Abstract
In the present investigation, polyethylenimine (PEI) was conjugated with succinic anhydride at four substitution degrees and the efficiency of the modified PEI derivatives in transferring the plasmid encoding interleukin-12 gene was evaluated. The results revealed that the conjugated PEI derivatives enhanced the transfection efficiency by up to 3-fold relative to unmodified PEI, with the highest increase occurring at conjugation degrees around 30%. The results demonstrated the ability of the modified PEI derivatives in condensation of the plasmid into the nanoparticles in the size range of approximately 100 nm. Also, the PEI derivatives exhibited substantial decrease in cell-induced toxicity.
Introduction
Cancer immune therapy has been considered as an effective route to stimulate immune response in order to treat several diseases including cancer (Hanci et al. Citation2016, Nezafat et al. Citation2015, Radford et al. Citation2014). Among the cytokines used for immunotherapy, great attention has been directed to interleukin-12 (IL-12) as a powerful cytokine for cancer immunotherapy (Choi et al. Citation2012). IL-12 is a heterodimeric glycoprotein composed of p35 and p40 subunits connecting via a disulfide bridge (Watford et al. Citation2003). This cytokine is able to improve T-cell differentiation, NK cell activation, promote cytotoxic T lymphocytes (CTLs) and macrophages as well as induction of IFN-γ and TNF-α (Choi et al. Citation2012). In other words, IL-12 acts as a key player in critical steps of innate and adaptive immunity through its regulatory functions. The systemic administration of IL-12 protein has led to severe side effects including systemic cytotoxicity (Orange et al. Citation1995). Therefore, the request for immunotherapy using IL-12 protein has been limited worldwide (Dehshahri et al. Citation2014). In order to reduce the systemic toxic effects associated with the administration of recombinant protein (Dass et al. Citation2010, Zhao et al. Citation2011), several investigations are underway for local production of IL-12. The aim of this approach is to produce IL-12 protein inside the target tumor tissues to increase the effectiveness of the IL-12 protein and reduce its toxicity. Transferring plasmids with the ability of cytokine expression inside tumor sites needs appropriate carriers for their entry into the target cells with low toxicity (Zintchenko et al. Citation2008). Recently, more efforts have been conducted on the development of non-viral vectors due to their low safety risks and production costs as well as their flexibility for chemical modification compared to the viral gene carriers (Ebrahiminezhad et al. Citation2013, Parhiz et al. Citation2013, Quezada et al. Citation2004). Nevertheless, low transfection efficiency and relatively high cytotoxicity have hampered the wide clinical application of non-viral carriers. Therefore, functionalization of non-viral delivery systems using various chemical moieties including hydrophobic chains, amino acids, carbohydrates polyethylene glycol (PEG), and targeting ligands could be considered as an effective strategy to improve the transfection efficiency and decrease the cytotoxicity (Kafshdooz et al. Citation2016, Kim et al. Citation2015, Malakooty Poor et al. Citation2014, Oskuee et al. Citation2009, Sabahi et al. Citation2015, Zhang et al. Citation2016). Nanoparticle forming polymers have demonstrated great potential for the delivery of nucleic acid materials such as plasmid encoding therapeutic genes as well as siRNA, aptamers, anitisense oligonucleotides, and more recently CRISPR/CAS9 (Li et al. Citation2015). Previous studies have shown the great ability of polyethylenimine (PEI) to transfer genetic materials in vitro and in vivo (Boussif et al. Citation1995). Since PEI has been applied as the golden standard of polycations for gene delivery, enormous investigations have been carried out to improve its characteristics for further purposes (Alvarez et al. Citation2014, Forrest et al. Citation2004, Lee et al. Citation2003, Mao et al. Citation2006). PEI intrinsic cytotoxicity is remarkably associated with the high positive charge density of the polymer resulting in the interaction with the negatively charged cell surfaces and consequently the damage of the cell membranes (Paul et al. Citation2014). Hence, researchers are seeking for new chemical modification routes to modulate the positive surface charge of the polymer (Forrest et al. Citation2004). Based on the previous investigations, acetylation, succinylation, and the introduction of various acrylates into the PEI structure have been considered as promising modification approaches for the improvement of PEI characteristics (Zintchenko et al. Citation2008). These types of conjugation strategies have been utilized to adjust the hydrophilic–hydrophobic balance of PEI along with the modulation of its cytotoxic effects.
In the present study, we modified high molecular weight 25 kDa branch PEI by succinic anhydride at four conjugation degrees to test the impact of various degrees of substitution on the effectiveness of PEI in transferring plasmid encoding IL-12 gene as well as its cytotoxicity, buffering capacity, plasmid binding affinity, and protection of the plasmid DNA against enzymatic degradation.
Materials and methods
Materials
Branched PEI (MW 25000), succinic anhydride, 3-(4,5-dimethylthiazol-2-yl)-2,5-diphenyl tetrazolium bromide (MTT) and N-[2-hydroxythyl] piperazine-N-[2-ethanesulfonic acid] (HEPES) were purchased from Sigma-Aldrich (Munich, Germany). Human IL-12 plasmid (pUMVC3-hIL12) and mammalian vector with independent tGFP expression (pCMV6-A-GFP) were obtained from Aldevron (Madison, WI) and Origene (Rockville, MD), respectively. Human IL-12 (p70) ELISA Kit was purchased from BD Biosciences (Heidelberg, Germany). Cell culture experiments were performed using fetal bovine serum (FBS) and Dulbecco’s Modified Eagle’s Medium (DMEM) (Gibco, Gaithersburg, MD). Spectra/Por dialysis membrane was obtained from Spectrum Laboratories (Houston, TX). TransformAid Bacterial Transformation Kit (K-2710) was purchased from Thermo Scientific Company (Hanover, MD). Plasmid purification was carried out by Qiagene Plasmid Giga Kit (Munich, Germany). All solvents and chemicals were purchased from Sigma-Aldrich (Munich, Germany).
