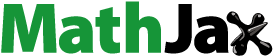
Abstract
Caesalpinia pulcherrima flower extract mediated synthesis of silver nanoparticles was attempted in the present work including optimization of some procedure parameters. Characterization of synthesized silver nanoparticles was done by various spectral analyses. The size of synthesized silver nanoparticles was 12 nm and they were spherical in shape. The green synthesized silver nanoparticles were further evaluated for antimicrobial, antioxidant, cytotoxic, and genotoxic activities; they showed good antimicrobial, antioxidant, and cytotoxic effects. Genotoxic study revealed non-toxic nature at lower concentration. Overall results suggest that the synthesized silver nanoparticles have pronounced applicability in pharmaceutical and biomedical field.
Introduction
Nanotechnology is a novel area of science that combines biology, chemistry, and physics. Nanoparticles possess more surface atoms than microparticles which enhance their functional capabilities. Different types of metals such as Ag, Au, Pt, Zn, Cu, and Pd have been used for the synthesis of nanoparticles. Metal nanoparticles have multifunctional properties and very interesting applications in various fields such as medicine, catalysis, sensors, optoelectronics, food industries, agriculture, and textile industry. Nanoparticles have unique size-dependent crystalline structures (1–100 nm), greater surface to volume ratios, drug encapsulation, surface structure, and biocompatibility due to their material composition. Among the several noble metal nanoparticles, silver nanoparticles (AgNPs) have attained special focus because of their unique distinctive properties, like good chemical stability, surface plasmon resonance properties, and electrical conductivity and they have wide application in pharmaceutical, industrial, and medical applications (Chanda Citation2013).
Antibiotic resistance and various micro-organisms infection has become a major bottleneck in public healthcare sectors. Almost every variant of microorganism has developed resistance to antibiotics mainly due to gene transfer. Another problem is oxidative damage created by free radical generation, is a critical factor implicated in several chronic human diseases such as cancer, atherosclerosis, arthritis, and neurodegenerative diseases. Cancer is one of the most common leading causes of mortality worldwide. Chemotherapy and radiation therapy are available for the treatment of cancer but they are not free from adverse effects. Nanomaterials are expected hopefully to revolutionize and prevent the spread of infections and various cancers.
The nanoparticles can be prepared by physical, chemical, and biological methods. Physical and chemical methods have low yields and the chemical methods cause contamination due to precursor chemicals, the use of toxic solvents, and the generation of hazardous by products (Wang et al. Citation2007). The most preferred method is synthesis using biological entities like bacteria, yeast, fungi, and plants; however, plant extracts mediated synthesis is potentially advantageous over microorganisms due to the ease of scale up, safe, simple, and environment friendly. Plant extracts have a broad variety of metabolites that can be used in the reduction of silver ions, capping and stabilizing agent for the nanoparticles synthesis (Moteriya et al. Citation2016).
On one hand, flowers are cheap, readily available, and are generally used in garlands for social and religious purposes in most of the countries; however, they generate huge amount of waste daily. On the other hand, plant waste is a “biofactory” of different organic compounds such as polyphenols, flavonoids, carotenoids and vitamins. Caesalpinia pulcherrima (CP) is an ornamental plant belonging to Caesalpiniaceae family. Caesalpinia pulcherrima flower is known for is antiviral, antimicrobial, antioxidant, analgesic, anti inflammatory, anthelmintic activities (Chiang et al. Citation2003; Dhaked et al. Citation2011; Patel et al. Citation2010; Pawar et al. Citation2009; Sudhakar et al. Citation2006).
In the present study, a novel, one-step biosynthesis of AgNPs using the flower extract of C. pulcherrima at room temperature is reported perhaps for the first time. Synthesized AgNPs were characterized using various spectral analyses like ultraviolet–visible (UV–Vis) spectroscopy, Fourier Transform Infrared spectroscopy (FTIR), Thermogravimetric analysis (TGA), X-ray diffraction analysis (XRD), and Transmission Electron Microscopy (TEM). In addition, an attempt was made to evaluate its antimicrobial, antioxidant, and anticancer potential by elucidating cytotoxic effects on HeLa cells and genotoxicity on normal human peripheral blood lymphocyte culture.
Materials and methods
Chemicals
The fresh flowers of Caesalpinia pulcherrima (CP) DC were collected from Saurashtra University campus, Rajkot, Gujarat, India. All the chemicals were obtained from Hi Media Laboratories and Sisco Research Laboratories Pvt. Limited, Mumbai, India. Ultra purified water was used for all the experiments.
