Abstract
Hemorrhage impairs myocardial contractile function and decreases oxygen delivery. This study investigates how polymerized bovine hemoglobin (PolyHb) solutions affect cardiac function after resuscitation from hemorrhagic shock (HS). Hamsters were hemorrhaged and resuscitated with PolyHb at 8.5 g/dL and 11.5 g/dL. Left ventricle (LV) function was assessed during shock and resuscitation using a miniaturize conductance catheter. PolyHb resuscitation had no beneficial effects in cardiac function; it increased cardiac afterload and systemic vascular resistance (SVR) of 46 and 116% for 8.5 and 11.5 g/dL, respectively. Study findings indicate that preclinical evaluation of cardiac function is essential to develop safe and efficacious alternatives to blood transfusion.
Introduction
Hemorrhagic shock (HS) is a condition that results in reduced tissue perfusion and inadequate delivery of oxygen and nutrients to maintain necessary cellular function. Hypovolemic shock, the most common of HS, is characterized by the loss of blood volume (BV) due to trauma or bleeding. The inherent decrease in red blood cells (RBCs) results in a reduction in tissue oxygenation and an increase in anaerobic metabolism. Furthermore, HS can lead to multiorgan dysfunction due to the heart’s inability to pump sufficiently to maintain appropriate blood supply (Bone Citation1996, Horton Citation1989). The first line of treatment to HS is to reestablish BV with the use of plasma expanders (PEs) or blood transfusion. Although, blood transfusion is considered the gold standard approach to treat HS, blood transfusion safety, availability and logistical constrains (e.g., natural disasters and battlefield) have become a concern (Blumberg Citation1997, Stramer et al. Citation2009). The American Red Cross tests for Hepatitis B and C viruses, human immunodeficiency virus (HIV), syphilis, West Nile viruses, and for the agents (Busch et al. Citation2003, Stramer Citation2007, Stramer et al. Citation2009). Nevertheless, there is always the risk that donated blood may contain unidentified infectious agents (Busch et al. Citation2003). As new infectious agents emerge, the cost per unit blood increases (Blumberg Citation1997). In addition, the safety of blood transfusion following extended storage time resulting from storage lesion (Koch et al. Citation2008). Transfusion-related adverse events are among the costliest contributors to health care expenditures (Blumberg Citation1997).
There is great interest on developing safe and efficacious alternatives to blood, including hemoglobin (Hb)-based oxygen carriers (HBOCs) (Stowell et al. Citation2001). The design of HBOCs have evolved from an initial approach involving cell-free Hb, to a more elaborate approach, where Hb is chemically polymerized to increase its molecular size and prevent extravasation (Elmer et al. Citation2011). Despite significant commercial efforts, recent clinical trials with polymerized hemoglobin (PolyHb) solutions hampered further development (Hess Citation1999, Silverman and Weiskopf Citation2009, Sloan et al. Citation1999, Weiskopf Citation2010). The key issues observed during PolyHb clinical trials include vasoconstriction, hypertension, cardiac events, and oxidative stress-induced tissue toxicity (Alayash Citation1999, Weiskopf Citation2010). PolyHb-induced vasoconstriction has been attributed to nitric oxide (NO) scavenging (Alayash Citation1999). Vasoconstriction and hypertension are further exacerbated by Hb extravasation (Kavdia et al. Citation2002, Matheson et al. Citation2002, Tsai et al. Citation2006). As the iron in the heme prosthetic group of Hb increases the formation of reactive oxygen species (ROS). ROS induce tissue toxicity and decrease Hb oxygen carrying capacity via the formation of methemoglobin (Alayash Citation2000, Alayash et al. Citation2001).