Synthesis and characterization of succinated PEI derivatives
In order to prepare PEI solution, 25 kDa PEI was dissolved in water containing 3 M NaCl. Then, the pH was adjusted to 5 using 1 M HCl. The molar ratio between succinic anhydride and PEI polymer was adjusted to reach 10–40% of primary amines substitution. After 3 h of reaction at room temperature, dialysis was carried out against 0.25 M NaCl solution. Then, the products were dialyzed at double distilled water (DDW) for two additional times. Following the removal of unreacted succinates and salts, the solution was frozen at −70 °C and then lyophilized. The PEI conjugates with different substitution degrees were analyzed by 1H NMR (D2O) on a Bruker Avance DRX-500 MHz NMR spectrometer (Bruker, Ettlingen, Germany) and FT-IR spectrometer (Bruker, Germany).
Preparation of the plasmids and PEI/plasmid polyplexes
The plasmids pUMVC3-hIL12 and pCMV6-A-GFP were utilized to evaluate the efficiency of modified PEIs in gene delivery. Each plasmid was transformed into Escherichia coli DH5α by TransformAid Bacterial Transformation kit and purified using the Qiagene Plasmid Giga Kit according to the manufacturer’s protocol. The composition of the polyplexes (i.e., polymer/plasmid complexes) was characterized by the weight:weight ratios of the PEI and its conjugates (C) to plasmid (P) in the final formulation (C/P ratio). The formation of the polyplexes was carried out by mixing different concentration of PEI and its derivatives in HEPES-buffered glucose (HBG) solution with a plasmid solution (40 μg/mL) in the same buffer. Following an incubation time of 20–30 min at room temperature, the prepared polyplexes were used for further tests.
Particle size and ζ potential measurements
The particle size and ζ potential of the prepared polyplexes were measured by dynamic light scattering (DLS) and laser Doppler velocimetry (LDV), respectively, using Malvern Nano ZS (Malvern Instruments, Malvern, UK) in HGB buffer. Various concentrations of PEI and its derivatives were prepared in 125 μL of buffer and added to an equal volume of the same buffer containing the plasmid. Data were collected for 30 cycles in automatic mode and the results were reported as mean ± SEM (n = 3).
Plasmid DNA binding affinity
Gel retardation assay
The ability of the modified polymers to interact electrostatically with the plasmid DNA was assessed using gel retardation assay. The polyplexes were prepared at the same condition as described above. Then, 10 μL of each polyplex was loaded onto the agarose gel (1%) containing gel red dye (0.5 mg/mL) in TBE buffer. Electrophoresis was carried out at 50 V for 40 min and the plasmid bands were visualized under UV illuminator.
Ethidium bromide exclusion assay
The capacity of the modified PEI derivatives to condense the plasmid DNA was measured by a fluorescence quenching method. Briefly, the solution of plasmid (20 μg/mL) in HBG containing ethidium bromide (0.4 μg/mL) was prepared and the fluorescence intensity of this solution was measured at the excitation of 510 nm and emission of 590 nm and set to 100%. Then, the polymer solution was added stepwise to the plasmid solution. The decrease of the fluorescence intensity following each addition of the polymer solution was recorded and the graph was drawn by plotting the relative fluorescence intensity (percentage) against weight to weight ratios of the PEI and its derivatives to the plasmid (C/P ratio).
Protection of the plasmid against DNase I degradation
In order to test the effect of PEI conjugation degree on the protection of plasmid against nuclease digestion, DNase I was utilized as an enzyme model. Following the formation of the polyplexes at varous C/P ratios, DNase I (0.25 units) was added to the complex solution and incubated for 30 min at 37 °C. Then, EDTA (250 mM) and sodium dodecyl sulfate (SDS) solutions were added to inactivate the enzyme and allow the dissociation of the plasmids from polyplexes, respectively. Finally, agarose gel electrophoresis was carried out to visualize the location of the plasmid bands.
Evaluation of the buffering capacity of the modified PEIs
In order to evaluate the buffering capacity of the PEI derivatives following the conjugation, polymers (0.8 mg) were dissolved in 2 mL of DDW and the pH value was adjusted to 12 by 1 M NaOH. Then the polymer solution was titrated by 1 M HCl to pH =2. The slope of the plot of pH against added HCl demonstrates the intrinsic buffering capacity of the unmodified PEI and its derivatives.
Hemolysis assay
Freshly human blood isolated erythrocytes were washed three times using 150 mM NaCl solution. Then, the erythrocyte pellet was diluted in phosphate-buffered saline (PBS) to yield a solution with 106 RBSs per 50 μL. The suspension of RBCs was immediately used for the assay. Unmodified PEI and its derivatives were serially diluted in HEPES-buffered saline (HBS) per well using triplicates in a V-bottom 96-well plate to reach the final concentrations of 0.2–2 mg/mL. Following the addition of 20 μL of the RBC suspension to each well, the plates were incubated at 37 °C for 45 min. Polymers with the membrane-lytic activity disrupt the erythrocyte cell membrane leading to the release of hemoglobin to the solution. Negative and positive control wells contained 150 μL of phosphate buffer alone and buffer with 1%Triton-X-100, respectively. Finally, the plates were centrifuged at 800 × g for 10 min followed by the analysis of supernatant for hemoglobin release at 550 nm using a microplate reader.