Preparation of the extract
Fresh flowers of C. pulcherrima were thoroughly washed with tap water, followed by double distilled water, and cut into small pieces. About 5 g of cut flowers were boiled in 100 ml ultra pure water and filtered through Whatmann No. 1 filter paper. The filtrate was cooled to room temperature and used as bioreductant for the synthesis of AgNPs.
Optimization of different parameters
The filtered C. pulcherrima flower extract was used for the synthesis of AgNPs. The synthesis of AgNPs was initially confirmed by UV–Vis absorption spectrophotometer. The reduction of the Ag+ ions in solution was monitored by UV–Vis spectrophotometer (Shimadzu UV-1601, Shimadzu Corporation, Kyoto, Japan) in 350–700 nm range operated at an interval of 10 nm. Influence of various parameters such as boiling time of plant extract (5 min, 10 min, and 15 min), extract amount (1.5 ml, 3 ml, 6 ml, 9 ml, and 12 ml), concentration of silver nitrate (0.5 mM, 1 mM, 1.5 mM, and 2 mM), pH (6–10), and incubating the reaction mixtures with optimum composition (0 min–24 h).
Characterization of the synthesized silver nanoparticles
FTIR analysis
Possible functional groups involved in the synthesis and stabilization of AgNPs was studied by FTIR spectroscopy. The FTIR spectrum was recorded in the range of 400–4000 cm−1 Nicolet IS10 (Thermo Scientific, Waltham, MA). Various modes of vibrations were identified and assigned to determine the different functional groups present in the C. pulcherrima flower extract.
XRD analysis
The structure and composition of synthesizd AgNPs was analyzed by XRD. The formation of AgNPs was determined by an X’Pert Pro X-ray diffractometer (PAN analytical BV) operated at a voltage of 40 kV and a current of 30 mA with Cu Kα radiation in θ–2θ configurations. The crystallite domain size was calculated from the width of the XRD peaks, assuming that they are free from non-uniform strains, using the Scherrer formula. D = 0.94 λ/β cos θ, where D is the average crystallite domain size perpendicular to the reflecting planes, λ is the X-ray wavelength, β is the full width at half maximum (FWHM), and θ is the diffraction angle.
Thermogravimetric analysis
Thermal stability and surface weight loss of AgNPs was analyzed by DTG-60H (Shimadzu Corporation, Kyoto, Japan). Instruments, under a nitrogen gas flow of 100.0 ml min−1 and heating rate of 10 ∘C min−1 to 1000 ∘C.
TEM analysis
TEM analysis was done to visualize the shape as well as to measure the diameter of the biologically synthesized AgNPs. The sample was dispersed in double distilled water. A drop of thin dispersion was placed on a “staining mat”. Carbon-coated copper grid was inserted into the drop with the coated side upwards. After about 10 min, the grid was removed and air dried. Then screened in JEOL JEM 2100 Transmission Electron Microscope (JOEL Corp, Tokyo, Japan).
Antimicrobial activity
Antimicrobial activity of AgNPs was tested by resazurin microtiter (REMA) plate method with some modifications (Palomino et al. Citation2002) against four Gram-positive bacteria (Bacillus cereus, Bacillus subtilis, Staphylococcus aureus, and Corallium rubrum) and four Gram-negative bacteria (Escherichia coli, Pseudomonas aeruginosa, Salmonella typhimurium, and Klebsiella pneumoniae) and three fungi (Candida albicans, Candida glabrata, and Cryptococcus neoformans). The inoculums of the test micro-organism were prepared using the colony suspension method (EUCAST Citation2003). Ninety-six-well culture plates (Tarsons Products Pvt. Ltd., Kolkata, India) were used, and serial two-fold dilutions of the nanoparticles (10–0.019 mg/ml) were dispensed into the plate wells. Two-fold dilutions of Chloramphenicol and Amphotericin B (10–0.019 mg/ml) were used as a positive control. The volume of dispensing nanoparticles was 20 μl per well along with 150 μl of Mueller Hinton Broth (MHB)/Sabouraud Dextrose Broth (SDB) for bacteria and fungi. About 30 μl of microbial culture at a density of 6 × 105 CFU/ml was added to the wells. Three control wells were maintained for each test batch: the positive control (antibiotic, MHB/SDB and test organism), sterility control (MHB/SDB and water), and negative control (MHB/SDB, test organism, and water). The plates were incubated at 37 °C and 28 °C for 24 h and 48 h for bacteria and fungi, respectively. The microbial activity in the test wells was detected by adding 50 μl of 0.01% w/v of Resazurin (HiMedia, Mumbai, India) solution dissolved in sterile distilled water to each well. The plates were incubated for further 2 h, and estimated visually for any change in blue to pink indicating reduction of the dye due to bacterial growth. The lowest concentration (highest dilution) of the nanoparticles required to inhibit visible growth of the tested microorganism was designated as the MIC. The experiment was done in triplicate and the average values were calculated for minimum inhibitory concentration value. Minimum bactericidal concentration (MBC) and minimum fungicidal concentration (MFC) were determined from all wells showing no growth as well as from the lowest concentration showing MIC assay for all the samples. Bacterial or fungal cells from the MIC plates were sub-cultured on freshly prepared solid nutrient agar plates by making streaks on the surface of agar. The plates were incubated at 37 °C and 28 °C for 24 h and 48 h for bacteria and fungi, respectively. Plates that did not show growth were considered to be the MBC/MFC for AgNPs used (Akinyemi et al. Citation2005).
