Abstract
Novel, safe, efficient, and cost effective surfactants from renewable resources has attracted attention for enhancing solubility and bioavailability of hydrophobic dugs. We report the synthesis, characterization, and biocompatibility of a novel non-ionic acyl glycoside double-tailed surfactant for niosomal drug delivery system. Structure of the surfactant was confirmed by 1H NMR and mass spectroscopy. Applications of surfactant in niosomal drug delivery were explored using Cefixime as model. The shape, size, and polydispersity index (PDI) of drug loaded vesicles were investigated with atomic force microscope (AFM) and dynamic light scattering (DLS). Drug entrapping efficiency (EE%) was determined using HPLC. Biocompatibility of the surfactant was evaluated by in vitro cytotoxicity, blood hemolysis, and in vivo acute toxicity. Bioavailability of the surfactant based formulation was investigated in rabbits using HPLC. Vesicles were found to be 159.76 ± 6.54 nm with narrow size distribution and spherical shape. EE% was found to be 71.39 ± 3.52%. Novel surfactant was non-cytotoxicity and hemo-compatible even at 1000 μg/mL concentration and was safe up to 2000 mg/kg body weight. The in vivo bioavailability of niosomal formulation showed elevated plasma concentration and decreased clearance of Cefixime. Current findings reveal that this novel surfactant is biocompatible and could be employed for niosomal drug delivery.
Introduction
Nano-carriers for enhanced in vivo efficacy of many drugs are well established and are widely used both in pharmaceutical research and clinical settings (Estanqueiro et al. Citation2015). Micro or nano range vesicles are novel means of delivering drugs in an engineered and controlled way (Singh et al. Citation2015). Surfactants are the most versatile and frequently used excipients in cosmetic and pharmaceutical products due to their surface or interface activities (Bárány et al. Citation1999, Bragagni et al. Citation2014). They are intended for enhancing bioavailability and therapeutic efficacy of both hydrophilic and hydrophobic drugs over extended period, encapsulated in hydrophilic compartments or outer lipid layers of amphiphiles respectively. Recently, niosomes which are non-ionic surfactants based vesicles are extensively studied for their potential as drug, antigens (Mahale et al. Citation2012), hormones, and other bioactive agents carriers. In addition, they have been used to solve the problem of solubility, stability, and rapid degradation of drugs (Muzzalupo et al. Citation2013, Rajera et al. Citation2011).
Glycoside-based surfactants bear hydrophobic tail and hydrophilic carbohydrate head group (glucose, sucrose, glycoside). They have gained greater attention in drug delivery because of their interesting characteristics like high surface activity, biodegradability, lower toxicity and are derived from renewable sources. Interestingly, glycosidic moieties in such surfactants represent a natural strategy for targeting drugs to diseased sites effectively. Moreover, glycosidic receptors are over-expressed in many tumor cells, whereby glycoside-based drug carriers easily act as tumor targeting ligands. Therefore, they are gifted candidates for replacing the traditional surfactants used in developing pharmaceutical dosage forms (Egan Citation1989, Holmberg Citation2003, Muzzalupo et al. Citation2013).
Bergenin (3,4,8,10-tetrahydroxy-2-hydroxymethyl-9-methoxy-2,3,4,4a-tetrahydropyrano[3,2-c] isochromen-6-one) is a naturally occurring C-glycoside of 4-O-methyl gallic acid (Nazir et al. Citation2007). It has been isolated in bulk from Mallotus japonicas, Mallotus philippinensis, genus Bergenia, and many other plants (Arfan et al. Citation2012, Lim et al. Citation2000). It is neuroprotective (Takahashi et al. Citation2003), hypolipidemic, antitussive, anti-HIV, PTP1B inhibitory, anti-inflammatory, and gastroprotective (Li et al. Citation2005). Its antioxidant activity is well established and its in vitro and in vivo hepatoprotective activity has been reported in various models (Shah et al. Citation2012). Its pharmacological profile adds to its biocompatibility and safety and makes it a strong candidate for the synthesis of glycoside based surfactants for vesicular drug delivery.
Herein, we have synthesized and characterized a novel amphiphilic C-glycoside double-tailed surfactant, “LRC-BG” resulting from the conjugation of lipophilic alkyl chains of lauroyl chloride to phenyl groups of hydrophilic sugar residue of Bergenin as shown in . The resultant surfactant was screened for in vitro and in vivo biocompatibility studies. Niosomal vesicles of the surfactant containing Cefixime were characterized for morphology, size, polydispersity index (PDI), and EE%. Rabbits were used to investigate the in vivo bioavailability and pharmacokinetic parameters of the Cefixime loaded in novel surfactant based niosomal formulation using HPLC quantification method.
Methods and materials
Chemicals and consumables
HPLC grade solvents, 3-(4,5-dimethylthiazol-2-yl)-2,5-diphenyltetrazolium bromide (MTT), Dulbecco's Modified Eagle's medium (DMEM), Bergenin, lauroyl chloride, Polylysine (PLL), Fetal Bovine Serum (FBS), K2CO3, Na2SO4, and Cefixime were all purchased from Sigma-Aldrich, Darmstadt, Germany. Tween 80 was purchased from Merck, Darmstadt, Germany, and cholesterol from BDH, Poole, UK. Oral Cefixime suspension (Maxpan) and capsules (Cefiget) were purchased from Indus and Gitz Pharmas, Karachi, Pakistan.