Cell culture and cell viability assay
Human HepG2 hepatocellular carcinoma cells (NCBI C158, Tehran, Iran) were maintained at 37 °C, 5% CO2 in DMEM supplemented with 10% FBS, streptomycin at 100 μg/mL, and penicillin at 100 U/mL. One day prior the cytotoxicity experiments, cells were seeded at the density of 1 × 104 cells/well in 96-well trays and grown at the appropriate culture medium containing FBS for 24 h. To prepare the polyplex formulation, polymer solutions at different concentrations in HBG buffer were prepared. Also, the plasmid solutions (40 μg/mL) were prepared at the same buffer in separate tubes. Then, 50 μL of the PEI solution was added to the plasmid solution to prepare various C/P ratios ranging from 0.25:1 to 8:1 at the final volume of 100 μL. In order to carry out the viability assay, 10 μL of the polyplex formulation was added to the wells of 96-well plates followed by the medium replacement with 100 μL fresh DMEM growth medium containing FBS after 4 h. Twenty-four hours later, the medium was aspirated and the MTT solution (5 mg/mL) was added to each well and incubated for 1.5 h. Following the incubation period, the formazan crystals were dissolved in 100 μL/well dimethyl sulfoxide (DMSO) and the absorbance was measured photometrically by an ELISA reader at 590 nm and background corrected at 630 nm. Data are presented as mean ± SD; n = 3.
Transfection procedure and the expression of the transgenes
In order to evaluate the ability of modified PEI conjugates in transferring the plasmid encoding human IL-12 gene, 10 μL of the polyplex formulations at various C/P ratios (i.e., 0.25:1, 4:1, and 8:1) was added to each well and the further treatments were performed as described for cytotoxicity assay. Following 48 h, the cell supernatants were collected for hIL-12 quantification. The level of hIL-12 in the cell supernatants was measured using the human IL-12 (p70) ELISA kit (BD Bioscience, Heidelberg, Germany) according to the manufacturer’s protocol. The results were normalized and presented as hIL-12 (pg/mL)/seeded cells. Furthermore, flow cytometry was carried out by seeding the cells into a 6-well plate at the density of 1 × 106 cells/well. The cells were treated with the polyplex formulation as described above and analyzed by flow cytometry (FACSCalibur, Becton Dickinson, Mountain View, CA) using FlowJo software (TreeStar Inc., San Carlos, CA). The cells treated with no plasmid or polyplex (i.e., with medium only) were considered as negative control.
Statistical analysis
Data are presented as the mean ± SD. The statistical significance was determined using Student’s t-test, and P values <0.05 were considered significant.
Results and discussion
Synthesis of modified PEI derivatives
In the present study, PEI was conjugated to succinic anhydride at four substitution degrees of 10, 20, 30, and 40 mole percentage of the estimated number of primary amines (Scheme 1) to evaluate the effect of increased hydrophobicity and the conjugation degree on the polymer properties including pDNA condensation ability, particle size, zeta potential, buffering capacity, protection against enzyme digestion as well as transfection efficiency, and cytotoxicity. The PEI conjugates were labeled as PEI-SUC X% in which SUC stands for succinic anhydride, and X represents the percentage of primary amines on PEI structure conjugated by succinic anhydride. The degree of substitution was calculated by 1H-NMR in which the PEI peaks appeared between 2.7 and 3.2 ppm, whereas the methylene of succinate protons was found at 2.5 ppm. The results indicated that the conjugation degrees for PEI-SUC 10% and PEI-SUC 20% were in the range of 9–19% whereas the observed degrees of conjugation for PEI-SUC 30% and PEI-SUC 40% were found to be lower than the calculated percentage of conjugated amines, indicating that the complete conjugation of PEI amines was not achievable at higher reagent/amine feed ratios. According to FT-IR spectra, two new peaks were appeared at 1659 and 1715 cm−1 corresponding to the carbonyls of amide and carboxylic acid groups, respectively. Also a broad peak between 2500 and 3500 cm−1 indicated the presence of hydroxyl group of carboxylic acid in the PEI-SUC conjugates.
Measurement of the particle size and ζ potential
One of the major factors affecting the transfection efficiency of polycation-based delivery systems is the particle size and surface charge density of the carriers used for transferring plasmid DNA into the cells (Rezvani et al. Citation2013). In this study, the nanoparticles were formed by the electrostatic interaction between the positively charged PEI and its conjugates with the negatively charged plasmid DNA at HBG buffer. The results of the size analysis illustrated that unmodified PEI formed nanoparticles with the size of around 75 nm whereas the modified PEI conjugates led to the polyplexes in the size range of approximately 100 nm. In other words, the conjugation strategy used in this investigation resulted in the formation of polyplexes slightly larger than the nanoparticles formed by unmodified PEI; however, the size of the modified PEI-based nanoparticles is still in the size range enabling the complexes to show enhanced permeability and retention (EPR) effect which in turn leads to the potential accumulation of the polyplexes inside the target tumors (Petros and DeSimone Citation2010). The zeta potential analysis revealed that the conjugation of succinic anhydride to the PEI primary amines decreased the positive charge density from 34 mV in the case of unmodified PEI to 19.3 and 8.6 for PEI-SUC 10% and PEI-SUC 40%, respectively. Since the attachment of hydrophobic chains of succinic anhydride to the PEI structures occurs through the surface amines, it is expected that the ζ potential reduces (). Another explanation for the reduced ζ potential could be associated with the introduction of negatively charged carboxylic acids of suucinate groups on the PEI surface. Despite the grafting of the moieties containing negative charge on the PEI surface, the net charge of the polyplexes was still positive which is necessary for the cell entry through the interaction between the positively charged polyplexes and the negative surface of the cell membranes. The results of the particle size and zeta potential measurements is consistent with the investigations in which the conjugation of various hydrophobic moieties into the PEI via its surface amines increases the particle size and leads to a decrease in the positive surface charge of the polyplexes (Dehshahri et al. Citation2012, Doody et al. Citation2006, Forrest et al. Citation2004, Nimesh et al. Citation2007, Oskuee et al. Citation2008).