Antioxidant activity
The antioxidant activity of C. pulcherrima flower extract synthesized AgNPs was measured by five in vitro antioxidant assays. The antioxidant assays evaluated were 2,2-diphenyl-1-picrylhydrazyl free radical scavenging assay (DPPH), superoxide anion radical scavenging assay (SO), 2,2′-Azino-bis-(3-ethyl) benzothiazoline-6-sulfonic acid radical cation scavenging assay (ABTS), reducing capacity assessment (RCA), and ferric reducing antioxidant power assay (FRAP). The details of the method followed are as described earlier (Kaneria et al. Citation2012).
In vitro cytotoxic activity
Cancer cell viability of the AgNPs was evaluated by the MTT assay (Labieniec and Gabryelak Citation2003). About 150 μl of HeLa cells were seeded in 96-well plates (Becton Dickinson (BD), Franklin Lakes, NJ) at a density of 1.1 × 104 viable cells/well (HeLa) and incubated 24 h to allow cell attachment. Following attachment, the medium was replaced with complete medium (150 μl/well) containing AgNPs (concentrations ranging from 2 to 200 μg/ml) for 72 h. Following treatment, the cells were washed with PBS and incubated with 100 μl/well fresh medium containing 0.5 mg/ml MTT. The MTT-containing medium was removed after 3 h incubation in dark condition. The MTT formazan was dissolved in 100 μl/well DMSO and the optical density was determined at 570 nm using an ELISA plate reader (Bio-Tek, Winooski, VT). Cell viability was calculated by the following equation:
where As is the absorbance of the cells incubated with the AgNPS and Acontrol is the absorbance of the cells incubated with the culture medium only.
Comet assay (single cell gel electrophoresis)
The genotoxicity of AgNPS was determined using comet assay, based on the measurement of DNA migration under electrophoresis as per slightly modified technique given by Singh et al. (Citation1988). In brief, 180 μl of 1% normal melting point agarose (NMP) was gelled on fully frosted slide (75 × 25 mm) and 100 μl 0.5% low melting point agarose (LMP)-containing cell suspension (20 μL) was layered on the top of the NMP agarose. After formation of cell suspension containing layer, additional 100 μl of LMP agarose was added to fill the residual hole and to form an additional layer to increase the distance between the cells and the gel surface. After agarose gel solidification, the slides were placed in a lysis solution [2.5 M NaCl, 100 mM EDTA and 10 mM Tris HCl (pH 10), plus 1% Triton X-100 and 10% DMSO were added just before use] for 24 h at 4 °C, incubated in an alkaline electrophoresis buffer (300 mM NaOH/1 mM EDTA, pH >13) for 40 min and electrophoresed (Midi-Submarine electrophoresis Unit-7050, Tarsons, Kolkata, India) for 35 min. After the comets were formed, the alkaline gel was neutralized by rinsing the slides with a suitable buffer (0.4 M Tris, pH 7.5) three times. The slides were then stained with the fluorescent dye SYBR Green I (SG) and the comet images were captured under fluorescence microscope. The proportion of DNA damaged was scrutinized by scoring 100 comets for each group (Tri-Tek Comet-Score™ V1.5 software, Tri-Tek, Bremen Germany).
