attributed that a low PLGA concentration resulted in a low viscosity of the polymer solution which in turn resulted in smaller emulsion droplets in the aqueous phase. In turn, increase in the PLGA content in the internal phase caused an increase in viscosity. The high viscosity of the internal phase might resist against the shear forces of high speed homogenizer during emulsification. Hence, the formation of small droplets was difficult and only a coarse emulsion was formed leading to bigger particles during the solvent elimination process (Budhian et al. Citation2005, Mainardes and Evangelista Citation2005). PVA is a water-soluble synthetic polymer and a macromolecular emulsifier widely used for the fabrication of polymeric nanoparticles. PVA increases the viscosity of the aqueous phase and have the capability to adsorb onto the water–oil droplet interface; hence, it is a good emulsifying agent and stabilizes emulsion droplets. In our present study, we tried to evaluate the effect of PVA concentration in external aqueous phase over particle size of drug-loaded nanoparticles. The O/W emulsion was prepared with a varied concentration PVA (%) in aqueous phase from 0.5 to 5% where the speed of homogenization fixed at 14 000 rpm. The results in show that there was a decrease in particle size from 387 to 229 nm when the PVA concentration in the external aqueous phase was increased from 0.5 to 5% (w/v). It can be explained that a higher concentration PVA yielded a higher viscosity of the solution. This viscous solution could better stabilize the emulsion droplet against coalescence, resulting in a smaller particle size (Yang et al. Citation2001). Earlier, Budhina et al. also reported that lower concentration of surfactant was unable to stabilize all the nanoparticles and thus some of them would tend to aggregate (Budhian et al. Citation2005). As a result, nanoparticles with larger size would be produced. The larger amount of surfactant plays an important role in the emulsification process to stabilize the emulsion droplets by preventing coalescence of globules. Hence, smaller particle was formed. In order to obtain better yield percentage and with maximum encapsulation efficiency (EE), centrifugation speed for separation of nanoparticles after the abovementioned process for different batches were varied with different centrifugation forces of 5500, 9500 and in 12,000 rpm (3315 × g, 9891 × g, 15,778 × g), respectively (). It was found that yields were more in case of the formulations PLGA-BCL-3ALS, PLGA-BCL-4ALS, and PLGA-BCL-5ALS exposed to lower speed of centrifugation as compared to the other experimental formulations separated high speed of centrifugation. These results are in agreement with the previous work reported by Sahana et al. (Sahana et al. Citation2010). Highest respective yield (%) values and loading efficiency obtained in case of the formulations PLGA-BCL-5ALS and PLGA-BCL-4ALS are shown in . Yields (%) of various experimental nanoparticles were between 44.39% and 87.45% (). It can be attributed that, less percentage yield due to recovery problem of the highly viscous emulsion (O/W), which have the tendency to stick on the surface of the inner wall of the homogenizer and glass container and was not fully recovered. The variation in loading efficiency might be due to the separation of smaller particles at higher centrifugal speed, which could entrap a smaller amount of drug. Batches separated at lower centrifugal speed (5500 rpm) had higher drug entrapment as compared to those separated at higher centrifugal speed (12,000 rpm). Batch no PLGA-BCL-5 (drug:polymer, 1:5) was found to be suitable to develop optimized formulation using 4% PVA with 14,000 rpm homogenization speed and low centrifugation speed for separation. Formulation PLGA-BCL-5E-LS was found as optimized batch with particle size of 270 nm, nanoparticles recovery of 87.62%, loading efficiency of 58.89%, polydispersity index of 0.221, and zeta potential of −30.34 mV (). The optimized formulation was prepared several times in three replicates for further characterization.
Figure 5. Effect of PVA concentration in the external aqueous phase of the single emulsion used for nanoparticles formulation on the percent of residual PVA associated with nanoparticles.