Synthesis
In a round bottom flask (100 mL) equipped with a magnetic stirrer and a condenser fit on it (Quickfit, Staffordshire, UK), Bergenin (492 mg; 1.5 mM), and K2CO3 (415 mg; 3 mM) were refluxed for 45 min in acetone (15 mL). Then lauroyl chloride (656 mg, 3 mM) was added and progress of reaction was monitored using thin layer chromatography (TLC). Complete conversion of starting material to product was observed after refluxing the reaction mixture for additional 4 h. The reaction was quenched with water (30 mL) and extracted with chloroform (3 × 30 mL). The combined organic phases were concentrated under vacuum and dried over Na2SO4 to give a pure colorless solid with a yield of 90%.
1H NMR (BRUKER AVANCE, AV-400 MHz, USA), DMSO-d6: δ 7.72 (s, 1H, ArH); 4.68 (d, J = 10.4 Hz, 1H, CH); 4.42 (d, J = 12.8 Hz, 1H, CH); 4.34 (d, J = 12.8 Hz, 1H, CH); 4.11 (t, J = 9.2 Hz, 2H, OCH2); 3.94 (d, J = 6.8 Hz, 1H, CH); 3.86 (s, 3H, OCH3); 3.55 (d, 1H, CH); 2.56 (q, 4H, CH2); 2.32 (t, J = 7.6 Hz, 4H, CH2CO); 1.23 (m, 32H, CH2); 0.85 (t, J = 4.8 Hz, 6H, CH3). EIMS (JEOL MS 600H-1, Tokyo, Japan), EI-m/z: 692.4.
Preparation of Cefixime loaded niosomal vesicles
Cefixime-loaded niosomal vesicles of the synthesized surfactant were prepared using thin film hydration method. Surfactant LRC-BG (40 mg) and cholesterol (20 mg) were dissolved in 2:1 w/w ratio in 20 mL mixed solvent system of chloroform and methanol (6:4 v/v). Cefixime (10 mg) was dissolved in 20 mL methanol, and then added to the solution of LRC-BG and cholesterol. The organic solvents were evaporated and the resulting lipid film was dried under reduced pressure using a rotary evaporator (BUCHI, 131 Rotavapor, Flawil, Switzerland). The film was hydrated with 10 mL of BPS (pH 7) at 45 °C for 30 min. The size of the resultant vesicles was reduced by sonication in ultrasonicator (LABSONIC L, B. Braun Biotech International, Allentown, PA, USA) for 4 min at 25 °C with 5 s on/off cycle. The niosomal suspension was stored at 4 °C for further experiments.
Morphology, size, and size distribution of the niosomal formulations
Morphology of drug loaded niosomal vesicles was characterized by atomic force microscope (AFM) (Agilent, 5500, Chandler, AZ). A drop of niosomal vesicles suspension was placed on mica slide. Sample was air-dried at 25 °C and visualized under the microscope. The vesicles shape was observed and imaged in non-contact mode. The mean diameter and PDI of the drug loaded and empty vesicles were determined by dynamic light scattering (DLS) using Zetasizer (Malvern Instruments, Malvern, UK). Vesicles were appropriately diluted in ultrapure water before analysis. Readings were taken in triplicate at scattering angle of 90° at 25 °C immediately after preparation.
Optimization of the chromatographic and plasma drug extraction conditions
For best separation and detection of Cefixime, parameters for HPLC (Shimadzu, LC20A, Kyoto, Japan, with Phenomenex C8 RP column (5 μ; 150 × 4.6 mm) using UV detector) method development were assessed on the basis of factors like peak height, peak width at half height, peak resolution, tailing factor, retention time for Cefixime, and comparison of individual values of the studied parameters. Mobile phase consisting of methanol and 0.4 M H3PO4 in the ratio of 15:85 (v/v) respectively at pH 3 with 1.0 mL/min flow rate at 50 °C temperature of the column were selected for further detection and quantification studies based on best results. The retention time for Cefixime was 13.5 ± 1.5 min. for detection of Cefixime, 288 nm was selected as optimum wavelength. Blank plasma was spiked with working standard solutions of Cefixime to obtain samples in 2–10 μg/mL range and run on HPLC for calibration curve.
Protein precipitation method was followed for drug extraction from plasma. Plasma (200 μL) containing specific concentrations of drug were added 600 μL acetonitrile, vortex-mixed for 1 min, and then centrifuged for 10 min at 10,000 rpm. Supernatant (600 μL) was taken, dried in concentrator, reconstituted in 100 μL mobile phase, and 50 μL was injected into HPLC.
Method validation
Plasma was spiked with Cefixime standard working solutions to have samples in 2–10 μg/mL range for obtaining calibration curves of Cefixime. The calibrators were assayed in triplicate on three different days to evaluate linearity, correlation coefficient, precision, accuracy, recovery, limit of quantification (LOQ), limit of detection (LOD), and stability.