Chromosomal aberration assay
Lymphocyte cultures were setup by Hungerford (Citation1965) with slight modifications. Heparinized whole blood (0.5 ml) was added to mixture containing 5 ml of culture medium RPMI 1640, 0.1 ml phytohemagglutinin (Lectin), and 0.05 ml heparin. Then the culture vials were put in incubator for 72 h, at 37 °C. Doses of AgNPs (2 μg/ml, 50 μg/ml, and 200 μg/ml) positive controls and internal controls were exposed/added at 72th h of incubation. After exposure, vials were put in incubator for 96 h. Then 0.1 ml demecolcine solution was added at last 2 h of incubation period to arrest cells at metaphase. The cells were collected by centrifugation, resuspended in a pre-warmed hypotonic solution (KCl, 0.075 M) for 20–25 min and fixed in chilled methanol/acetic acid (3:1, v/v) solution (Carnoy’s fixative). Suspensions of cells were prepared after several changes of carnoy’s fixative washes. Using the cell suspension slides were prepared and air dried on a hot plate at 50–60 °C. After air dry preparation, slides were stained with 2% Giemsa’s stain for 10 min. From each slide, 100-well spread metaphase chromosomes were scored for chromosome and chromatid type of aberrations.
Results and discussion
Optimization of different parameters
Optimization of various procedure parameters is essential in order to achieve optimum conditions for AgNPs formation. It will yield smaller particles and best activity as also reported by Ibrahim (Citation2015). UV–Vis spectroscopy was used to monitor the formation of nanoparticles during the reaction. When the plant extract is added to silver nitrate solution, there is a color change from pink to dark brown indicating the formation of AgNPs in solution. They exhibit brown color in aqueous media owing to SPR bands of synthesized AgNPs (Moteriya and Chanda Citation2014a). The characteristic absorption maxima are invisible region in the range of 350–700 nm.
It is well known that broader absorption peak indicates larger particle size and narrow absorption peak indicates smaller particle size (Jin et al. Citation2001); while the absorption intensity indicates the number of nanoparticles formed (Ahmad et al. Citation2013). This criterion was used for the selection of correct parameter for the synthesis of AgNPs. The parameters optimized were effect of boiling time of plant extract preparation, plant extract concentration, silver ion concentration, pH of the reaction medium, and time of synthesis reaction. The results are presented in . In general, the absorption peak width and intensity changed when the level of variable changed. A narrow peak with greater intensity was observed when plant extract was boiled for 5 min. Increasing the time of boiling leads to lower and broader absorption peak (). Similar results are reported by Moteriya and Chanda (Citation2014b) for Cassia roxburghii stem-mediated synthesis of AgNPs.
Figure 1. UV–Vis spectroscopy shows the effects of variuos parameters for efficient of AgNps: (A) effect of boiling time, (B) effect of extra amount, (C) effect of silver nitrate concentration, (D) effect of pH, and (E) different incubation times of reaction mixture.
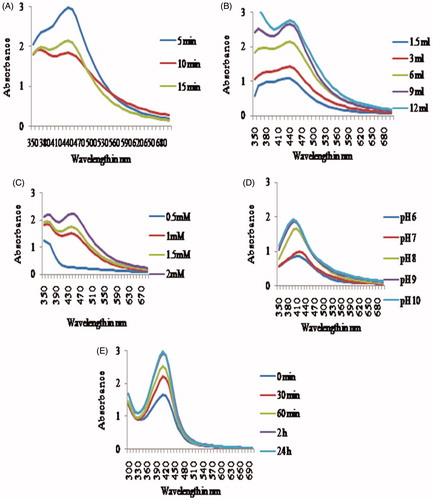
Increasing the amount of plant extract concentration from 1.5 ml to 12 ml, a steady increase in absorption peak was observed after which no change was found (). When the plant extract concentration is increased, the biomolecules involved in the reduction of silver ions to silver nanoparticles are more available which results in more AgNPs formation (Ibrahim Citation2015). Further, Kathiravan et al. (Citation2014) reported that particle size decreased with increased plant extract.
On one hand, the silver ion concentration also had a tremendous effect on AgNPs formation. At 0.5 mM concentration, no particles were formed as evidenced by its absorption peak (no peak was found). On the other hand, at 1 mM silver ion concentration AgNPs formation was rapid and a maximum absorption peak was found at 410 nm (). At higher concentration (1.5 mM and 2.0 mM), the absorption peak broadened indicating larger particle size formation (Valodkar et al. Citation2011). Metal ion concentration also influences the AgNPs formation (Vimala et al. Citation2015).
pH of the reaction medium also affected the AgNPs formation. Both acidic pH (pH 6 and pH 7) induced less number and larger size AgNPs as evidenced by its broader absorption peak. Alkaline pH produced narrow peak with greater intensity but best was with pH 8 (). In acidic pH, the biomolecules responsible for AgNPs formation may be inactivated (Roopan et al. Citation2013). Generally, alkaline pH is utilized for AgNPs formation (Mittal et al. Citation2014).