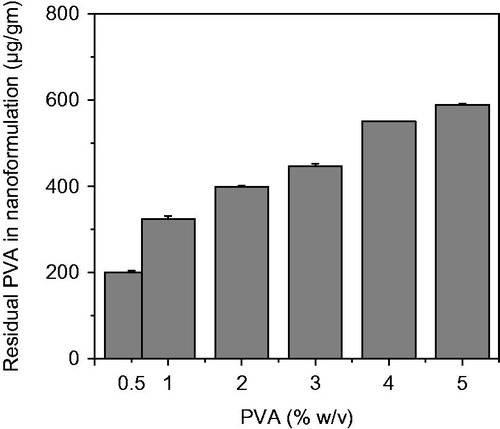
Figure 6. FESEM micrographs of (a) PLGA-BCL-5E-LS in low magnification and (b) PLGA-BCL-5E-LS in high magnification.
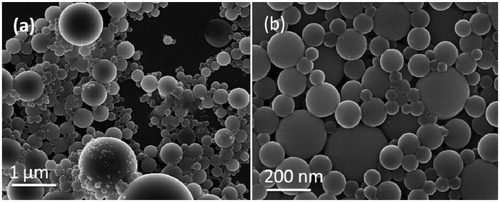
Table 3. Effect of polymer (PLGA) concentration over mean diameter of particle in different batch (Where polymer concentration increased).
Table 4. Percentage yield and loading efficiency of the experimental formulations.
Table 5. Results of optimized nanoparticles formulation(PLGA-BCL-5E-LS).
exhibits the FTIR spectra of active drug bicalutamide, PLGA and freshly prepared optimized formulation PLGA-BCL-5E-LS. The FTIR spectra of pure drug () exhibit the absorption bands 3340 cm−1 corresponding to (NH stretch), 1689 cm−1 (C=O stretch), 2235 cm−1 (C = N stretch) and 3490 cm−1 (H-bonded OH stretch) (Sancheti et al. Citation2008). exhibits vibration bands of PLGA, due to stretching of alkyl group (2850–3000−1), carbonyl –C=O stretching (1700–1800−1), C–O stretching (1050–1250−1) and –OH stretching (3200–3500−1) (Erbetta et al. Citation2012). Whereas the drug peak shown in FTIR spectra of the PLGA-BCL-5E-LS nanoparticles can be attributed to the fact that during preparation of the KBr disk of nanoparticles under high pressure and mixing, the nanoparticles were crushed and drug came out. The optimized formulation of Bicalutamide loaded PLGA nanoparticles PLGA-BCL-5E-LS shows almost all peaks of Bicalutamide with decreased peak intensity (). The data suggest that there was no chemical interaction between the drug and the polymer PLGA since no shifting of characteristic peaks of drug as well as polymer was noticed as the shifting of characteristic peak is claimed as chemical interaction. The drug entrapment is further characterized by XRD and DSC.
shows XRD diffractogram of pure PLGA polymer, Bicalutamide and freshly prepared lyophilized powder of PLGA-BCL-5E-LS nanoparticles. The XRD pattern of Bicalutamide showed () intense and sharp peaks, indicating its crystalline nature of the pure drug itself. Bicalutamide showed sharp peaks at 2θ around 6.35, 12.29, 17.79, 23.25, 24.22, 29.1, 29.42, 31.55, and 34.62° (Dash et al. Citation2015). In case of PLGA polymer a broad hump was observed at 2θ value 18.89°, confirming the amorphous nature of the polymer (Kasperczyk et al. Citation1996). On the other hand Bicalutamide loaded optimized formulation exhibit less intensity of the diffraction peak compared to pure drug. This clearly indicate that the encapsulation of the Bicalutamide within the PLGA polymer (Venkatesh et al. Citation2015).