Many previous studies demonstrate that PolyHb improved oxygen delivery to tissues (Jahr et al. Citation2008). Our group using intra-vital microscopy during resuscitation form HS with PolyHb has shown that PolyHb improves tissue oxygenation via facilitated oxygen diffusion (Cabrales et al. Citation2009). However, given the observation of transient ischemia, strokes and myocardial infarctions observed in recent clinical trials with PolyHb (Silverman and Weiskopf Citation2009). This study was designed to the hypothesis that the concentration of PolyHb determines cardiac function after resuscitation from HS via affecting the vasoconstriction and systemic vascular resistance (SVR). Tissue blood flow and oxygenation must be restored after resuscitation from HS by recovering an adequate cardiac output (CO), sufficient to maintain perfusion of vital organs and tissues. This study’s additional objective is to confirm that PolyHb can provide the required circulatory support after resuscitation from HS, stroke volume (preload, myocardial contractility, and afterload) and heart rate (HR), without the use of vasoactive agents to improve CO after resuscitation. Two resuscitation fluids were investigated for the purpose of the study: a conventional PE (Dextran 70 kDa) and PolyHb (Hemopure, HBOC-201, OPK Biotech, Boston, MA). PolyHb solution was studied at high concentration, 11.5 g/dL (PolyHb 11.5) and at low concentration, 8.5 g/dL (PolyHb 8.5). Hemopure is compatible with all blood types; blood-type testing and cross-matching is unnecessary. Hemopure has been administered to more than 800 human subjects in 22 completed clinical trials, including blood-controlled trials in elective surgical patients where the product was administered at doses up to 300 g of Hb (10 units) (Pearce et al. Citation2005).
Methods
Animal preparation
Studies were performed in male Golden Syrian hamsters (Charles River Laboratories, Boston, MA) weighing 80–90 g. Animal handling and care followed the NIH Guide for Care and Use of Laboratory Animals. The experimental protocol was approved by the UCSD Institutional Animal Care and Use Committee. Briefly, animals were anesthetized using sodium pentobarbital (40 mg/kg IP). Animal preparation included: (i) left jugular vein and left femoral artery catheterization, (ii) tracheotomy (polyethylene-90 tube), and (iii) left ventricle (LV) conductance catheter introduction through the right carotid artery. Animals were placed in the supine position on a heating pad to maintain core body temperature at 37 °C. Animals were mechanically ventilated (TOPO ventilator, Kent Scientific, Torrington, CT) using room air (respiration rate of 90 breaths/min; peak inspiratory pressure of 20 cmH2O). After instrumentation, volatile anesthesia (0.6%/vol Isoflurane, Drägerwerk AG, Lübeck, Germany) was administered using a vaporizer connected to the ventilator. Depth of anesthesia was continually verified via toe pinch, if needed, isoflurane was increased by 0.1%/vol to prevent animal discomfort. Experimental setup is presented in (Chatpun and Cabrales Citation2010).
Figure 1. Representation of experimental setup. Hamster temperature was maintained at 37 °C. Tracheotomy allows animal mechanical ventilation and administration of anesthetic (isoflurane). Pressure–volume conductance catheter was inserted through carotid artery and advanced into the left ventricle. Femoral artery was catheterized for blood withdrawal, blood sample collection, and pressure measurements. Jugular vein was catheterized for fluid administration. Cardiac function and systemic hemodynamics measurements were using analog to digital acquisition system and stored for off-line analysis.
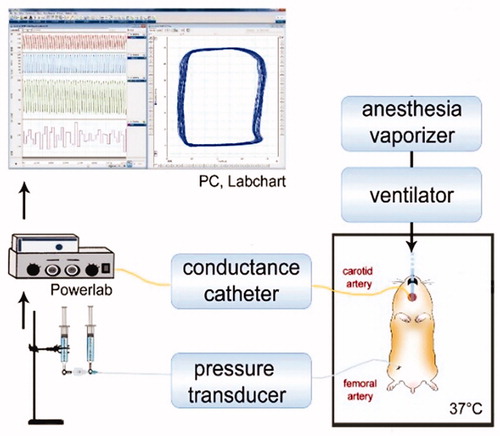
Inclusion criteria
Animals were suitable for the experiments if: (i) mean blood arterial pressure (MAP) > 80 mmHg at baseline, (ii) HR >320 beats/min at baseline, and (iii) systemic hematocrit (Hct) > 45.
Cardiac function
The closed chest method was used to study cardiac function. Briefly, the right common carotid artery was exposed to insert a 1.4F pressure–volume conductance catheter (pressure–volume PV catheter; SPR-839, Millar Instruments, Houston, TX). The PV catheter was advanced passing through the aortic valve into the LV (Pacher et al. Citation2008). The pressure and volume signals were continuously acquired (MPVS300, Millar Instruments, Houston, TX and PowerLab 8/30, AD Instruments, Colorado Springs, CO). Left ventricular volume was measured continuously in conductance units (relative volume unit; RVU) and converted to actual BV (μl) at the end of the experiment (Pacher et al. Citation2008). Parallel volume was calibrated at the end of the experiment via IV injection of 10 μL hypertonic saline (15%) (Pacher et al. Citation2008).