Increasing the synthesis reaction time from 30 min to 24 h caused a steady increase in the number of AgNPs formed (). After 24 h, no change in absorption peak was observed. In optimal condition, the absorption maxima peak was observed at 410 nm which is due to surface plasmon vibrations. The AgNPs synthesized using C. haematocephala extract showed an absorption peak at 414 nm (De Aragão et al. Citation2016).
Thus, it was evident that all the parameters definitely contributed in AgNPs formation. Therefore, optimum conditions for green synthesis of AgNPs by C. pulcherrima flower extract was 5 min boiling time for flower extract preparation, 12 ml flower extract addition to reaction medium, 1 mM silver nitrate concentration, pH of reaction medium is 8, and reaction time for synthesis of AgNPs is 24 h.
Green synthesis of silver nanoparticles at optimum conditions and characterization
Using the above-optimized conditions, AgNPs were synthesized using C. pulcherrima flower extract and characterization was done using various spectral analyses like FTIR, XRD, TGA, and TEM.
FTIR analysis
FTIR analysis is carried out to identify the possible functional groups responsible for the reduction of silver ions to silver nanoparticles. The FTIR spectrum was recorded in the range of 500–4000 cm−1. FTIR spectrum of AgNPs showed peaks at 2916.37, 2848.86, 1741.72, 1602.85, 1357.89, 1205.51, 1033.85, and 669.30 cm−1 in . The peaks at 2916.37 cm−1 and 2848.86 cm−1 correspond to C–H stretching of the alkanes group and the aldehyde group, respectively. Peak at 1741.72 cm−1 indicates the C = O stretching of the ester group. Peak at 1602.85 cm−1 is due to N–H bend of primary amines. A value of 1357.89 corresponds to N–O symmetric stretch of nitro compounds and 669.30 corresponds to C–H bend of the alkanes group. A value of 1205.51 cm−1 assigned to the C–N stretching of aliphatic amines, 1033.85 cm−1 indicate the C–O stretching of the alcohols group. A value of 1750–1735 cm−1 showed carbonyl C = O stretch of esters also found by Augustine et al. (Citation2014) in P. nigrum leaves biosynthesized AgNPs. Aliphatic amines and alcohols group as also reported by Mittal et al. (Citation2014) in Syzygium cumini fruit extract synthesized AgNPs. Huang et al. (Citation2011) reported that –C–O group was responsible for reductants and protecting agents. The amine group also plays a reducing and controlling role during the formation of AgNPs (Li et al. Citation2007).
X-ray diffraction analysis
The crystalline nature of AgNPs synthesized by C. pulcherrima flower extract was studied by XRD analysis (). It is apparent that the characteristic peaks at 38.10, 44.59, 64.64, and 77.35 in the 2θ values correspond to the lattice planes (111), (200), (220), and (311), respectively (JCPDS no. 4.0783). The strong and narrow diffraction peaks confirmed the crystalline structure of synthesized AgNPs. All the different peaks confirm the face-centered-cubic (fcc) structure of AgNPs. Padalia et al. (Citation2015) and Arthanari et al. (Citation2016) reported similar lattice planes (111), (200), (220), and (311).
Thermogravimetric analysis
The flower extract responsible for the capping action on the surface of biomolecules was further confirmed by TGA (). The first weight loss was observed at 100 °C due to the loss of adsorbed water molecules present in the samples. Also, there is a steady weight loss in temperature range of 0–800 °C. The total weight loss up to 800 °C for AgNPs is about 71.68%. It indicates that a bioorganic compound present in the nanoparticle powder is responsible for the reduction and stabilizing of the particles (Mittal et al. Citation2014).
TEM analysis
TEM is used to determine the morphology, shape, and size of AgNPs. TEM images reveal that the particles are spherical in shape and are uniformly distributed without agglomeration (). The AgNPs size ranges from 2 to 22 nm and possess an average size of 12 nm. Average particle size of 14 nm is reported in Mustard seed synthesized AgNPs (Khatami et al. Citation2015). The selected area electron diffraction (SAED) pattern of AgNPs prepared by C. pulcherrima flower extract suggests the crystalline nature of AgNPs which is in good agreement with the X-ray diffraction (XRD) results ().