The hydrodynamic diameter of PLGA nanoparticles and bicalutamide-encapsulated optimized formulation PLGA-BCL-5E-LS aqueous suspension are measured by light scattering and are given in . In this study, Blank-PLGA nanoparticles (without bicalutamide) was taken as standard for comparison of particle size. The average particle size of PLGA nanoparticles in aqueous suspension was found 183 nm with polydispersity index (PDI 0.126 ± 0.112). But, very interestingly we found the particle size increase up to 270 nm (PDI 0.221 ± 1.09) for optimized formulation PLGA-BCL-5E-LS when conjugated with bicalutamide. Zeta potential also plays an important role by preventing drug-loaded nanoparticles to aggregate. More −/+ the zeta potential more is the repulsion of nanoparticle so they can remain far apart without aggregation. We found zeta potential values for both drug-loaded optimized formulation and unloaded one was −30.34 mV and −31.29 mV, respectively, confirming excellent stability of the optimized formulation.
To understand the possible interactions between bicalutamide and PLGA the polymer, DSC analysis was performed with pure drug and both unloaded and bicalutamide-loaded optimized nanoparticle formulation (PLGA-BCL-5E-LS). Thermograms are typical of PLGA, exhibiting a glass temperature transition around 40 °C () and another peak around 310 °C (Erbetta et al. Citation2012, Kasperczyk Citation1996, Kranz et al. Citation2000). In case of pure drug bicalutamide, a sharp melting endotherm was found at temperature around 194 °C () (Sancheti et al. Citation2008). However, such a peak is not visible in the thermogram of PLGA-BCL-5E-LS. Very interestingly, it was observed that on encapsulation of bicalutamide to the PLGA its endothermic peak shifted toward lower temperature from 310 °C to 295 °C for PLGA (). Hence, from the previously said study it can be suggested that there is no crystalline drug material in the nanosphere samples. It could be concluded that in prepared optimized nanoformulation the drug was present in the amorphous phase and may have been homogeneously dispersed throughout the polymer matrix at an equal molecular level (Hariharan and Price, Citation2002).
To develop the different batch of nanoparticles formulation, different concentration (0.5 to 5% w/w) of PVA was used as emulsion stabilizer (). It was clearly observed that as the concentration of PVA was increased in external aqueous phase to stabilize the O/W emulsion, increase the residual amount of PVA associated with the drug-loaded nanoparticles. Earlier, Iler (Citation1975) proposed that hydroxyl groups of PVA molecules are fixed to the acetyl groups of PLGA via hydrophobic bond, which cannot be removed by washing for several time. In the present study, we observed that higher concentration of PVA in the continuous phase could lead to an increase in PVA molecule density at the O/W inter phase of emulsion droplet, which may increase the thickness of PVA on surface (Boury et al. Citation1995, Murukami et al. Citation1997 and Citation1999). The batch of bicalutamide-loaded PLGA nanoparticles formulated with 3, 4 and 5% PVA were found to be more hydrophilic compared to those formulated with 0.5 and 1% PVA as seen from the lower binding constants of Rose Bengal to the surface of the nanoparticles (). PVA is a hydrophilic polymer than PLGA and therefore the higher amount of residual PVA at the surface of the nanoparticles formulated with 5% PVA () could account for their higher hydrophilicity.
The optimized formulation of Bicalutamide loaded PLGA nanoparticles were found spherical in shape as shown in . The size was around 300 nm with good uniformity, which is consistent with the result determined by the laser light scattering.
In vitro drug release study of Bicalutamide from optimized formulation PLGA-BCL-5E-LS were performed as per the protocol mentioned in the experimental section. The release behavior of the pure drug Bicalutamide and optimized formulation shown in . The release of drug from the polymer matrix shows a 'bi-phasic' pattern with an initial 'burst release' followed by slower sustained release. An initial burst release of drug around 58% was observed up to 24 h may be due to presence of loosely bound Bicalutamide on/or near the surface of particles. Whereas a constant slow drug release was observed up to (98%) 120 h (). In case of pure drug around 90% drug released within 6 h (). The drug release data fitted into different kinetics model i.e. first order, zero order, Higuchi model and Korsmeyer–Pappas model to understand the release mechanism (Dash et al. Citation2010). The best fit kinetic model was found Higuchi model as the maximum correlation coefficient (R2) value was found 0.9991, indicating the release of Bicalutamide from nanoparticles was diffusion controlled mechanism, as the polymer matrix disintegrated slowly (Huang et al. Citation2001).