Cardiac pressure–volume indices
Cardiac function was analyzed with PVAN software (Millar Instruments, Houston, TX). Cardiac function parameters were averaged from 10–15 cardiac cycles at each time point. End systolic pressure (Pes) was directly measured. Maximum rate of pressure change (dP/dtmax), minimum rate of pressure change (dP/dtmin), maximum filling volume rate (dV/dtmax), ejection fraction (EF), CO, and stroke work (SW) were calculated. SVR was calculated as SVR = MAP/CO. Oxygen delivery (DO2) was calculated as the product of Hb, CO arteriolar oxygen saturation. In addition, internal energy utilization (IEU) was calculated, to measure the LV metabolism following (Doyle et al. Citation2013).
Hemorrhagic shock resuscitation protocol
After PV catheter insertion, the animal was allowed to rest for 30 min to stabilize before baseline measurements were taken. Anesthetized hamsters were hemorrhaged by withdrawing 30% of the animal’s BV (estimated 7% of body weight) via the femoral artery catheter within 10 min. The hypovolemic shock condition was maintained for 20 min. Resuscitation was implemented by infusion of 100% of the shed volume of test solutions via jugular vein catheter at 100 μL/min. Animals were allowed to recover for 10 min after fluid resuscitation before data collection. MAP and HR were obtained from femoral artery catheter (PowerLab, AD Instruments, Colorado Springs, CO). Hematocrit (Hct) was measured via centrifugation of heparinized capillary tubes. Hemoglobin (Hb) was determined photometrically (Hemocue, Stockholm, Sweden). Arterial blood gases were measured using RapidLab 248 (Bayer, Norwood, MA). Indices of cardiac function and systemic values (MAP, HR, Hct, Hb, and blood gases) were monitored at baseline, during shock, and 10, 20, 30, 45, and 60 min post resuscitation. Animals were euthanized at the end of the experiment. Lack of resuscitation is 100% lethal, although resuscitation yields a recovery on systemic and microvascular parameter.
Test solutions
The PEs evaluated were Dextran 70, 6% Dextran 70 kDa (B. Braun Medical, Irvine, CA); and PolyHb (Hemopure™, HBOC-201 was received as gift from OPK Biotech) at 11.5 g/dL (PolyHb 11.5) and 8.5 g/dL (PolyHb 8.5). Physical properties of blood and resuscitation fluids are presented in .
Table 1. Resuscitation fluid and blood physical properties.
Experimental groups
Animals were randomly assigned into experimental groups based on fluid used during resuscitation, namely: (i) Dextran 70, animals resuscitated with Dextran 70 kDa; (ii) PolyHb 11.5, animals resuscitated with Hemopure at 11.5 g/dL, and (iii) PolyHb 8.5, animals resuscitated with Hemopure at 8.5 g/dL (PolyHb 8.5).
Oxygen affinity, viscosity, colloid oncotic pressure (COP)
Oxygen equilibrium curves were obtained by deoxygenating oxygen equilibrated PolyHb solutions or hamster blood in Hemox buffer at 37 °C, using a Hemox Analyzer (TCS Scientific Corporation, New Hope, PA). Viscosity was measured in a cone and plate viscometer DV-II + (Brookfield Engineering Laboratories, Middleboro, MA). COP was measured using a 4420 membrane colloid osmometer (Wescor, Logan, UT).
Statistical analysis
Results are presented as mean ± standard deviation. The values are presented as absolute values and relative to the baseline. A ratio of 1.0 signifies no change from the baseline, whereas lower or higher ratios are indicative of changes proportionally lower or higher compared to baseline. The Grubbs’ method was used to assess closeness for all measured parameters at baseline and shock. As the data were collected, interim analysis was implemented, and following animal care regulation, no more animals were included as statistical significance was reached. Statistically significant changes between solutions and time points were analyzed using two-way analysis of variance (ANOVA), followed by post hoc analyses using Tukey’s multiple comparisons test when appropriate. All statistics were calculated using GraphPad Prism 6 (GraphPad, San Diego, CA). Results were considered statistically significant if P < .05.
Results
Eighteen animals were included in the study; Dextran 70 (n = 6), PolyHb 8.5 (n = 6), and PolyHb 11.5 (n = 6). All animals tolerated the experimental protocol without signs of stress or discomfort, and passed the Grubbs’ test ensuring that all parameters at baseline and shock were within a similar population (P > .3).