EE% determination
EE% of Cefixime loaded LRC-BG niosomes was determined using HPLC. LRC-BG niosomes (1 mL) containing approximately 1 mg of the drug was taken. Untrapped Cefixime was separated by centrifugation (Universal 16, Hettich, Tuttlingen, Germany) at 12,000 rpm for 25 min. Isolated pellets were washed twice with 5 mL PBS, and centrifuged again at the same speed for the same time. Isolated pellets containing entrapped Cefixime were disrupted with 10 mL methanol, vertexed (Heidolph Reax Top, Schwabach, Germany) for 1 min and then centrifuged for 10 min at 10,000 rpm. A 50 μL aliquot of the resulting solution supernatant was injected into the HPLC using mobile phase of methanol and 0.4 M H3PO4 (15:85 v/v) at pH 3 with 1.0 mL/min flow rate at 50 °C. Cefixime peak was detected at 288 nm with retention time of 13.5 ± 1.5 min.
The drug EE was determined by using the following equation:
In vitro release study
Niosomal vesicles containing approximately 2 mg of Cefixime were taken in phosphate buffer (2 mL, pH 7.4, 6.8, 4, and 1.2 separately), packed into the dialysis membrane with 12,000 kDa, and placed in a bottle (100 mL) containing 50 mL phosphate buffer of respective pH. Bottle was placed in shaker with 100 rpm at 37 °C. Media (2 mL) was withdrawn at specific time intervals (0–24 h). Fresh media was added after each withdrawal to prevent drug saturation. The study was performed for each pH separately. Cefixime released into media was quantified using the UV–vis spectrophotometer (Shimadzu, UV-240, Hitachi U-3200, Kyoto, Japan) at 288 nm.
Evaluation of in vitro cytotoxicity
Cytotoxicity of surfactant LRC-BG surfactant was investigated using MTT assay. Mouse embryonic fibroblast NIH/3T3 cell line was cultured in DMEM containing FBS (10%) and antibiotics (streptomycin and penicillin, each 50 units/mL) in 5% CO2 humidified atmosphere at 37 °C. Cells were seeded into 96-well plates at a density of 8.0 × 103 cells/well in culture medium (200 μL). After 24 h incubation, medium was replaced with fresh medium (200 μL) containing test samples in various concentrations dissolved in culture medium (0.0625–1 mg/mL). For negative control, cells were incubated with media only. Cells were grown for further 24 and 48 h. MTT solution (20 μL; 5 mg/mL) in PBS was added to each well. After 4 h incubation, medium containing the unreacted dye was removed. The obtained purple formazan crystals were dissolved in 200 μL per well dimethyl sulfoxide (DMSO) and the absorbance was measured in microplate reader (ELx808, BioTek, Winooski, VT) at 570 nm. Tween 80 and poly (l) lysine (PLL) were used as reference standard and positive control, respectively. Following formula was used to calculate cells viability for the test samples after 24 and 48 h:
Hemolysis assay
Hemo-compatibility of LC-BG was investigated using human venous blood. Fresh human venous blood was obtained from the Diagnostic Laboratory of Dr. Panjwani Center for Molecular Medicine and Drug Research, International Center for Chemical and Biological Sciences (ICCBS), Pakistan. Blood was centrifuged (700×g for 10 min) to separate the erythrocytes from plasma via sedimentation. The sedimented erythrocyte pellet was washed three times with PBS (pH 7.4), centrifuged (700×g for 10 min) and re-suspended in PBS in 1:10 w/v ratio (erythrocytes:PBS). Test samples (4 mL) were added to the erythrocyte suspension (0.2 mL) in concentrations ranging from 62.5 to 1000 μg/mL. Tween 80 was tested as reference standard. After incubation for 4 h at 37 °C, the samples were centrifuged (700×g for 10 min) to remove the non-lysed erythrocytes. Supernatants were collected and analyzed at 540 nm for the release of hemoglobin using UV spectrophotometer. For 100 and 0% hemolysis, the erythrocyte suspension was added to distilled water and PBS, respectively. The percent hemolysis was determined by the following equation:
where Abs, Abs0, and Abs100 are the absorbance of the test samples, a solution of 0% hemolysis and a solution of 100% hemolysis, respectively (Cenni et al. Citation2008).
Experimental animals
Animal studies adhered to the principles of Institutional Animal Care and Use. The study protocols were approved by the institutional ethical committee for the use of animals in research, International Center for Chemical and Biological Sciences (ICCBS), University of Karachi, Pakistan (ICCBS-027-CEF-2014/Protocol/1.0).
Acute toxicity in vivo
Acute toxicity was carried out in Swiss albino mice (18–25 g) of either sex in two phases. The mice were kept fasting for 12 h. In the first phase, the mice were divided into three groups, each having four mice and were injected intraperitoneally (i.p.) with surfactant LRC-BG at doses of 100, 500, and 1000 mg/kg body weight and observed for mortality. Lethal dose in 50% of objects (LD50) was determined. In second phase, mice were divided into two groups, each having four mice and treated i.p. with LRC-BG at doses of 1500 and 2000 mg/kg body weight. The number of animals died in each group for 24 h was recorded and the mean lethal dose (LD50) was accordingly calculated (Akuodor et al. Citation2013).