The half maximal inhibitory concentration or IC50 value of bicalutamide was determined to measure the potency of the drug by inhibiting proliferation of DU-145 cell line, which can be considered as a direct measure of antagonist drug power. shows the DU-145 cell viability in a media containing varying concentration of bicalutamide, as mentioned in experimental section. shows that at concentration of 40 μg/ml of drug, the cell viability was 50%. Hence, the cell viability at IC50 concentration of PLGA-BCL-5E-LS (equivalent amount of formulation containing 40 μg of drug) and pure bicalutamide was evaluated at 24, 48 and 72 h incubation time intervals at 37 °C using DU-145 cells and is shown in . In case of optimized formulation, no significant cell inhibition was observed after 24 h. However, after 48 h incubation, at the same concentration, optimized nanoparticles formulation was found to inhibit 50% of the cells, compared to the control. In case of the pure drug (bicalutamide), 50% cell inhibition was observed after 72 h compared to control as shown in . These results confirm that higher efficacy of PLGA-BCL-5E-LS optimized nanoparticles formulation compared to the bare bicalutamide, under identical concentration (40 μg/ml) on DU-145 cells. As expected, bare PLGA nanoparticles without bicalutamide have shown no cytotoxicity as the control on the proliferation (∼99%), at 48 and 72 h incubation in both the cases confirming the inertness of PLGA.
Several literatures reported that cellular uptake of nanoparticles depends on size of the carrier matrix, the uptake decreases with increase in size with surface hydrophilicity (Yameen et al. Citation2014). In the present study of optimized formulation of PLGA-BCL-5E-LS in DU 145 (), we found that the drug-loaded nanoparticles uptake was significantly increased with respect to time compared to pure drug. At all the time period 0.5h, 1h and in 2 h drug concentration was found more in case of drug-loaded nanoparticles. A number of literatures demonstrate that cellular uptake may be via phagocytic or via endocytosis mechanism depending on the particle size (Suh et al. Citation1998, Tabata and Ikada 2005). From our study, we found that intracellular uptake of optimized formulation fluid-phase endocytosis, as the particle size of the optimized nanoparticles <500 nm (Foster et al. Citation2001). It can also be explained that the pure drug uptake by simple diffusion mechanism depend on the concentration gradient mechanism (Kohler et al. Citation2005).
Very interesting feature was observed when the incubation temperature was reduced from 37 ° C to 4 °C with optimized formulation (). It was clearly observed that at same loading concentration cellular uptake of optimized formulation was significantly decreased at all the time period (30 min, 1 h and 2 h) compared to the uptake found at 37 °C. Earlier, Cui et al. developed a cationic nanoparticle-based DNA vaccine delivery system by O/W microemulsion techniques, and studied cellular uptake study with mannose receptor positive (MR+) mouse macrophage cell line (J774E) using nanoparticles coated or uncoated with mannan as a ligand precursors at two different temperature 37 °C and 4 °C (Cui et al. Citation2003). They reported that after 1 h incubation at 37 °C, the uptake of mannan-coated nanoparticles was 50% higher than that of the uncoated nanoparticles, whereas in case of 4 °C, after 1 h, the binding of the mannan-coated nanoparticles by J774E was twofold higher than that of the uncoated nanoparticles. The increase of binding to J774E could be abolished by preincubating the cells with free mannan, suggesting that the binding and uptake were receptor-mediated in cells. Another researcher, Mo and Lim reported that significant reduction in cellular uptake of two different types of nanoparticles at 4 °C, and suggesting that the nanoparticles bind to the receptor on the cell membrane or uptake governed by receptor-mediated endocytic process, confirmed by confocal microscopy (Mo and Lim 2004). There are a number of literature demonstrating that endocytic pathways are energy-depended, or temperature sensitive process is blocked at low temperatures. In our present study, we observed that nanoparticles uptake was markedly decreased at low temperature (4 °C) suggesting that uptake is mediated by endocytosis mechanism and the process is energy dependent.