Systemic and blood chemistry
Systemic parameters are presented in . Hemorrhage reduced Hct and Hb, and resuscitation further decreased the Hct. Hb concentration after resuscitation increased in animals resuscitated with PolyHb, proportional to the concentration infused. pH showed no significant differences between groups. Overall, PO2 levels remained higher than physiological levels after hemorrhage and resuscitation. MAP and HR are presented in . Animals resuscitated with PolyHb 11.5 had a recovery significantly higher in MAP at R10 compared to Dextran 70. MAP after resuscitation with PolyHb 11.5 and PolyHb 8.5 were no different. HR decreased after hemorrhage, it never recovered after resuscitation with PolyHb 11.5 ().
Figure 2. Mean arterial pressure and heart rate during the hemorrhagic shock resuscitation protocol. †, P < .05 compared to baseline; ‡, P < .05 compared to Dextran 70; and ¶, P < .05 compared to PolyHb 8.5.
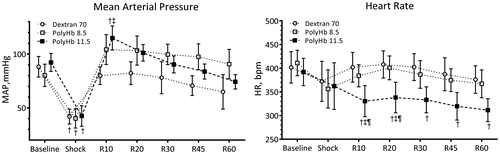
Table 2. Hematocrit, hemoglobin, and blood gases.
Systemic hemodynamics
Normalized to systemic hemodynamic parameters are presented in , and the baseline absolute values are reported in the figure legend. Stroke volume (SV) decreased during shock and gradually recovered after resuscitation in all groups. Resuscitation showed recovered CO compared to HS. CO decreased during shock. Resuscitation recovered CO; however, animals resuscitated with PolyHb 11.5 showed a lower CO at 10 and 20 min after resuscitation compared to resuscitation with Dextran 70 or PolyHb 8.5. Resuscitation with PolyHb increased SVR proportional to PolyHb concentration. Resuscitation with PolyHb 11.5 induced significant hypertension and increased SVR compared to PolyHb 8.5 and Dextran 70, respectively. Resuscitation with Dextran 70 did not increase SVR compared to baseline. Using the measure parameters in the study at baseline, DO2 was 2.2 ± 0.3 mL O2/g.h. DO2 decreased after hemorrhage and resuscitation with PolyHb 8.5 restored DO2 to baseline level. However, resuscitation with PolyHb 11.5 and Dextran 70 did not restore DO2 compared to baseline. Post hemorrhage and during extreme anemia, DO2 fell below the critical DO2, determined by the oxygen consumption (VO2). Whole body VO2 for Golden Syrian hamsters is 1.25 mL O2/g.h (Cabrales et al. Citation2003). This VO2 was satisfied after resuscitation with PolyHb, but Dextran 70 was unable to restore DO2 above the VO2.
Figure 3. Stroke volume (SV), cardiac output (CO), systemic vascular resistance (SVR), and oxygen delivery (DO2) during the hemorrhagic shock resuscitation protocol. Estimated oxygen consumption (VO2) at baseline is presented in the DO2 panel. †, P < .05 compared to baseline; ‡, P < .05 compared to Dextran 70; and ¶, P < .05 compared to PolyHb 8.5.
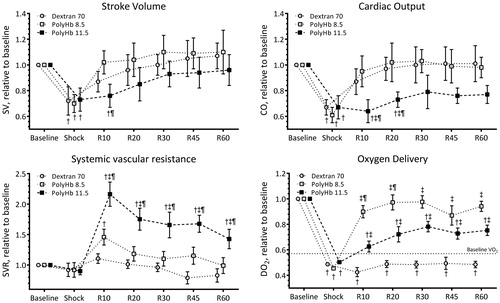
Cardiac function
Normalized to baseline indices of cardiac function (EF, dP/dtmax, dP/dtmin, dV/dtmax, Pes, and Ped) are presented in , and the baseline absolute values are reported in figure legend. dP/dtmax, dP/dtmin, and Pes decreased after the hemorrhage and were recovered by resuscitation. There was no difference on indices of LV function resuscitation with Dextran 70, PolyHb 8.5, or PolyHb 11.5. End systolic pressure, Pes, decreased during shock and resuscitation with PolyHb at 8.5 and 11.5 g/dL, increased Pes by 12 and 35% from baseline, respectively. Energetic indices of LV function (SW and IEU) are presented in . SW decreased during shock, and resuscitation recovered SW to baseline level in all groups. IEU decreased during shock and increased after resuscitation with PolyHb. Resuscitation with PolyHb 11.5 and PolyHb 8.5 increased IEU by 65 and 32% from baseline, respectively.