Table 1. Degree of primary amine conjugation in 25 kDa PEI estimated and size and zeta potential of the plasmid/DNA polyplexes.
The impact of the polyplex particle size on their biodistribution has been elucidated in several investigations. Generally, it has been proved that the polyplexes larger than 300 nm are removed by the reticuloendothelial system (RES) in various organs including lung, liver or spleen. On the other hand, the polyplexes smaller than 5 nm are rapidly removed from blood via renal clearance or extravasation. Furthermore, the size of the colloidal particles plays a key role in the mechanism by which the polyplexes enter the cells. These mechanisms include macropinocytosis for the polyplexes larger than 1000 nm whereas the particles with the size range of 60–120 nm can be internalized via several pathways including caveolar-mediated endocytosis, clathrin-mediated endocytosis, and clathrin-independent and caveolin-independent endocytosis. Therefore, the optimal size range for the polyplex uptake and favorable biodistribution for in vivo applications seems to be around 200 nm or less (Petros and DeSimone Citation2010). Another major factor affecting the association of the colloidal particles with the cell membrane is thought to be the charge of the polyplex surface. Although the positive surface charge enables the polyplexes to interact with the negatively charged cell surface components such as proteoglycans and subsequently their entrance to the cells, the high positive charge may be associated with the high toxicity of the PEI-based nanoparticles (vide infra). Furthermore, the high positive charge on the surface of the polyplexes may trigger the opsonization process which in turn leads to the removal of the particles from circulation. Therefore, reducing the particle surface charge has been considered as an effective way for addressing the negative properties associated with the high positive charge of the polyplexes (Petros and DeSimone Citation2010, Thomas and Klibanov Citation2002).
Plasmid DNA affinity test and gel retardation assay
One of the key characteristics of polycationic polymers to act as an efficient carriers for nucleic acid materials is their ability to interact with the negatively charged plasmid and form nano-sized complexes namely polyplex (Dehshahri et al. Citation2009). The binding affinity of the various PEI derivatives to plasmid DNA can be analyzed by ethidium bromide exclusion assay in which the added polycation (e.g., PEI-SUC) interacts with plasmid DNA and consequently excludes the EtBr from plasmid. This exclusion of the intercalating dye leads to a decrease in fluorescence intensity which is associated with the strength of polycation to bind electrostatically with plasmid DNA. As shown in , the relative fluorescence intensity of different PEI-SUC conjugates decreased in a similar manner as for the unmodified parent polymer. In other words, the conjugation degrees ranging from 10% to 40% could not change the DNA binding affinity at higher C/P ratios. However, at the lowest C/P ratio tested (i.e., 0.25), the fluorescence intensity decreased to 40–50% whereas the higher C/P ratios reduced the intensity around 80%. As shown in , the florescence intensity reaches to plateau at the C/P ≥ 2.
Figure 1. Plasmid DNA condensation by 25 kDa PEI and its conjugated derivatives determined by the ethidium bromide exclusion assay.
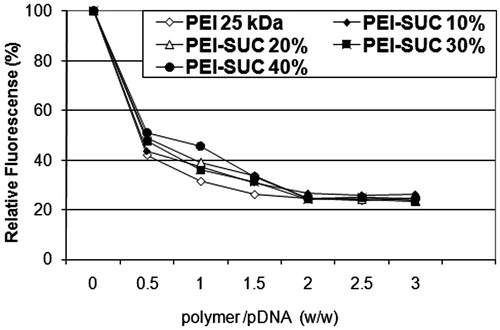
Since the binding of polycationic polymers to plasmid DNA occurs through electrostatic interaction, the formed complexes show retention in electrophoretic migration. exhibits the retardation assay for the different PEI conjugates at C/P ratios of 0.25, 4, and 8. Although this test was carried out at various C/P ratios including 1 and 2, the results were limited to three C/P ratios due to the similar patterns observed for various C/P ratios tested. As demonstrated in , all the polyplexes prepared either by unmodified PEI or conjugated derivatives could condense the plasmid DNA at C/P ratios ≥4 while the lowest C/P ratio tested (C/P = 0.25) resulted in the weakest condensation. This observation is consistent with the results obtained in the pDNA binding affinity test in which the optimal condensation was achieved at C/P ratios higher than 0.25. Based on the previous investigations, substitution of surface PEI amines by pendant moieties reduced the DNA binding affinity of the polymer particularly at lower polymer concentrations while no substantial difference was observed between the unmodified PEI and its conjugates at higher C/P ratios. Since the conjugation of primary surface amines reduced the ability of polymers to interact electrostatically with pDNA, more polymer was needed for the formation of tight complexes (Doody et al. Citation2006, Forrest et al. Citation2004, Gabrielson and Pack Citation2006, Nimesh et al. Citation2007, Sabahi et al. Citation2015).
Figure 2. Gel retardation assay of unmodified PEI and its derivatives at various carrier/plasmid (C/P) ratios. Lanes 1, 6, and 11: unmodified 25 kDa PEI/pDNA at C/P ratios of 0.25, 4, and 8, respectively; Lanes 2, 7, and 12: PEI-SUC 10%/pDNA at C/P ratios of 0.25, 4, and 8, respectively; Lanes 3, 8, and 13, PEI-SUC 20%/pDNA at C/P ratios of 0.25, 4, and 8, respectively; Lanes 4, 9, and 14, PEI-SUC 30%/pDNA at C/P ratios of 0.25, 4 and 8, respectively; Lanes 5, 10, and 15, PEI-SUC 40%/pDNA at C/P ratios of 0.25, 4, and 8, respectively. Lane 16: DNA ladder.