Figure 7. (a) Cumulative release of pure drug Bicalutamide and (b) from PLGA-BCL-5E-LS as a function of time in PBS at pH 7.4.
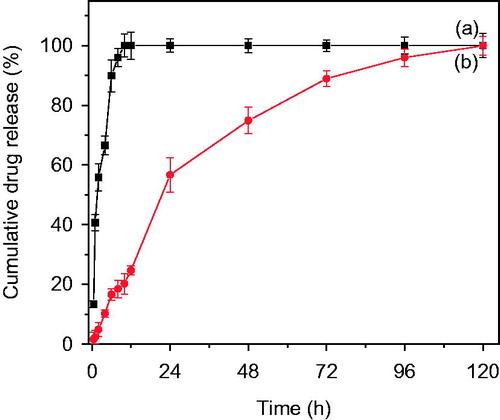
For the stability study of the drug in formulations, the samples were kept at different temperatures (at 25 °C, 45 °C, and 60 °C) for 90 days following ICH guidelines and drug content study of the formulation were determined by UV spectroscopy and compared with those of the freshly prepared formulations. The study suggests that the drug remained stable in the formulations at those storage conditions and there was no physicochemical interaction between the drug and the excipients or degradation of optimized formulation under the storage conditions.
Conclusion
In the present work, we reported the effect of different preparation parameters including polymer concentration, surfactant concentration, and centrifugation force for separation of drug (bicalutamide)-loaded PLGA nanoparticles to develop an optimized formulation within the size range of 200–400 nm with a better EE of more than 50%. The result presented herein showed that an increase in concentration of polymer, mean diameter of the particle. PVA content have also affected the morphological and size characteristics. Meanwhile, it came to light that optimum PVA concentration and optimized centrifugation speed yield better nanoparticles.
Finally, the optimized nanoparticles formulation loaded with bicalutamide was characterized and their surface morphology, thermal stability, and drug release profile has been investigated and compared with pure drug. XRD studies suggested the encapsulation of drug moiety within the polymer matrix. Spherical globular shapes of 200–300 nm size of the optimized formulation was confirmed by SEM. Bicalutamide release from optimized nanoparticles formulation appeared to have two phasic systems. An initial burst release due to surface associated drug followed by slower exponential release of the drug which was dissolved in the core of the polymeric nanoparticles. Further, in vitro evaluation of optimized formulation of bicalutamide-loaded nanoparticles showed a strikingly higher sensitivity/efficacy, confirmed by attainment of the IC50 of bicalutamide on DU-145 cell lines by a period of 48 h in comparison to the same of the bare unbound drug bicalutamide by a period of 72 h. The optimized formulation of nanoparticles were internalized well in prostate cancer cells and thereby suggesting their suitability in prostate cancer treatment.
Figure 8. (a) The half maximal inhibitory concentration (IC50) of bicalutamide using DU-145 cell line. (b) DU-145 cell viability of nanoparticles with PLGA-BCL-5E-LS and without bicalutamide drug. Statistical analysis was performed using Student’s t-test to compare the cell viability study between the optimized formulation and free drug groups. The level of significance was p < 0.05.
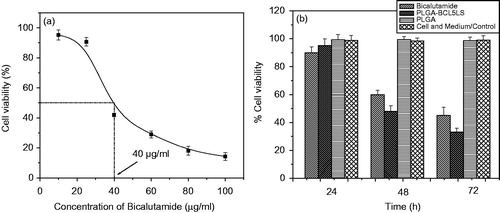
Figure 9. (a) Time-depended cellular uptake of bicalutamide-loaded optimized formulation in time depended manner at 37 °C and (b) the uptake of optimized formulation to DU-145 cells at different time points after incubation at 4 °C. Statistical analysis was performed using Student’s t-test to compare the drug uptake study by cell study between the optimized formulation and free drug groups. The level of significance was p < 0.05.