In vivo oral pharmacokinetics study
Local species of rabbits (Oryctolagus Cuniculus) were obtained from Dow University of Health Sciences, Karachi, Pakistan. Eighteen male rabbits, weighing 1.6–2.2 kg (1.9 kg average weight) were used. Animals were housed under standard conditions (i.e., at 25 °C, and 12 h day-night cycles having access to food and water). Before study, animals were fasted for 12 h with free access to water only. Animals were divided into three groups each of six. First group was given Cefixime loaded LRC-BG niosomal suspension orally at 6 mg per kg body weight. Animals in second and third groups were given commercially available Cefixime suspension and capsules (powder suspended in 0.5% Tween 80) at 6 mg per kg body weight respectively as reference standards. Blood samples (1 mL each) were collected in heparinized tubes by means of marginal ear vein catheter using insulin plastic syringe at 0, 0.5, 1, 2, 4, 6, 8, 12, 18, and 24 h time intervals. Plasma from blood was separated by centrifuging it at 4000 rpm for 5 min and stored at −80 °C until further analysis. The HPLC validated method was used for quantification of Cefixime in plasma samples. Pharmacokinetic parameters were investigated by the linear trapezoidal rule using non-compartmental model from the individual plasma drug concentration–time curves for Cefixime after the oral administration of its LRC-BG niosomal suspension or the commercial brands. The values of peak height (Cmax) and peak time (Tmax) were obtained directly from the individual plasma drug concentration time curves. Concentration versus time findings were used to calculate various pharmacokinetic parameters like area under the concentration–time curve from zero to the last measurable plasma concentration point (AUC0 − 24), mean residence time (MRT), area under the first moment curve (AUM0–24), and clearance (Cl).
Statistical analysis
Results were expressed as mean ± SEM. All experiments were performed in triplicate. For drug plasma concentrations study, two-way ANOVA followed by Bonferroni’s test was applied for the comparison of niosomal formulation with commercial suspension standard. P values less than or equal to 0.05 were considered statistically significant.
Results and discussion
Characterization
The 1H NMR spectra of the titled compound shows a singlet peak for aromatic proton at δ 7.72 ppm. A triplet peak for terminal methyl protons of the aliphatic side chains is observed at δ 0.85 ppm (6H) and all the other protons of the aliphatic side chains give multiplet peak of 32H at δ 1.23–1.32 ppm. A singlet peak for the methoxy protons is observed at δ 3.86 ppm (). Molecular ion peak in the EI-MS spectrum was observed at m/z 692 which was in agreement with the calculated molecular weight for molecular formula C38H60O11 (). The reaction resulted in 90% yield of the product.
Surface morphology, size, and size distribution drug loaded niosomal formulation
Using DLS, the size of the drug loaded vesicles was found to be 159.76 ± 6.54 nm with 0.32 ± 0.01 PDI. While empty vesicles showed 103.59 ± 3.61 nm mean diameter with 0.38 ± 0.03 PDI as shown in . The size of Cefixime-loaded LRC-BG vesicles was significantly bigger than that of its empty vesicles. Large size of drug loaded vesicles is attributed to increased hydrophobicity of acyl chains of surfactant and lipophilic nature of the drug, resulting in the interaction of drug with surfactant head groups. This in turn increases the mutual repulsion of the surfactant bilayers, causing increased amount of drug to be entrapped and thereby enlarging vesicle size (Essa Citation2010, Schwendener et al. Citation1981). The size of nonionic surfactant vesicles is an important parameter with respect to physical properties and biological fate of them and their entrapped substances. Size of vesicles has also a considerable impact on therapeutic efficiency, toxicity, their phagocytic uptake, drug targeting, and membrane permeability and formulation stability (Šentjurc et al. Citation1999, Shi et al. Citation2006, Verma et al. Citation2003). Vesicle size between 100 and 200 nm is regarded more stable and exhibits better drug retention compared to larger size vesicles (Essa Citation2010). The PDI value shown by our surfactant based niosomes is technically excellent as it falls near 0.3, indicating narrow size distribution and homogeneous formulations (Tavano et al. Citation2013). AFM was used to directly visualize the morphology of Cefixime-loaded LRC-BG vesicles. Smooth sphere morphology was observed for Cefixime-loaded vesicles as shown in . Excellent morphology, nano-range vesicles and narrow PDI of this novel surfactant based niosomal formulation confirm the effective drugs delivery and its prolonged retention in the body.
Table 1. Characteristics of drug loaded LRC-BG niosomes (composition, particle size, PDI, and drug entrapment efficiency).