Figure 4. Indices of left ventricle cardiac function during the hemorrhagic shock resuscitation protocol. Directly measured indices of left ventricle cardiac function were end systolic pressure (Pes) and end diastolic pressure (Ped). Calculated indices of left ventricle cardiac function were ejection fraction (EF), maximum rate of pressure change (dP/dtmax), minimum rate of pressure change (dP/dtmin), and maximum filling volume rate (dV/dtmax). †, P < .05 compared to baseline; ‡, P < .05 compared to Dextran 70; and ¶, P < .05 compared to PolyHb 8.5.
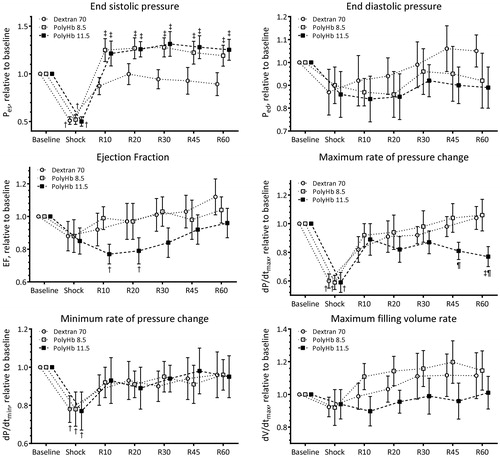
Figure 5. Energetic indices of left ventricle cardiac function during the hemorrhagic shock resuscitation protocol. Calculated external left ventricle cardiac work was estimated using stroke work, and energy metabolism utilization estimated using the internal energy utilization (Doyle et al. Citation2013). †, P < .05 compared to baseline; ‡, P < .05 compared to Dextran 70; and ¶, P < .05 compared to PolyHb 8.5.
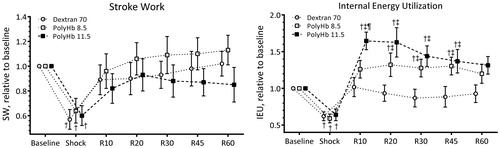
Discussion
The principal finding of this study was that resuscitation from HS with PolyHb restored oxygen carrying capacity, DO2, and MAP, although it increased LV metabolic energy utilization. Resuscitation with PolyHb increased SVR relative to baseline and a PE. Several indices of cardiac function, MAP, and SVR were influenced by PolyHb concentration. Resuscitation with PolyHb expedited the recovery of MAP compared to a PE; although, MAP decreased later on for resuscitation with PolyHb. The rapid recovery of MAP of DO2 could improve the outcome after resuscitation via repaying the HS oxygen debt. Despite adequate fluid resuscitation, only blood transfusion is assumed to improve tissue oxygenation. HS compromises both O2 carrying capacity (Hb concentration) and blood flow, thus the compensatory mechanisms to restore homeostasis to reduce O2 carrying capacity reach their limits in different organs. Our results demonstrate that PolyHb at 8.5 g/dL recovers oxygen delivery to baseline levels and well above the VO2.
The indices of LV cardiac function heavily weighted by stroke volume (such, SV, CO, SW, and EF) after resuscitation from HS were not drastically different between PolyHb and the PE, as the severity of the hemorrhage and the volume infused during the resuscitation are controlled in this HS model. Meanwhile, indices of LV cardiac function more heavily weighted by changes in LV pressure (such as, Pes, SVR, and IEU) showed clear differences between the PolyHb and the PE. While the concept of external LV work performed is well established by the SW, the concept of IEU is less appreciated and not widely used when assessing cardiovascular function. IEU estimated the LV energy metabolism expenditure (Doyle et al. Citation2013). IEU increased after resuscitation with PolyHb 11.5 compared to PolyHb 8.5 and to Dextran 70, respectively. The IEU of both PolyHb concentrations significantly increased form baseline, whereas the IEU after resuscitation with the PE never increased above baseline.