Antimicrobial activity
The MIC and the MBC of synthesized AgNPs were performed against four Gram-positive bacteria, four Gram-negative bacteria, and three fungi using different concentrations (0.019–10 mg/ml) by resazurin assay (. Resazurin is a simple method used to detect viable cells in the medium. Different organisms represented different levels of MIC values. The MIC value of Gram-positive bacteria S. aureus and fungi C. glabrata had the lowest MIC value (2.5 mg/ml), B. cereus, E. coli, S. typhimurium, C. albicans, and C. neoformans exhibited slightly higher MIC value (5 mg/ml) and B. subtilis and C. rubrum exhibited the highest MIC value (10 mg/ml). Pseudomonas aeruginosa and K. pneumoniae were resistant strains against AgNPs. MBC/MFC of all the organisms was >10 (mg/ml) except C. glabrata (10 mg/ml). The green synthesized AgNPs exhibited broad spectrum antimicrobial activity but best activity was shown against Gram-positive bacteria and fungi. The most susceptible strains were S. aureus and C. glabrata while resistant strains were P. aeruginosa and K. pneumonia. In accordance with the present results, Barapatre et al. (Citation2016) also reported that S. aureus was most sensitive and P. aeruginosa was resistant to AgNPs biosynthesized by lignin degrading fungus.
Islam et al. (Citation2016) reported greater zone of inhibition against Gram-positive bacteria S. aureus as compared with Gram-negative strains E. coli and P. aeruginosa by P. armeniaca fresh gum extract synthesized AgNPs. According to Ciobanu et al. (Citation2013a) and Ciobanu et al. (Citation2013b), Ag:HAp showed good antibacterial and antibiofilm activity against both Gram-positive and Gram-negative bacteria. Bonilla et al. (Citation2015) found good antifungal activity of AgNPs against fluconazole-resistant Candida species while Groza et al. (Citation2016) found good antibiofilm activity against C. albicans using Ag:HAp-PDMS. In general in the present work, AgNPs inhibited Gram-positive bacteria more than Gram-negative bacteria.
Rajkuberan et al. (Citation2015) reported good antibacterial activity against B. cereus, Enterococci sp, Shigella sp, P. aeruginosa, K. pneumonia, S. aureus, and E. coli. They (AgNPs) generally show antibacterial activity more against Gram-negative bacteria than Gram-positive bacteria (He et al. Citation2013). This differential activity may be due to the difference found in their cell wall composition. The Gram-negative bacteria possess a thin peptidoglycan layer as compared with cell wall of Gram-positive bacteria which is composed of thick peptidoglycan layer which facilitates easier penetration of AgNPs into cell wall of Gram-negative bacteria, while Gram-positive bacterial cell wall forms a tough, formidable barrier for AgNPs penetration. The differential response of synthesized AgNPs against multi-drug-resistant micro-organisms can be attributed to size and shape of AgNPs, secondary metabolites present in the plant extract and also the micro-organism under study. Uddin and Akrema (Citation2016) found similar types of relationship between the size of the nanoparticles and antibacterial activity in C. viminalis leaves-mediated AgNPs. The efficient antimicrobial property AgNPs is because they have extremely large surface area providing better contact with cell wall of micro-organisms (Ibrahim Citation2015). AgNPs interfere with the thiol group of bacterial cell which affects respiratory chain reaction, cell division, and leads to death (Rajkuberan et al. Citation2015). Positively charged silver ions interact with negatively charged bio-macromolecule components (phosphate and disulfide or sulfhydride of enzymes and nucleic acids), which cause structural changes in bacterial cell walls and disruption of the metabolic process followed by cell death (Khan et al. Citation2016). The AgNPs get attached to cell surface of bacteria and alter physical and chemical properties of cell membrane (Marambio-Jones and Hoek Citation2010). This further disturbs membrane permeability, osmoregulation, electron transport, and respiration.
Antioxidant potential
The colorimetric assay of AgNPs synthesized from C. pulcherrima flower extract was done to confirm the antioxidant potential of synthesized AgNPs. The antioxidant activity was tested using DPPH, superoxide anion radical, ABTS cation radical scavenging activity, and reducing capacity assessment. In general, the antioxidant activity increased with an increase in the concentration of AgNPs.
The results of effect of different concentrations of AgNPs on DPPH radical scavenging activity is given in . At concentrations 20–120 μg/ml, AgNPs showed scavenging rate ranging from 15% to 70%. The DPPH radical scavenging activity was evident but it was less than that of standard since the IC50 value of AgNPs was 70 μg/ml while that of standard ascorbic acid was 11.5 μg/ml. Mittal et al. (Citation2014) reported similar results in S. cumimi fruit extract synthesized AgNPs. The IC50 value of synthesized AgNPs was 31 μg/ml while that of standard was 22.19 μg/ml.
Figure 5. Antioxidant activity of AgNPs (A) DPPH-free radical scavenging activity, (B) superoxide anion radical scavenging activity, (C) ABTS radical scavenging activity, and (D) reducing capacity assessment.