Method validation
Calibration curves, linearity, accuracy, and precision
The calibration curve for Cefixime was linear in the concentration range of 2–10 μg/mL in plasma. The correlation coefficient of the line was 0.9959 as shown in . The method was quite sensitive for both LOD (1 μg/mL) and LOQ (2 μg/mL). The precision and accuracy of the method were assessed from the low (HQC) (3 μg/mL), medium (MQC) (5 μg/mL), and high (LQC) (8 μg/mL) QC plasma samples. Inter-day assays were determined by analyzing QC samples in triplicate on three different days. Intra-day assays were determined for each QC sample in plasma, each in triplicate on one day. Intra- and inter-day precision in this study was expressed as percent of coefficient of variation (CV). Accuracy was expressed as the mean percentage of analyte recovered in the assay. Inter and intra-day mean accuracy and precision of calibration standards and QCs were well within the acceptance limits as shown in .
Table 2. Intra- and inter-day accuracy and precision of the assay method.
Recovery and stability
%Mean recovery was assessed for three days by measuring the response of the extracted plasma quality control samples at HQC, MQC, and LQC against un-extracted quality control samples at HQC, MQC, and LQC. The %mean recovery for Cefixime was found to be 98.42%, 98.61%, and 96.50% on three successive days. Cefixime freeze–thaw stability was investigated by the analysis of spiked quality control samples for three cycles at −20 °C. The results obtained after the third freeze–thaw cycle were within ±7%, confirming acceptable freeze–thaw stability.
EE% determination
EE% of Cefixime loaded LRC-BG niosomes was determined by HPLC. The calculated average EE% of the niosomes was 71.39 ± 3.52% (). This means that 0.11 mg of Cefixime was entrapped per 1 mg of the lipid phase (LRC-BG and cholesterol). This may be linked to the increased cholesterol content (33% of the total lipid phase) as supported by reported literature that EE% was increased with increasing cholesterol content in niosomes (Attia et al. Citation2007, Yoshioka et al. Citation1994). Cholesterol has the ability to cement the leaking spaces in bilayers membranes, enabling the vesicles to retain maximum amount of drug (Agarwal et al. Citation2001). Structure of surfactant also plays important role in drug EE%. Usually, surfactants with long alkyl or acyl chains show high phase transition temperatures and increased EE% (Hao et al. Citation2002). The aforementioned trend of increased EE% is fulfilled here well by virtue of the long acyl chains attached to both sides of glycosidic moiety. As important parameter, high EE% imparts increased release time characteristic to niosomes (Muzzalupo et al. Citation2013), justifying the efficient use of this novel surfactant as drug carrier.
In vitro release study
In vitro release behavior of Cefixime loaded LRC-BG niosomes was studied at 7.4, 6.8, 4, and 1.2 pH. The formulation released encapsulated drug in a programmed way at different tested pH. Percent release of drug was different for different pH medium showing the effect of the acidity of the medium on drug release from the niosomal formulation. At pH 7.4, the formulation showed extended and increased release pattern, confirming the stability and enhanced bioavailability in in vitro. With increase in acidity (decreasing pH), percent drug release from the formulation decreased as shown in . Interestingly, no abrupt release of drug from the formulation was observed at any pH, confirming the stability of the drug-loaded vesicles (Chen et al. Citation2014). Results show stability of this novel surfactant based formulation at physiological pH of blood.
In vitro cytotoxicity
Cell-based tests are initial step toward systematically biological screening approaches. Besides, in vitro cell culture-based cytotoxicity screening has been reliable mainstay of modern toxicology testing strategies (Krewski et al. Citation2009, Monteiro-Riviere et al. Citation2009). MTT assay for cell viability evaluation has been described suitable method for detection of biomaterial toxicity (Mosmann Citation1983). Surfactant was evaluated for cell cytotoxicity against NIH/3T3 cell line and results are summarized in . Novel surfactant was screened for cytotoxicity at higher doses (0.0625–1.0 mg/mL) so that its use as pharmaceutical excipient could be established. LRC-BG showed cell viability in a dose dependent manner and did not exhibit cytotoxicity even at the highest concentration as compared to reference standard Tween 80. Cell viability of the novel synthesized surfactant was much higher than that of Tween 80, validating its safety profile. The viability was as high as 90.94 ± 2.46% and 88.19 ± 3.35% at 1000 μg/mL concentration after 24 and 48 h respectively as compared to 66.69 ± 3.78% and 38.52 ± 5.93% respectively using Tween 80 solution. The most probable reason of its nontoxic nature can be the saturation in its structure as hypothesized by the previous studies (Pignatello et al. Citation2009). Recently surfactants derived from lysine have been reported with cytotoxicity profile like our surfactant and have been successfully employed as drug carriers (Ménard et al. Citation2012). Moreover, the nonionic nature of the surfactant can also be linked to its lower cytotoxicity (Ullah et al. Citation2016). Cytotoxicity results confirm the nontoxic nature of this novel surfactant and may be used as a safe entity for drug delivery.