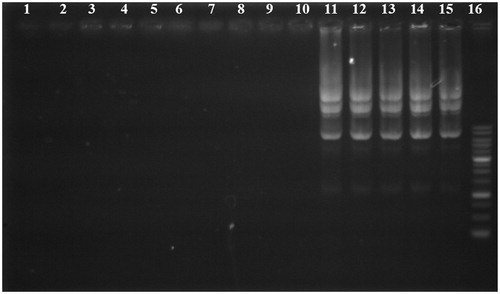
Plasmid protection assay
In order for PEI-SUC derivatives to act as a potent gene carrier, DNA-loaded nanoparticles should be able to protect nucleic acid materials against nucleases and serum enzymes during their transport inside the human body (Hashida et al. Citation1996). The protection ability of PEI-SUC conjugates was tested by DNase I and the integrity of the plasmid was illustrated following the enzymatic treatment by agarose gel electrophoresis. As demonstrated in , all the polyplexes at the lowest C/P ratio (i.e., 0.25) were not able to completely protect the plasmid digestion by DNase I whereas the highest carrier to plasmid ratios could protect the genetic material from enzymatic digestion. In other words, by increasing the polymer concentration the effect of protection against nuclease digestion enhanced. The polyplexes prepared at higher C/P ratios not only were able to fully condense the plasmid DNA () but also could demonstrate the favorable protective effect. However, there are some controversial investigations reporting the different condensation and protection ability of the polyplexes. In these studies, it was shown that full condensation of plasmid DNA does not necessarily lead to complete protection. This behavior could be explained by the fact that some segments of the plasmids could be exposed on the surface of the polyplexes which makes these parts more accessible for further enzymatic degradation, whereas the plasmid sections hindering inside the complexes could be protected from digestion by various nucleases (Hashida et al. Citation1996, Ogris et al. Citation1998). It might be assumed that the administration of the polyplexes even at lower C/P ratios used in this study might lead to plasmid protection against enzymatic degradation due to the lower concentrations of nuclease enzymes in physiological condition in comparison with the DNase I concentration used in this investigation (Mao et al. Citation2001).
Figure 3. DNase I protection assay. Lanes 1–4: PEI-SUC 10% series treated with DNase I at C/P ratios of 0.25, 2, 4, and 8, respectively; Lanes 5–8: PEI-SUC 20% series treated with DNase I at C/P ratios of 0.25, 2, 4, and 8, respectively; Lanes 9–12: PEI-SUC 30% series treated with DNase I at C/P ratios of 0.25, 2, 4, and 8, respectively; Lanes 13–16: PEI-SUC 10% series treated with DNase I at C/P ratios of 0.25, 2, 4, and 8, respectively; Lane 17: DNA size marker.
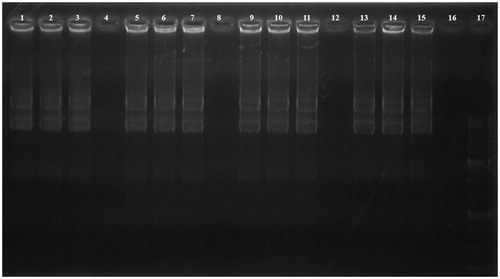
Buffering capacity of the modified PEIs
In order to study the buffering capacity of the unmodified PEI and its conjugates, the substituted PEIs were titrated by an acid/base titration method. Since the conjugates with higher buffering capacity need more amounts of acid to change the pH of their solutions, the titration curves shift to the right. The buffering capacity in the pH range of around 5.5–7 plays a critical role in proton sponge effect which has been considered as the major potential mechanism by which the polyplexes induce the early escape from endo/lysosomal compartments before starting the enzymatic degradation by acid hydrolases (Thomas and Klibanov Citation2002). As illustrated in , no significant difference was observed in the buffering capacity of the unmodified PEI and its derivatives. The buffering capacity of PEI and its derivatives at the pH values higher than 9 might be associated with the primary and secondary amine content of the polymer with the pKa values of 8–9. On the other hand, the buffering capacity of PEI at lower pH values could be attributed to the tertiary amines with the reported pKa values around 6–7 (Oskuee et al. Citation2009, Wang et al. Citation2002). The buffering capacity in such pH acts as a driving force to induce osmotic burst mechanism which leads to the rupture of the endosomal vesicles and releasing the polyplexes into the cytoplasm. Overall, the substantial buffering capacity of the PEI and its substituted forms is the result of the protonation ability of primary, secondary, and tertiary PEI amines at different pH ranges. In the conjugated PEI derivatives, the unreacted primary amines along with the secondary and tertiary ones induce the proton sponge effect and result in the vesicle disruption.
RBC hemolysis experiment
Minimal interaction with blood components is a necessary prerequisite for in vivo gene delivery using polycations. Cell-induced toxicity has been considered as one of the major limiting factors for in vivo application of gene carriers based on polycationic compounds. Polycations including PEI have demonstrated the ability to disrupt cell membrane which in turn leads to cell lysis and death. The effect of unmodified PEI and its derivatives on mammalian cell membranes was evaluated by monitoring the release of hemoglobin from human RBCs. Generally, hemolysis rate below 5% has been considered as an index for tolerable blood compatibility. As shown in , the hemolysis rate for unmodified PEI was higher than 5% at the concentrations ≥0.4 mg/mL. In other words, the parent polymer-induced hemolysis lower than 5% only at the lowest concentration tested (i.e., 0.2 mg/mL). On the other hand, the hemolysis rate of the PEI conjugates decreased as the conjugation degree increased. Unmodified PEI induced 50% of hemolysis at the concentration of 1.4 mg/mL; however, PEI-SUC 30% and PEI-SUC 40% caused less than 40% of hemolysis at the highest concentration used in this investigation (i.e., 2 mg/mL). For future in vivo application of these carriers, PEI-SUC 30% can be used at the C/P ratios up to 15 while the highest allowable C/P ratio for unmodified PEI would be around 5. These results show that high positive charge density on the polymer surface could be responsible for the destructive interaction between the polycations and cell membrane resulting in the loss of the plasma membrane integrity (Forrest et al. Citation2004, Oskuee et al. Citation2009).