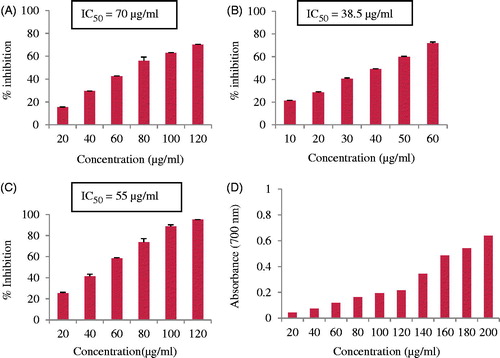
The antioxidant potential was further assessed by their ability to quench superoxide ions by PMS-NBT reduction system using gallic acid as a positive control. The AgNPs showed an increasing trend of quenching super oxide radicals with increase in the concentration of AgNPs (). In fact, at a concentration of 60 μg/ml, 72% inhibition was found. The IC50 value of AgNPs was 38.5 μg/ml while that of standard gallic acid was 185 μg/ml. The super oxide quenching ability of AgNPs was many folds better than even the standard. Mata et al. (Citation2015) reported 52% inhibition at a concentration of 200 μg/ml as compared with standard rutin which exhibited 78% inhibition in Abutilon indicum silver nanoparticles.
The results of effect of different concentration of AgNPs on ABTS cation radical scavenging activity are given in . There was a steady increase in the ABTS cation radical scavenging activity by increasing the concentration of synthesized AgNPs. In the concentration range of 20–120 μg/ml, an inhibition of 23–95% was envisaged. Mittal et al. (Citation2014) found 63% inhibition at 50 μg/ml, while in the present work, it was 57% inhibition is found at a concentration of 60 μg/ml. The reducing capacity of AgNPs showed a steady increase in the absorbance of the reaction mixture with an increase in the concentration of AgNPs (). Similar results are reported by Muniyappan and Nagarajan (Citation2014). In the ferric reducing power assay, the presence of antioxidants in sample would result in the reduction of Fe3+ to Fe2+ and the amount of Fe2+ complex monitored by measuring the formation of blue color at 700 nm. Ferric reducing antioxidant power (FRAP) of AgNPs was 8.8 (Mg−1). FRAP activity of green synthesized AgNPs is also reported by Nayak et al. (Citation2016).
In living organisms, thermal oxidation and reactive oxygen species stimulation oxidation of membrane lipids lead to the formation of super oxide radical, hydroxyl radical, nitrous oxide radical, hydrogen peroxide radical, singlet oxygen radical, etc. which cause many degenerative human diseases such as cancer, glaucoma, atherosclerosis, Alzheimer’s disease, Parkinson’s disease, and diabetes (Barhe and Feuya Tchouya Citation2016). In the present work, the antioxidant activity of C. pulcherrima flower extract-synthesized AgNPs as determined by DPPH-free radical, super oxide anion radical, ABTS cation radical scavenging activity, FRAP, and reducing power assessment suggest that they can be a good source of natural antioxidants and AgNPs have a great potential to scavenge various reactive oxygen species. Among all the free radicals, most dangerous are super oxide radicals since although it itself is weak oxidant, it gives rise to other powerful and dangerous hydroxyl radicals as well as singlet oxygen both of which contribute significantly to oxidative stress and also if they are not scavenged they could damage DNA and protein in living cells. In the present study, among the various antioxidant activities tested, superoxide radical scavenging activity was best by the synthesized AgNPs. Thus, they can be fruitfully used as drug candidates to treat against oxidative stress-related diseases.
Cytotoxicity study
The cell viability assay is one of the important methods for toxicology analysis which explain the cellular response to toxic materials, and it can provide information on cell death, survival, and metabolic activities (Rani et al. Citation2009). The cytotoxicity of the nanoparticles synthesized from C. pulcherrima flower extract on HeLa cells was examined by the MTT assay. The cytotoxic effect of HeLa cell line was dose dependent and cell viability decreased with an increased concentration of Ag NPs. The HeLa cells showed a decrease in the viability of cells with an increase in the concentration of Ag NPs (). The highest inhibition (18%) was found at a concentration of 200 μg/ml of AgNPs, while at 50 μg/ml concentration, 23% cells were viable (). Vasanth et al. (Citation2014) found 6% cell viability at 250 μg/ml concentration, while Li et al. (Citation2015) reported 83.3 ± 3.5% cell viability at 30 μg/ml concentration against HeLa cell line. The cytotoxicity exhibited by AgNPs may be due to physical and chemical interactions of silver ions with functional groups of intracellular proteins, nitrogen bases, and phosphate groups. Gurunathan et al. (Citation2013) suggested that nanoparticles induce generation of reactive oxygen species, activate Caspase 3, induced DNA fragmentation and membrane leakage which results in cell death.