In vitro hemolysis assay
Solubility of drugs in aqueous media is one of the prime problems in drug delivery, particularly when they are intended for parenteral use. Surfactants have been widely utilized for this purpose, but due to safety concerns strict restrictions are imposed (Strickley Citation2004) as many amphiphilic substance are known to induce hemolysis of blood (Pethica and Schulman Citation1953). Hemolysis occurs through partitioning of surfactant into the outer cell membrane, followed by formation of surfactant-lipid mixed micelles, resulting in cell leakage. Surfactant structure is believed to play an imperative role in hemolytic activity (Neimert-Andersson et al. Citation2006). Few reports describe hemolytic activities of glycoside based amphiphilic surfactants (Söderlind et al. Citation2003), making them best alternative for pharmaceutical purposes. Hemolysis study of LRC-BG and Tween 80 shows concentration-dependent results. LRC-BG showed 4.53 ± 1.51% hemolytic activities which was quite less than that of the Tween 80 (27.78 ± 3.11%) at same concentration as shown in . LRC-BG behaved well within the permitting limit (Kong et al. Citation2013) under all concentrations (62.5–1000 μg/mL). The results justify that LRC-BG is compatible with blood and may be employed as safe drug carrier.
In vivo acute toxicity
Safety is an important aspect for an innovative drug delivery system. Surfactants have been used to enhance the solubility of drugs in aqueous phase but due to safety concerns; restrictions are imposed (Valdés et al. Citation2014). To ascertain the safety of drug delivery systems, in vivo acute toxicity test in proper animal’s model is of vital importance and validates in vitro toxicity strategies. This test also aims to identify dose that cause serious adverse effects and assesses lowest dose causing lethality. In vivo acute toxicity evaluation of LRC-BG was conducted in mice by i.p. administration and the mice were monitored continuously for 24 h. No lethal dose or median lethal dose (LD50) was achieved even at the highest LRC-BG concentration (2000 mg/kg body weight) as shown in , confirming its safe use.
Table 3. In vivo acute toxicity of LRC-BG in mice.
Oral pharmacokinetic analysis
Average plasma drug concentration time curves after a single oral dose of Cefixime (6 mg/kg) in rabbits as LRC-BG niosomal formulation, commercial capsule and suspension are given in and representative HPLC chromatograms are given in . Pharmacokinetic parameters of Cefixime were calculated from individual curves and mean values are presented in . LRC-BG based Cefixime niosomal formulation showed significantly higher values for Cmax, AUC0–24, MRT, and AUMC0–24 as compared to commercial formulations. No significant difference was found between the values of Tmax for the surfactant based niosomal and commercial formulations of Cefixime. An increase in MRT and AUC0–24 and decrease in Cl indicate sustained release effect of this novel surfactant based niosomal formulation. Most probable approach for this sustained release effect is that niosomes act as slow release vehicle. Niosomes carry drug into the deeper layers of the mucosa through the epithelium, where the entrapped drug is gradually released (Strickley Citation2004). Niosomal formulations of drugs have been reported for increasing their MRT in the body (Parnami et al. Citation2014). Interestingly, increased hydrophobicity of surfactants leads to vesicles stability and reduces their permeability, attributing to drug release from the formulation for longer time (Attia et al. Citation2007). Cmax of LRC-BG niosomal formulation of drug was found to be 10.75 ± 0.85 μg/mL and was compared to those of commercial formulations. These results indicate that about two-fold increase in the oral bioavailability of Cefixime was achieved by the LRC-BG niosomal formulation as compared to commercial formulations. The superior oral bioavailability can also be attributed to the lipophilic nature of the niosomes and the nonionic surfactant effect on the permeability of the gastrointestinal membrane. The unique vesicle size in nano diameter also plays important role in the mechanism of the gastric absorption of drug (Cenni et al. Citation2008, Jain et al. Citation2012). Results of in vivo study reveal that increase in the oral bioavailability and MRT and decrease in Cl was achieved by the niosomal formulation based on our novel surfactant. So, LRC-BG could be used as promising delivery system for lipophilic drugs with sustained release profiles and enhanced bioavailability.
Figure 8. Plasma drug concentrations of Cefixime loaded in LRC-BG, commercial Cefiget (Capsule) and Maxpan (Suspension) at different time intervals, after oral administration at 6 mg/kg body weight dose (n = 6, mean ± SEM). P values less than 0.05 were considered significantly different.

Figure 9. Representative HPLC chromatograms at A: 2 h, B: 6 h, C: 8 h, D: 12 h, E: 18 h time intervals, showing peaks of Cefixime in plasma after its LRC-BG based niosomal formulation.
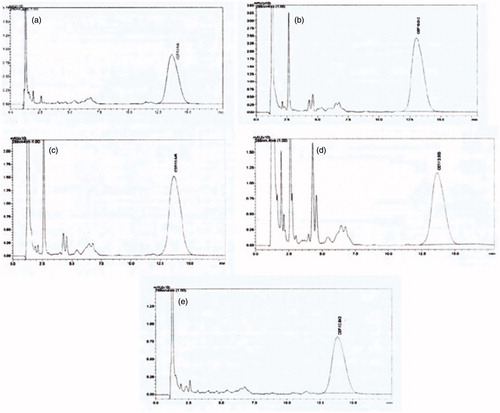
Table 4. Pharmacokinetic parameters of LRC-BG based Cefixime niosomal suspension, commercial brands Maxpan (suspension) and Cefiget (capsules) after their oral administration.