Cell-induced toxicity
The cytotoxicity of the PEI-based nanoparticles was measured by MTT assay. Polyplexes were formulated in three different carriers to plasmid ratios (i.e., 0.25, 4, and 8) and their cytotoxic effects were evaluated on HepG2 cell line. As shown in , unmodified PEI-based nanoparticles exhibited the highest toxic effects at the C/P ratio of 8 in which the cell survival reduced to approximately 17%, while the lowest C/P ratio tested (i.e., C/P = 0. 25) resulted in the cell viability of around 85%. The modified PEI derivatives illustrated a similar pattern in which the lowest carrier plasmid ratios induced less toxic effects in comparison with the higher C/P ratios. In other words, there is a concentration-dependent manner in the induction of cytotoxic effects on the cell line. However, the cell-induced toxicity of PEI-SUC 40% was remarkably lower than the rest of the conjugates with lower degrees of substitution. By increasing the conjugation degree, the positive charge density on the polymer surface reduced and resulted in the new conjugates with lower toxic effects. At the C/P ratio of 8, PEI-SUC 40% resulted in the cell viability around 66%, while the PEI-SUC 10% led to the cell survival of approximately 23%. Based on the results obtained in this study, the cytotoxicity of the PEI-SUC 40% conjugates at C/P ratio of 8 is 4-fold lower than unmodified PEI at the same C/P ratio which shows the substantial reduction of the toxic effects in the modified PEIs compared to underivatized polymer. According to this study which is consistent with the previous investigations, the reduction of positive surface charge and/or introduction of negatively charged groups on the polymer surfaces have been considered as an efficient strategy for the reduction of cytotoxicity (Forrest et al. Citation2004, Gabrielson and Pack Citation2006, Nimesh et al. Citation2007, Oskuee et al. Citation2009, Thomas and Klibanov Citation2002).
Figure 6. Cytotoxicity of unmodified 25 kDa PEI and its conjugates complexed with pUMVC3-hIL12 plasmid at C/P ratios of 0.25, 4, and 8 determined in triplicate in HepG2 cells. Metabolic activity was assayed using MTT method and expressed as the percentages of cell viability. *P < 0.05, conjugated PEI compared to unmodified parent polymer at the same C/P ratio.
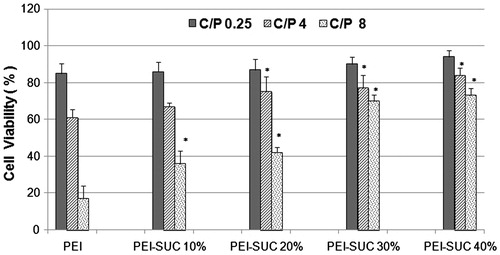
Transfection efficiency
Cytokine-mediated gene therapy is a promising approach for the treatment of diseases such as cancer (Alvarez et al. Citation2014). Among the various interleukins used in cytokine-mediated therapies, IL-12 has been considered as a potent agent due to its antitumor effects (Dass et al. Citation2010). Although the application of recombinant IL-12 protein has shown great potential for the treatment of tumors in clinical trials, major concerns have been raised due to the deaths reported following the systemic injection of the protein (Cohen Citation1995). Multiple high doses of the recombinant IL-12 led to a significant increase in the level of INF-γ followed by severe systemic toxicity. Following this observation, various alternative routes have been examined to reduce the adverse effects of the IL-12 therapy including the intratumoral expression of the plasmid encoding IL-12 gene in order to decrease its systemic toxicity. In this investigation, the ability of modified PEI derivatives was evaluated for the delivery of plasmid encoding IL-12 gene into HepG2 cell line (). The polyplexes were prepared at the same condition as described in the cytotoxicity section and the level of IL-12 expression was normalized and expressed as IL-12 (pg/mL)/seeded cells. The results of the gene delivery experiment obtained in this study indicated that the highest level of IL-12 expression was achieved by PEI-SUC 30% at the C/P ratio of 8 in which the production of IL-12 was 3-fold higher than the parent polymer at the same C/P ratio. The results of the transfection efficiency of PEI-SUC 30% polyplexes at the C/P ratio of 8 prepared by pCMV6-A-GFP plasmid revealed that the gene transfer ability increased to the level of 27.9% of the treated cells. Based on the results of the present investigation, all of the PEI derivatives showed higher gene transfer ability compared to unmodified PEI at the same C/P ratios. At the lowest C/P ratio tested, the IL-12 production level was increased at least 3-fold in comparison with the unmodified polymer (). The impact of hydrophobic modifications on the gene transfer ability of different polycations has been widely discussed in several studies (Dehshahri and Sadeghpour Citation2015). The conjugation of hydrophobic moieties on the surface of polycations facilitates the cell entry of the modified polymers through more efficient interactions with the hydrophobic environment of the plasma membrane. The incorporation of the hydrophobic groups into the PEI structure can alter not only the interaction of modified polymers with plasma membrane, but also reduces the surface positive charge of the polymer which in turn leads to the new conjugates with lower toxicities (Doody et al. Citation2006, Forrest et al. Citation2004, Gabrielson and Pack Citation2006, Nimesh et al. Citation2007, Sabahi et al. Citation2015). As demonstrated in this study, all of the PEI derivatives substituted with succinic anhydride showed lower toxicity. The higher efficiency of modified polymers in transferring the plasmid encoding IL-12 gene could be explained by the fact that various characteristics of the conjugated PEIs have been improved compared to the parent unmodified polymer. For instance, the positive charge density and particle size of the modified polymers were in the favorable ranges which made these conjugated forms of PEI to act as powerful agents for gene delivery. It seems that the optimal properties of PEI-SUC 30% make the carrier as an efficient vehicle for the delivery of IL-12 containing plasmid with low toxicity.