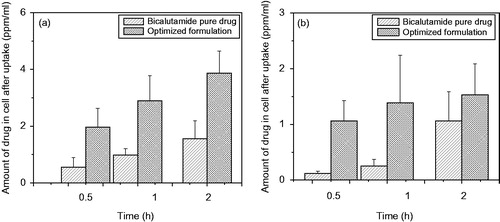
Acknowledgements
The authors would like to acknowledge central characterization facility, Indian Association of Cultivation of Sciences, Kolkata, for providing instrumental facility.
Disclosure statement
The authors [S. Ray, S. Ghosh (Ray), S. Mandal] declare that they have no conflicts of interest.
References
- Adams ML, Lavasanifar A, Kwon GS. 2003. Amphiphilic block copolymers for drug delivery. J Pharm Sci. 92:1343–1355.
- Aulton ME. 1988. Pharmaceutics: The Science of Dosage Form Design. 4th ed. London, UK: Churchill Livingstone, pp. 69–86.
- Bolourchian N, Mahboobian MM, Dadashzadeh S. 2013. The effect of PEG molecular weights on dissolution behavior of simvastatin in solid dispersions. Iran J Pharm Res. 12:11–20.
- Boury F, Ivanova T. Panaiotov, et al. 1995. Dynamic properties of poly (D, L-lactide-co-glycolide) and Polyvinyl alcohol monolayers at the air/water and dichloromethane air/water interfaces. J. Colloid Interface Sci. 169:380–392.
- Budhian A, Siegel SJ, Winey KI. 2005. Production of haloperidol-loaded PLGA nanoparticles for extended controlled drug release of haloperidol. J Microencapsul. 22:773–785.
- Clarke GS. 1994. The validation of analytical methods for drug substances and drug products in UK pharmaceutical laboratories. J Pharm Biomed Anal. 12:643–652.
- Cui Z, Hsu CH, Mumper RJ. 2003. Physical characterization and macrophage cell uptake of mannan-coated nanoparticles. Drug Dev Ind Pharm. 29:689–700.
- Dash S, Murthy PN, Nath L, Chowdhury P. 2010. Kinetic modeling on drug release from controlled drug delivery systems. Acta Pol Pharm. 67:217–223.
- Dhas NL, Ige PP, Kudarha RR. 2015. Design, optimization and in-vitro study of folic acid conjugated-chitosan functionalized PLGA nanoparticle for delivery of bicalutamide in prostate cancer. Powder Tech. 283:234–245.
- Erbetta CD, Alves RJ, Resende JM, de Souza Freitas RF, de Sousa RG. 2012. Synthesis and characterization of poly (D, L-lactide-co-glycolide) Copolymer. J Biomater Nanobiotechnol. 3:208–210.
- Foster KA, Yazdanian M, Audus KL. 2001. Microparticulate uptake mechanisms of in-vitro cell culture models of the respiratory epithelium. J Pharm Pharmacol. 53:57–66.
- Hariharan M, Price JC. 2002. Solvent, emulisifier and drug concentration factors in poly-(D-L-Lacticacid) microspheres containing hexamethylmelamine. J Microencap. 19:95–109.
- Herrero-Vanrell R, Ramirez L, Fernandez-Carballido A, Refojo MF. 2000. Biodegradable PLGA microspheres loaded with ganciclovir for intraocular administration. Encapsulation technique, in vitro release profiles, and sterilization process. Pharm Res. 17:1323–1328.
- Hoskins C, Thoo-Lin PK, Cheng WP. 2012. A review on comb-shaped amphiphilic polymers for hydrophobic drug solubilization. Ther Deliv. 3:59–79.
- Hsu HC, Chien CS. 1994. Validation of analytical methods: a simple model for HPLC assay methods. Food Drug Anal. 2:161–176.
- Huang X, Brazel CS. 2001. On the importance and mechanisms of burst release in matrix-controlled drug delivery systems. J Control Release. 73:121–136.
- Iler RK. 1975. Coacervates of polyvinyl alcohol and colloidal silica. J Colloid Interface Sci. 51:388–393.