Increasing oxygen transport via resuscitation with PolyHb is expected to increase oxygen supply to tissues, including the heart, and potentially supplying more metabolic energy to the LV. However, the estimated IEU takes into consideration the relation between the LV internal energy dissipation and the LV external work, which is limited by the LV oxygen supply. Thus, for the heart to pump blood after resuscitation with PolyHb is more energetically costly than after resuscitation with a PE. Resuscitation with PolyHb increases the potential energy required to overcome the increased SVR, which prevents the heart of animals resuscitated with PolyHb from obtaining a benefit by the increase in oxygen carrying capacity. In fact, this study shows that the LV of animals resuscitated with PolyHb at 11.5 g/dL increased IEU by 65% above baseline, where SW and DO2 were only restored to baseline level after resuscitation. Post hemorrhage DO2 was below the critical DO2, based on published values and on our previous studies, as Hamster’s VO2 is 1.25 mL O2/g.h. When CO is reduced by HS, DO2 falls below a critically low threshold, when VO2 starts to fall. An abrupt increase in blood lactate concentrations then occurs, indicating the development of anaerobic metabolism. Altogether, these observations suggest a circulatory shock post hemorrhage. Although, resuscitation with Dextran 70 maintained the ration SW/IEU, LV mechano-energetic efficiency, at baseline level, it was unable to increase DO2 above the VO2. Resuscitation with PolyHb 11.5 and 8.5 decreased LV mechano-energetic efficiency to 55 and 80% of baseline, respectively. Resuscitation with PolyHb at 11.5 g/dL caused an increase in SVR of 116% from baseline. The increase in SVR after resuscitation with PolyHb was concentration dependent, as resuscitation with PolyHb at 8.5 g/dL only increased SVR by 46% from baseline. PolyHb at 8.5 g/dL rapidly restored viable cardiovascular function after resuscitation from HS in this model to baseline DO2, independently of the reduced O2 carrying capacity.
Complete mechanistic studies have shown that the cardiac contractile work relation to the myocardial oxygen extraction is preserved over a wide range of altered hemodynamic conditions (Dhainaut et al. Citation1987, Dole and Nuno Citation1986, Doyle et al. Citation2013, Zhang et al. Citation2011). Therefore, resuscitation with PolyHb from HS induced increase on myocardial internal work is greater than the increase in myocardial oxygen extraction provided by PolyHb’s oxygen carrying capacity. In vivo analysis of cardiac function is the only way to study IEU, since in vitro or ex vivo analysis of oxygen consumption relative to pressure–time per minute, force–time per minute, or fiber shortening are not effective marker of energy utilization by the heart (Dole and Nuno Citation1986, Doyle et al. Citation2013). Based on the results obtained in this study, the clinical cardiac complications observed during clinical trials with Hemopure™ and other polymerized Hb oxygen carriers, could be explained by the increased IEU in hearts with limited functional capacity (e.g., individuals with mild to severe cardiac dysfunction, which ventricular and arterial properties are matched up at expense of cardiac health). Thus, PolyHb-induced increase in IEU in hearts with limited functional capacity could foster the occurrence of cardiac events, including myocardial infarction. On the other hand, PolyHb was able to recover adequate DO2, which is essential to preserve organ function, as a low DO2 is a straightforward path to organ failure and death. Overall, the 24-h survival rate of the resuscitation from HS model used in this study is 50% according to our previous studies (Kerger et al. Citation1996). Animals with recovered cardiac function after resuscitation have greater survival rate and good physical condition, judged by the normalcy of consciousness, motor activity, sleeping, and feeding habits, whereas animal unable to recover cardiac function after resuscitation show poor physical appearance (Kerger et al. Citation1996).
The major theory to explain PolyHb-induced increase in SVR, especially at the early after resuscitation, is vasoconstriction caused by PolyHb scavenging of NO (Alayash Citation1999); and/or auto regulatory response to oversupply of O2 oversupply by PolyHb to the vasculature (Cabrales, Sun et al. Citation2009). Small sized PolyHb cannot avoid vasoconstriction, hypertension, and toxicity (Cabrales et al. Citation2009). Hence, increasing PolyHb size can prevent the extravasation by reducing the PolyHb diffusion coefficient, and thus decreasing both the extent of NO scavenging and hyper-oxygenation. Prevention of PolyHb extravasation should also decrease ROS-induced tissue oxidative damage. Our group has shown that PolyHbs with higher molecular weight (>500 kDa) and high cross link density produce less vasoconstriction compared to low molecular weight PolyHbs (Cabrales, Sun et al. Citation2009). As they had a decreased concentration of unpolymerized Hb, increased solution viscosity, and reduced facilitated diffusion relative to low molecular weight PolyHb (Buehler et al. Citation2010, Cabrales, Sun et al. Citation2009). Further research is required to study how PolyHbs with higher molecular weight (>500 kDa) and high cross link density affect cardiac function, as well as the capabilities and limitations of these less vasoactive PolyHb as oxygen carrying therapeutic solutions. Future pre-clinical evaluation of PolyHbs with higher molecular weight and other HBOC should give careful consideration to the analysis of cardiac function in the appropriate animal models to ensure safety and efficacy. Findings of this study may be relevant for clinical application of HBOCs in medicine, suggesting that volume replacement and restitution of adequate O2-carrying capacity with HBOC per se may not be sufficient to restore normal cardiac function, O2 delivery, and metabolic conditions in HS. Although results cannot be translated directly to other vital organs and species, they imply that improved cardiac function could affect long-term survival after resuscitation from HS with PolyHb.