Figure 7. Transfection efficiency of unmodified and modified PEIs complexed with plasmid DNA at polymer:pDNA (weight:weight) ratios of 0.25, 4 and 8 determined in triplicate in HepG2 cell cultures. Cells were treated with polymer/pUMVC3-hIL12 polyplexes and ELISA was carried out to determine the level of hIL-12 production.
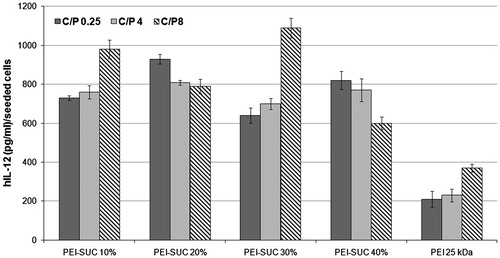
Conclusion
The amine conjugation of PEI with succinic anhydride at four substitution degrees significantly enhanced the efficiency of plasmid DNA delivery. The most probable reasons for the elevated gene transfer ability of PEI are the improved hydrophobic/hydrophilic balance of the polymer and its reduced toxicity.
Funding information
Financial support for this study obtained from Shiraz University of Medical Sciences (SUMS), Shiraz, Iran, for the accomplishment of the Ph.D. thesis of Bahman Khalvati. The results presented in this paper were parts of thesis of Bahman Khalvati.
Disclosure statement
The authors report no declarations of interest. The authors alone are responsible for the content and writing of the paper.
References
- Alvarez RD, Sill MW, Davidson SA, Muller CY, Bender DP, De Bernardo RL, et al. 2014. A phase II trial of intraperitoneal EGEN-001, an IL-12 plasmid formulated with PEG-PEI-cholesterol lipopolymer in the treatment of persistent or recurrent epithelial ovarian, fallopian tube or primary peritoneal cancer: a gynecologic oncology group study. Gynecol Oncol. 133:433–438.
- Boussif O, Lezoualc’h F, Zanta MA, Mergny MD, Scherman D Demeneix B, et al. 1995. A versatile vector for gene and oligonucleotide transfer into cells in culture and in vivo: polyethylenimine. Proc Natl Acad Sci USA. 92:7297–7301.
- Choi KJ, Zhang SN, Choi IK, Kim JS, Yun CO. 2012. Strengthening of antitumor immune memory and prevention of thymic atrophy mediated by adenovirus expressing IL-12 and GM-CSF. Gene Ther. 19:711–723.
- Cohen J. 1995. IL-12 deaths: explanation and a puzzle. Science. 270:908.
- Dass C, Hallaj-Nezhadi S, Lotfipour F. 2010. Nanoparticle-mediated interleukin-12 cancer gene therapy. J Pharm Pharm Sci. 13:472–485.
- Dehshahri A, Oskuee RK, Ramezani M. 2012. Plasmid DNA delivery into hepatocytes using a multifunctional nanocarrier based on sugar-conjugated polyethylenimine. Gene Ther Mol Biol. 14:62–71.
- Dehshahri A, Oskuee RK, Shier WT, Hatefi A, Ramezani M. 2009. Gene transfer efficiency of high primary amine content, hydrophobic, alkyl-oligoamine derivatives of polyethylenimine. Biomaterials. 30:4187–4194.
- Dehshahri A, Sadeghpour H. 2015. Surface decorations of poly(amidoamine) dendrimer by various pendant moieties for improved delivery of nucleic acid materials. Colloids Surf B Biointerfaces. 132:85–102.
- Dehshahri A, Sadeghpour H, Oskuee RK, Fadaei M, Sabahi Z, Alhashemi SH, et al. 2014. Interleukin-12 plasmid DNA delivery using l-thyroxine-conjugated polyethylenimine nanocarriers. J Nanopart Res. 16:1–14.
- Doody AM, Korley JN, Dang KP, Zawaneh P, Putnam D. 2006. Characterizing the structure/function parameter space of hydrocarbon-conjugated branched polyethylenimine for DNA delivery in vitro. J Control Release. 116:227–237.
- Ebrahiminezhad A, Ghasemi Y, Rasoul-Amini S, Barar J, Davaran S. 2013. Preparation of novel magnetic fluorescent nanoparticles using amino acids. Colloids Surf B Biointerfaces. 102:534–539.
- Forrest ML, Meister GE, Koerber J, Pack DW. 2004. Partial acetylation of polyethylenimine enhances in vitro gene delivery. Pharm Res. 21:365–371.
- Gabrielson NP, Pack DW. 2006. Acetylation of polyethylenimine enhances gene delivery via weakened polymer/DNA interactions. Biomacromolecules. 7:2427–2435.
- Hanci D, Sahin E, Muluk N, Cingi C. 2016. Immunotherapy in all aspects. Eur Arch Otorhinolaryngol. 273:1347–1355.
- Hashida M, Mahato RI, Kawabata K, Miyao T, Nishikawa M, Takakura Y. 1996. Pharmacokinetics and targeted delivery of proteins and genes. J Control Release. 41:91–97.
- Kafshdooz T, Kafshdooz L, Akbarzadeh A, Hanifehpour Y, Joo SW. 2016. Applications of nanoparticle systems in gene delivery and gene therapy. Artif Cells Nanomed Biotechnol. 44:581–587.
- Kim J, Wilson DR, Zamboni CG, Green JJ. 2015. Targeted polymeric nanoparticles for cancer gene therapy. J Drug Target. 23:627–641.