- Jain S, Mittal AK, Jain R, Mahajan R, Singh D. 2010. Cyclosporin A loaded PLGA nanoparticle: preparation, optimization, in-vitro characterization and stability studies. Curr Nanosci. 6:422–431.
- Jeffery H, Davis SS, O'Hagan DT. 1991. The preparation and characterisation of poly(lactide-co-glycolide) microparticles. I: oil-in-water emulsion solvent evaporation. Int J Pharm. 77:169–175.
- Kasperczyk J. 1996. Microstructural analysis of poly[(l, l-lactide)-co-(glycolide)] by 1H and 13C NMR spectroscopy. Polymer. 37:201–203.
- Kohler N, Sun C, Wang J, Zhang M. 2005. Methotrexate-modified super paramagnetic nanoparticles and their intracellular uptake into human cancer cells. Langmuir. 21:8858–8864.
- Kranz H, Ubrich N, Maincent P, Bodmeier P. 2000. Physicomechanical properties of biodegradable poly(D,L-lactide) and poly(D,L-lactide-co-glycolide) films in the dry and wet states. J Pharm Sci. 89:1558–1566.
- Lawrence MJ, Rees GD. 2000. Microemulsion-based media as novel drug delivery systems. Adv Drug Deliv Rev. 45:89–121.
- Lee SC, Oh JT, Jang MH, Chung SI. 1999. Quantitative analysis of polyvinyl alcohol on the surface of poly(D, L-lactide-co-glycolide) microparticles prepared by solvent evaporation method: effect of particle size and PVA concentration. J Control Release. 59:123–132.
- Li C, Le Y, Chen JF. 2011. Formation of bicalutamide nanodispersion for dissolution rate enhancement. Int J Pharm. 404:257–263.
- Mainardes RM, Evangelista RC. 2005. PLGA nanoparticles containing praziquantel: effect of formulation variables on size distribution. Int J Pharm. 290:137–144.
- Maji R, Dey NS, Satapathy BS, Mukherjee B, Mondal S. 2014. Preparation and characterization of Tamoxifen citrate loaded nanoparticles for breast cancer therapy. Int J Nanomed. 9:3107–3118.
- Masiello D, Cheng S, Bubley GJ, Lu ML, Balk SP. 2002. Bicalutamide functions as an androgen receptor antagonist by assembly of a transcriptionally inactive receptor. J Biol Chem. 277:26321–26326.
- McGowen R, Biliran H, Sager R, Sheng S. 2000. The surface of prostate carcinoma DU145 cells mediates the inhibition of urokinase-type plasminogen activator by maspin. Cancer Res. 60:4771–4778.
- Mo Y, Lim LY. 2004. Mechanism study of the uptake of wheat germ agglutinin-conjugated PLGA nanoparticles by A549 cells. J Pharm Sci. 93:20–28.
- Müller RH. 1991. Colloidal Carriers for Controlled Drug Delivery and Targeting: Modification, Characterization, and In Vivo Distribution. Stuttgart Boca Raton: Wissenschaftliche Verlagsgesellschaft, CRC Press. pp. 38–44.
- Murakami H, Kobayashi M, Takeuchi H, Kawashima Y. 1999. Preperation of poly (D, L-lactide-co-glycolide) nanoparticles by modified spontaneous emulsification solvent diffusion method. Int J Pharm. 187:143–152.
- Murukami H, Kawashima Y, Nawa T, Hino T, Takeuchi H, Kobayashi M. 1997. Influence of the degree of hydrolization and polymerization of polyvinyl alcohol on the preperation and properties of PLGA nanoparticles. Int J Pharm. 149:43–49.
- Nakano M. 2000. Places of emulsions in drug delivery. Adv Drug Deliv Rev. 45:1–4.
- Okamoto R, Delansorne R, Wakimoto N, Doan NB, Akagi T, Shen M, et al. 2012. Inecalcitol, an analog of 1α,25(OH)(2) D(3), induces growth arrest of androgen-dependent prostate cancer cells. Int J Cancer. 130:2464–2473.