Conclusion
There is increasing demand for non-ionic glycoside surfactants owing to their use in designing niosomal drug delivery systems of poor soluble drugs. This work represents the first report on the synthesis and characterization of acyl glycoside based non-ionic surfactant LRC-BG. Based upon the result of this study, it may be concluded that the novel surfactant was found highly biocompatible and nontoxic. Moreover, EE%, morphology, size, and PDI profiles of its niosomal formulation were also up to the mark. The in vivo release behavior of this novel surfactant based niosomes supports its potential as controlled release delivery system. The results suggest that LRC-BG is safe and effective biomaterial for manufacturing non-ionic surfactant vesicles for delivery of hydrophobic drugs.
Disclosure statement
The authors report no conflicts of interest. The authors alone are responsible for the content and writing of the paper.
Funding
Authors are thankful to Higher Education Commission (HEC), Pakistan for providing PhD scholarship to Muhammad Imran is supported by a PhD scholarship from (PIN No.: 112-23671-2BM1-136).
References
- Agarwal R, Katare O, Vyas S. 2001. Preparation and in vitro evaluation of liposomal/niosomal delivery systems for antipsoriatic drug dithranol. Int J Pharm. 228:43–52.
- Akuodor G, Usman MI, Ibrahim J, Chilaka K, Akpan J, Dzarma S, et al. 2013. Anti-nociceptive, anti-inflammatory and antipyretic effects of the methanolic extract of Bombax buonopozense leaves in rats and mice. Afr J Biotechnol. 10:3191–3196.
- Arfan M, Amin H, Khan I, Shah MR, Shah H, Khan AZ, et al. 2012. Molecular simulations of bergenin as a new urease inhibitor. Med Chem Res. 21:2454–2457.
- Attia IA, El-Gizawy SA, Fouda MA, Donia AM. 2007. Influence of a niosomal formulation on the oral bioavailability of acyclovir in rabbits. AAPS PharmSciTech. 8:206–212.
- Bárány E, Lindberg M, Lodén M. 1999. Biophysical characterization of skin damage and recovery after exposure to different surfactants. Contact Dermatitis. 40:98–103.
- Bragagni M, Mennini N, Furlanetto S, Orlandini S, Ghelardini C, Mura P. 2014. Development and characterization of functionalized niosomes for brain targeting of dynorphin-B. Eur J Pharm Biopharm. 87:73–79.
- Cenni E, Granchi D, Avnet S, Fotia C, Salerno M, Micieli D, et al. 2008. Biocompatibility of poly(d,l-lactide-co-glycolide) nanoparticles conjugated with alendronate. Biomaterials. 29:1400–1411.
- Chen D, Yu H, Mu H, Li G, Shen Y. 2014. Novel multicore niosomes based on double pH-sensitive mixed micelles for Ginsenoside Rh2 delivery. Artif Cells Nanomed Biotechnol. 42:205–209.
- Egan P. 1989. Surfactants from biomass. Chemtech. 19:758–762.
- Essa E. 2010. Effect of formulation and processing variables on the particle size of sorbitan monopalmitate niosomes. Asian J Pharm. 4:227.
- Estanqueiro M, Amaral MH, Conceição J, Lobo JMS. 2015. Nanotechnological carriers for cancer chemotherapy: the state of the art. Colloids Surf B Biointerfaces. 126:631–648.
- Hao Y, Zhao F, Li N, Yang Y, Li K. 2002. Studies on a high encapsulation of colchicine by a niosome system. Int J Pharm. 244:73–80.
- Holmberg K. 2003. Novel Surfactants: Preparation Applications and Biodegradability, Revised and Expanded. Florida, USA: CRC Press.
- Jain S, Valvi PU, Swarnakar NK, Thanki K. 2012. Gelatin coated hybrid lipid nanoparticles for oral delivery of amphotericin B. Mol Pharm. 9:2542–2553.
- Kong M, Park H, Feng C, Hou L, Cheng X, Chen X. 2013. Construction of hyaluronic acid noisome as functional transdermal nanocarrier for tumor therapy. Carbohydr Polym. 94:634–641.
- Krewski D, Andersen ME, Mantus E, Zeise L. 2009. Reply to invited commentaries on toxicity testing in the 21st century: implications for human health risk assessment. Risk Anal. 29:492–497.
- Li Y-F, Hu L-H, Lou F-C, Li J, Shen Q. 2005. PTP1B inhibitors from Ardisia japonica. J Asian Nat Prod Res. 7:13–18.
- Lim H-K, Kim H-S, Chung M-W, Kim YC. 2000. Protective effects of bergenin, the major constituent of Mallotus japonicus, on d-galactosamine-intoxicated rat hepatocytes. J Ethnopharmacol. 70:69–72.
- Mahale N, Thakkar P, Mali R, Walunj D, Chaudhari S. 2012. Niosomes: novel sustained release nonionic stable vesicular systems—an overview. Adv Colloid Interface Sci. 183:46–54.