- Lee C-H, Ni Y-H, Chen C-C, Chou C-K, Chang F-H. 2003. Synergistic effect of polyethylenimine and cationic liposomes in nucleic acid delivery to human cancer cells. Biochim Biophys Acta. 1611:55–62.
- Li L, He Z-Y, Wei X-W, Gao G-P, Wei Y-Q. 2015. Challenges in CRISPR/CAS9 delivery: potential roles of nonviral vectors. Hum Gene Ther. 26:452–462.
- Malakooty Poor E, Baghaban Eslaminejad M, Gheibi N, Bagheri FA, tyabi F. 2014. Chitosan–pDNA nanoparticle characteristics determine the transfection efficacy of gene delivery to human mesenchymal stem cells. Artif Cells Nanomed Biotechnol. 42:376–384.
- Mao H-Q, Roy K, Troung Le VL, Janes KA, Lin KY, Wang Y, et al. 2001. Chitosan-DNA nanoparticles as gene carriers: synthesis, characterization and transfection efficiency. J Control Release. 70:399–421.
- Mao S, Neu M, Germershaus O, Merkel O, Sitterberg J, Bakowsky U, et al. 2006. Influence of polyethylene glycol chain length on the physicochemical and biological properties of poly(ethylene imine)-graft-poly (ethylene glycol) block copolymer/SiRNA polyplexes. Bioconjug Chem. 17:1209–1218.
- Nezafat N, Sadraeian M, Rahbar MR, Khoshnoud MJ, Mohkam M, Gholami A, et al. 2015. Production of a novel multi-epitope peptide vaccine for cancer immunotherapy in TC-1 tumor-bearing mice. Biologicals. 43:11–17.
- Nimesh S, Aggarwal A, Kumar P, Singh Y, Gupta K, Chandra R. 2007. Influence of acyl chain length on transfection mediated by acylated PEI nanoparticles. Int J Pharm. 337:265–274.
- Ogris M, Steinlein P, Kursa M, Mechtler K, Kircheis R, Wagner E. 1998. The size of DNA/transferrin-PEI complexes is an important factor for gene expression in cultured cells. Gene Ther. 5:1425–1433.
- Orange JS, Salazar-Mather TP, Opal SM, Spencer RL, Miller AH, McEwen BS, et al. 1995. Mechanism of interleukin 12-mediated toxicities during experimental viral infections: role of tumor necrosis factor and glucocorticoids. J Exp Med. 181:901–914.
- Oskuee RK, Dehshahri A, Shier W, Ramezani M. 2008. Modified polyethylenimine: self assemble nanoparticle forming polymer for pDNA delivery. Iran J Basic Med Sci. 11:33–40.
- Oskuee RK, Dehshahri A, Shier W T., Ramezani M. 2009. Alkylcarboxylate grafting to polyethylenimine: a simple approach to producing a DNA nanocarrier with low toxicity. J Gene Med. 11:921–932.
- Parhiz H, Shier WT, Ramezani M. 2013. From rationally designed polymeric and peptidic systems to sophisticated gene delivery nano-vectors. Int J Pharm. 457:237–259.
- Paul A, Eun C-J, Song JM. 2014. Cytotoxicity mechanism of non-viral carriers polyethylenimine and poly-l-lysine using real time high-content cellular assay. Polymer. 55:5178–5188.
- Petros RA, DeSimone JM. 2010. Strategies in the design of nanoparticles for therapeutic applications. Nat Rev Drug Discov. 9:615–627.
- Quezada A, French M, Perrard J, Durland R, Coleman M, Larson J, et al. 2004. Biodistribution and safety studies of hDel-1 plasmid-based gene therapy in mouse and rabbit models. J. Pharm. Pharmacol. 56:177–185.
- Radford KJ, Tullett KM, Lahoud MH. 2014. Dendritic cells and cancer immunotherapy. Curr Opin Immunol. 27:26–32.
- Rezvani Z, Rahimizadeh M, Eshghi H, Dehshahri A, Ramezani M. 2013. The effect of cationic charge density change on transfection efficiency of polyethylenimine. Iran J Basic Med Sci. 16:150–156.
- Sabahi Z, Samani SM, Dehshahri A. 2015. Conjugation of poly (amidoamine) dendrimers with various acrylates for improved delivery of plasmid encoding interleukin-12 gene. J Biomater Appl. 29:941–953.
- Thomas M, Klibanov AM. 2002. Enhancing polyethylenimine’s delivery of plasmid DNA into mammalian cells. Proc Natl Acad Sci USA. 99:14640–14645.
- Wang DA, Narang AS, Kotb M, Gaber AO, Miller DD, Kim SW, et al. 2002. Novel branched poly(ethylenimine)-cholesterol water-soluble lipopolymers for gene delivery. Biomacromolecules. 3:1197–1207.
- Watford WT, Moriguchi M, Morinobu A, O’Shea JJ. 2003. The biology of IL-12: coordinating innate and adaptive immune responses. Cytokine Growth Factor Rev. 14:361–368.
- Zhang Y, Wei H, Xu L, Yan G, Ma C, Yu M, et al. 2016. Preparation and evaluation of a non-viral gene vector for SiRNA: multifunctional envelope-type nano device. Artif Cells Nanomed Biotechnol. 44:1259–1265.
- Zhao L, Wu J, Zhou H, Yuan A, Zhang X, Xu F, et al. 2011. Local gene delivery for cancer therapy. Curr Gene Ther. 11:423–432.
- Zintchenko A, Philipp A, Dehshahri A, Wagner E. 2008. Simple modifications of branched PEI lead to highly efficient siRNA carriers with low toxicity. Bioconjug Chem. 19:1448–1455.