- Qiu LY, Bae YH. 2006. Polymer architecture and drug delivery. Pharm Res. 23:1–30.
- Saeedi M, Akbari J, Morteza-Semnani K, Enayati-Fard R, Sar-Reshteh-Dar S, Soleymani A. 2011. Enhancement of dissolution rate of indomethacin: using liquisolid compacts. Iran J Pharm Res. 10:25–34.
- Sahana B, Santra K, Basu S, Mukerjee B. 2010. Development of biodegradable polymer based tamoxifen citrate loaded nanoparticles and effect of some manufacturing process parameters on them: a physicochemical and in-vitro evaluation. Int J Nanomed. 5:621–630.
- Sahoo S, Panyam J, Prabha S, Labhasetwar V. 2002. Residual polyvinyl alcohol associated with poly (D,L-lactide-co-glycolide) nanoparticles affects their physical properties and cellular uptake. J Control Release. 82:105–114.
- Sancheti PP, Vyas MV, Shah M, Karekar P, Pore YV. 2008. Development and characterization of bicalutamide-poloxamer F68 solid dispersion systems. Pharmazie. 63:571–575.
- Schleich N, Sibret P, Danhier P, Ucakar B, Laurent S, Muller RN, et al. 2013. Dual anticancer drug/superparamagnetic iron oxide-loaded PLGA-based nanoparticles for cancer therapy and magnetic resonance imaging. Int J Pharm. 447:94–101.
- Schwendener RA, Schott H. 1996. Lipophilic 1-beta-D-arabinofuranosyl cytosine derivatives in liposomal formulations for oral and parenteral antileukemic therapy in the murine L1210 leukemia model. J Cancer Res Clin Oncolo. 122:723–726.
- Suh H, Jeong B, Liu F, Kim SW. 1998. Cellular uptake study of biodegradable nanoparticles in vascular smooth muscle cells. Pharm Res. 15:1495–1498.
- Sun BS, Liu P, Shao FM, Miao QL. 2015. Formulation and evaluation of PLGA nanoparticles loaded capecitabine for prostate cancer. Int J Clin Exp Med. 8:19670–19681.
- Svenson S. 2009. Dendrimers as versatile platform in drug delivery applications. Eur J Pharm Biopharm. 71:445–462.
- Swamivelmanickam M, Gomes AR, Manavalan R, Satyanarayana D, Reddy PG. 2009. Determination and validation of UV spectrophotometric method for estimation of bicalutamide tablets. Int J ChemTech Res. 4:1189–1193.
- Tabata Y, Ikada Y. 2005. Phagocytosis of polymer microspheres by macrophages. Adv Polymer Sci. 94:107–141.
- Vasconcelos T, Sarmento B, Costa P. 2007. Solid dispersions as strategy to improve oral bioavailability of poor water soluble drugs. Drug Discov Today. 12:1068–1075.
- Veiga F, Teixeira-Dias JJ, Kedzierewicz F, Sousa A, Maincent P. 1996. Inclusion complexation of tolbutamide with β-cyclodextrin and hydroxypropyl-β-cyclodextrin. Int J Pharm. 129:63–71.
- Venkatesh DN, Baskaran M, Karri VV, Mannemala SS, Radhakrishna K, Goti S. 2015 Fabrication and in vivo evaluation of nelfinavir loaded PLGA nanoparticles for enhancing oral bioavailability and therapeutic effect. Saudi Pharm J. 23:667–674.
- Yameen B, Choi WI, Vilos C, Swami A, Shi J, Farokhzad OC. 2014. Insight into nanoparticle cellular uptake and intracellular targeting. J Control Release. 190:485–499.
- Yang YY, Chung TS, Ng NP. 2001. Morphology, drug distribution, and in vitro release profiles of biodegradable polymeric microspheres containing protein fabricated by double-emulsion solvent extraction/evaporation method. Biomaterials. 22:231–241.