In summary, the results presented here show that cardiac bioenergetics is affected after resuscitation from HS with PolyHb to compensate for the vasoconstriction induced by PolyHb. Although, resuscitation with PolyHb unquestionable could benefit aerobic myocardial metabolism, the increase in heart’s total IEU forces the heart to work harder to compensate for the higher cardiac afterload resulting from the increased SVR. Lastly, resuscitation from HS with PolyHb does not appear to be beneficial compared to resuscitation with a PE, when only 30% of the oxygen carrying capacity is lost during the hemorrhage. This could change after more severe hemorrhages, but requires further investigation. Availability of quantitative tools to study functional changes in cardiac function are crucial to evaluate and understand the PolyHb potential to restore oxygen carrying capacity relative to PolyHb’s side-effects. The monitoring of cardiac function is imperative to define the appropriate conditions where PolyHb, and in general HBOCs, should the utilized.
Ao-ieong_et_al._supplemental_content.pdf
Download PDF (72.9 KB)Acknowledgments
Hemopure™ (HBOC-201) was received as gift from OPK Biotech, Cambridge, MA.
Research was conducted in compliance with the US Animal Welfare Act and other federal statutes and regulations relating to animals and experiments involving animals and adheres to principles stated in the Guide for the Care and Use of Laboratory Animals, NRC Publication, 2011 edition.
Disclosure statement
Authors declare no competing financial interests by the results presented in this manuscript. No financial support was received from OPK Biotech, Cambridge, MA for the completion of the study. OPK Biotech did not participate in the design of the experimental study.
Funding
This work was supported by NIH grants from the Heart Lung and Blood Institute, P01-HL110900, R01-HL052684, R01-HL126945, and R56-HL123015.
References
- Alayash AI, Patel RP, Cashon RE. 2001. Redox reactions of hemoglobin and myoglobin: biological and toxicological implications. Antioxid Redox Signal. 3:313–327.
- Alayash AI. 1999. Hemoglobin-based blood substitutes: oxygen carriers, pressor agents, or oxidants? Nat Biotechnol. 17:545–549.
- Alayash AI. 2000. Hemoglobin-based blood substitutes and the hazards of blood radicals. Free Radic Res. 33:341–348.
- Blumberg N, editor. 1997. Allogeneic transfusion and infection: economic and clinical implications. Semin Hematol. 34: 34–40.
- Bone RC. 1996. Immunologic dissonance: a continuing evolution in our understanding of the systemic inflammatory response syndrome (SIRS) and the multiple organ dysfunction syndrome (MODS). Ann Int Med. 125:680–687.
- Buehler PW, Zhou Y, Cabrales P, Jia Y, Sun G, Harris DR, et al. 2010. Synthesis, biophysical properties and pharmacokinetics of ultrahigh molecular weight tense and relaxed state polymerized bovine hemoglobins. Biomaterials. 31:3723–3735.
- Busch MP, Kleinman SH, Nemo GJ. 2003. Current and emerging infectious risks of blood transfusions. JAMA. 9:959–962.
- Cabrales P, Acero C, Intaglietta M, Tsai AG. 2003. Measurement of the cardiac output in small animals by thermodilution. Microvasc Res. 66:77–82.
- Cabrales P, Sun G, Zhou Y, Harris DR, Tsai AG, Intaglietta M, et al. 2009. Effects of the molecular mass of tense-state polymerized bovine hemoglobin on blood pressure and vasoconstriction. J Appl Physiol (1985). 107:48–58.
- Cabrales P, Tsai AG, Intaglietta M. 2009. Polymerized bovine hemoglobin can improve small-volume resuscitation from hemorrhagic shock in hamsters. Shock. 31:300–307.
- Chatpun S, Cabrales P. 2010. Cardiac mechanoenergetic cost of elevated plasma viscosity after moderate hemodilution. Biorheology. 47:225–237.
- Dhainaut J-FO, Huyghebaert M, Monsallier J, Lefevre G, Dall'Ava-Santucci J, Brunet F, et al. 1987. Coronary hemodynamics and myocardial metabolism of lactate, free fatty acids, glucose, and ketones in patients with septic shock. Circulation. 75:533–541.