- Ménard N, Tsapis N, Poirier C, Arnauld T, Moine L, Lefoulon F, et al. 2012. Drug solubilization and in vitro toxicity evaluation of lipoamino acid surfactants. Int J Pharm. 423:312–320.
- Monteiro-Riviere N, Inman A, Zhang L. 2009. Limitations and relative utility of screening assays to assess engineered nanoparticle toxicity in a human cell line. Toxicol Appl Pharmacol. 234:222–235.
- Mosmann T. 1983. Rapid colorimetric assay for cellular growth and survival: application to proliferation and cytotoxicity assays. J Immunol Methods. 65:55–63.
- Muzzalupo R, Tavano L, La Mesa C. 2013. Alkyl glucopyranoside-based niosomes containing methotrexate for pharmaceutical applications: evaluation of physico-chemical and biological properties. Int J Pharm. 458:224–229.
- Nazir N, Koul S, Qurishi MA, Taneja SC, Ahmad SF, Bani S, Qazi GN. 2007. Immunomodulatory effect of bergenin and norbergenin against adjuvant-induced arthritis-a flow cytometric study. J Ethnopharmacol. 112:401–405.
- Neimert-Andersson K, Sauer S, Panknin O, Borg T, Söderlind E, Somfai P. 2006. Synthesis of new sugar-based surfactants and evaluation of their hemolytic activities. J Org Chem. 71:3623–3626.
- Parnami N, Garg T, Rath G, Goyal AK. 2014. Development and characterization of nanocarriers for topical treatment of psoriasis by using combination therapy. Artif Cells Nanomed Biotechnol. 42:406–412.
- Pethica B, Schulman J. 1953. The physical chemistry of haemolysis by surface-active agents. Biochem J. 53:177.
- Pignatello R, Paolino D, Panto V, Pistara V, Calvagno M, Russo D, et al. 2009. Lipoamino acid prodrugs of paclitaxel: synthesis and cytotoxicity evaluation on human anaplastic thyroid carcinoma cells. Curr Cancer Drug Targets. 9:202–213.
- Rajera R, Nagpal K, Singh SK, Mishra DN. 2011. Niosomes: a controlled and novel drug delivery system. Biol Pharm Bull. 34:945–953.
- Schwendener R, Asanger M, Weder H. 1981. n-alkyl-glucosides as detergents for the preparation of highly homogeneous bilayer liposomes of variable sizes (60–240 nm phi) applying defined rates of detergent removal by dialysis. Biochem Biophys Res Commun. 100:1055–1062.
- Šentjurc M, Vrhovnik K, Kristl J. 1999. Liposomes as a topical delivery system: the role of size on transport studied by the EPR imaging method. J Control Release. 59:87–97.
- Shah MR, Arfan M, Amin H, Hussain Z, Qadir MI, Choudhary MI, et al. 2012. Synthesis of new bergenin derivatives as potent inhibitors of inflammatory mediators NO and TNF-α. Bioorg Med Chem Lett. 22:2744–2747.
- Shi B, Fang C, Pei Y. 2006. Stealth PEG-PHDCA niosomes: effects of chain length of PEG and particle size on niosomes surface properties, in vitro drug release, phagocytic uptake, in vivo pharmacokinetics and antitumor activity. J Pharm Sci. 95:1873–1887.
- Singh D, Pradhan M, Nag M, Singh MR. 2015. Vesicular system: versatile carrier for transdermal delivery of bioactives. Artif Cells Nanomed Biotechnol. 43:282–290.
- Söderlind E, Wollbratt M, von Corswant C. 2003. The usefulness of sugar surfactants as solubilizing agents in parenteral formulations. Int J Pharm. 252:61–71.
- Strickley RG. 2004. Solubilizing excipients in oral and injectable formulations. Pharm Res. 21:201–230.
- Takahashi H, Kosaka M, Watanabe Y, Nakade K, Fukuyama Y. 2003. Synthesis and neuroprotective activity of bergenin derivatives with antioxidant activity. Bioorg Med Chem. 11:1781–1788.
- Tavano L, Gentile L, Rossi O, Muzzalupo R. 2013. Novel gel-niosomes formulations as multicomponent systems for transdermal drug delivery. Colloids Surf B Biointerfaces. 110:281–288.
- Ullah S, Shah MR, Shoaib M, Imran M, Elhissi AM, Ahmad F, et al. 2016. Development of a biocompatible creatinine-based niosomal delivery system for enhanced oral bioavailability of clarithromycin. Drug Deliv. 1–12.
- Valdés K, Morill MJ, Romero E, Chávez J. 2014. Physicochemical characterization and cytotoxic studies of nonionic surfactant vesicles using sucrose esters as oral delivery systems. Colloids Surf B Biointerfaces. 117:1–6.
- Verma D, Verma S, Blume G, Fahr A. 2003. Particle size of liposomes influences dermal delivery of substances into skin. Int J Pharm. 258:141–151.
- Yoshioka T, Sternberg B, Florence AT. 1994. Preparation and properties of vesicles (niosomes) of sorbitan monoesters (Span 20, 40, 60 and 80) and a sorbitan triester (Span 85). Int J Pharm. 105:1–6.