- Dole WP, Nuno DW. 1986. Myocardial oxygen tension determines the degree and pressure range of coronary autoregulation. Circ Res. 59:202–215.
- Doyle M, Weinberg N, Pohost GM, Merz CNB, Shaw LJ, Sopko G, et al. 2013. Left ventricular energy model predicts adverse events in women with suspected myocardial ischemia: results from the NHLBI-sponsored women’s ischemia syndrome evaluation (WISE) study. Cardiovasc Diagn Ther. 3:64.
- Elmer J, Cabrales P, Wang Q, Zhang N, Palmer AF. 2011. Synthesis and biophysical properties of polymerized human serum albumin. Biotechnol Prog. 27:290–296.
- Hess CJR. 1999. Blood substitutes for surgery and trauma: efficacy and toxicity issues. BioDrugs. 12:81–90.
- Horton JW. 1989. Hemorrhagic shock depresses myocardial contractile function in the guinea pig. Circ Shock. 28:23–35.
- Jahr JS, Mackenzie C, Pearce LB, Pitman A, Greenburg AG. 2008. HBOC-201 as an alternative to blood transfusion: efficacy and safety evaluation in a multicenter phase III trial in elective orthopedic surgery. J Trauma. 64:1484–1497.
- Kavdia M, Tsoukias NM, Popel AS. 2002. Model of nitric oxide diffusion in an arteriole: impact of hemoglobin-based blood substitutes. Am J Physiol Heart Circ Physiol. 2:H2245–H2253.
- Kerger H, Saltzman D, Menger M, Messmer K, Intaglietta M. 1996. Systemic and subcutaneous microvascular Po2 dissociation during 4-h hemorrhagic shock in conscious hamsters. Am J Physiol. 270:H827–H836.
- Koch CG, Li L, Sessler DI, Figueroa P, Hoeltge GA, Mihaljevic T, et al. 2008. Duration of red-cell storage and complications after cardiac surgery. N Engl J Med. 358:1229–1239.
- Matheson B, Kwansa HE, Bucci E, Rebel A, Koehler RC. 2002. Vascular response to infusions of a nonextravasating hemoglobin polymer. J Appl Physiol (1985). 93:1479–1486.
- Pacher P, Nagayama T, Mukhopadhyay P, Batkai S, Kass DA. 2008. Measurement of cardiac function using pressure-volume conductance catheter technique in mice and rats. Nat Protoc. 3:1422–1434.
- Pearce LB, Gawryl MS, Rentko VT, Moon-Massat PF, Rausch CW. 2005. HBOC-201 (hemoglobin glutamer-250 (bovine), Hemopure®): clinical studies. Blood Substitutes. San Diego (CA): Academic Press, p. 437.
- Silverman TA, Weiskopf RB. 2009. Hemoglobin-based oxygen carriers: current status and future directions. Transfusion. 49:2495–2515.
- Sloan EP, Koenigsberg M, Gens D, Cipolle M, Runge J, Mallory MN, et al. 1999. Diaspirin cross-linked hemoglobin (DCLHb) in the treatment of severe traumatic hemorrhagic shock: a randomized controlled efficacy trial. JAMA. 2:57–64.
- Stowell CP, Levin J, Spiess BD, Winslow RM. 2001. Progress in the development of RBC substitutes. Transfusion. 41:7–299.
- Stramer SL, Hollinger FB, Katz LM, Kleinman S, Metzel PS, Gregory KR, et al. 2009. Emerging infectious disease agents and their potential threat to transfusion safety. Transfusion. 49:1S–29S.
- Stramer SL. 2007. Current risks of transfusion-transmitted agents: a review. Arch Pathol Lab Med. 1:702–707.
- Tsai AG, Cabrales P, Manjula BN, Acharya SA, Winslow RM, Intaglietta M. 2006. Dissociation of local nitric oxide concentration and vasoconstriction in the presence of cell-free hemoglobin oxygen carriers. Blood. 108:3603–3610.
- Weiskopf RB. 2010. Hemoglobin-based oxygen carriers: compassionate use and compassionate clinical trials. Anesth Analg. 110:659–662.
- Zhang C, Rogers PA, Merkus D, Muller‐Delp JM, Tiefenbacher CP, Potter B, et al. 2011. Regulation of coronary microvascular resistance in health and disease. Compr Physiol. 12:521–549.