Genotoxicity study
The normal human peripheral blood lymphocyte culture was used for single cell gel electrophoresis (comet assay) and chromosomal aberration for the evaluation of DNA damage assay.
Comet assay
The comet assay is a promising technique which is applied mainly to the study of single-strand DNA breaks induced by a variety of toxic agents, including chemical compounds, ionizing radiation, and nanoparticles in cells (Collins Citation2004). The genotoxicity of AgNPs to damage DNA in the lymphocyte culture was evaluated by alkaline comet assay. DNA damage (according to tail length) in lymphocyte culture exposed to three different concentrations of AgNPs is given in . In the control, halo surrounding cell nuclei was clearly visible (). In the positive control, cells were treated with mitomycin C drug (). Microscopic images confirmed that 2 μg and 50 μg AgNPs-treated cells showed that nuclei were intact and round without any fragmented DNA ( and ). In 200 μg-treated cells, fragmented DNA was found (). The comet length was increased with an increasing concentration of AgNPs (. Out of three different concentrations, a maximum comet length was found at 200 μg concentration, but it was less than positive control. Thus, dose-dependent genotoxicity was found in C. pulcherrima flower-synthesized AgNPs as also reported in MCF-7 cells (Farah et al. Citation2016).
Chromosomal aberration assay
The metaphase chromosomal aberration is given in . The control sample where no AgNPs are added show clean metaphase () while cells treated with mitomycin C drug as a positive control is given in which showed break and interchange in metaphase. The cells treated with three different concentrations of AgNPs (2 μg/ml, 50 μg/ml, and 200 μg/ml) are given in ). Lower concentration of AgNPs treatment showed no appearance of aberrations ( and ) while cells treated with higher concentration of AgNPs showed chromatid gap in chromosome (). Dose-dependant genotoxic effect of AgNPs is also reported by Patlolla et al. (Citation2015).
Conclusion
The use of greener and safer method for synthesis of silver nanoparticles was applied in the present study by using flower extracts of C. pulcherrima. The synthesis of AgNPs depends on pH, reaction time, plant extract, and silver nitrate concentration. The reaction rate is optimum in alkaline pH. The synthesized AgNPs with optimized reaction parameters showed maximum absorption peak at 410 nm. The particle size was 12 nm and was highly crystalline in nature. FTIR analysis revealed as various phytoconstituents of C. pulcherrima flower extract which were responsible for reducing silver ions to silver nanoparticles and also acted as capping agents. TGA analysis revealed its stability. The synthesized AgNPs showed broad spectrum antimicrobial activity with greater efficacy against Gram-positive bacteria and fungi, excellent antioxidant activity, and effective anticancer activity evidenced through MTT assay against HeLa cell line. In vivo genotoxic study demonstrated that AgNPs do not cause any toxic effects at lower concentration. The outcome of this study illustrates a broad range of applications of biosynthesized silver nanoparticles from C. pulcherrima flower extract. Hence, the flower-mediated synthesis of nanoparticles can be used as good therapeutic agent against human pathogens and cancer. They can be used for successful development of drug delivery in future. Therefore, further studies are needed to fully characterize the toxicity and the molecular mechanisms involved in antimicrobial and anticancer activity of these nanoparticles.
Acknowledgements
The authors thank Department of Biosciences (UGC-CAS) for providing excellent research facilities.
Disclosure statement
The authors report no conflicts of interest. The authors alone are responsible for the content and writing of this article.
Funding
One of the authors Ms. Pooja Moteiya is thankful to UGC, New Delhi, for providing meritorious Junior Research Fellowship.
References
- Ahmad T, Wani IA, Manzoor N, Ahmed J, Asiri AM. 2013. Biosynthesis, structural characterization and antimicrobial activity of gold and silver nanoparticles. Colloids Surf B: Biointerfaces. 107:227–234.
- Akinyemi KO, Oladapo O, Okwara CE, Ibe CC, Fasure KA. 2005. Screening of crude extract of six medicinal plants used in south west Nigerian unorthodox medicine for anti-methicillin resistant Staphylococcus aureus activity. BMC Complement Altern Med. 5:6–12.
- Arthanari S, Peng MM, Mani G, Jayabalan J, Mohankumar M, Jang HT. 2016. Low-cost and eco-friendly green synthesis of silver nanoparticles using Prunus japonica (Rosaceae) leaf extract and their antibacterial, antioxidant properties. Artif Cells Nanomed Biotechnol. 9:1–7.
- Augustine R, Kalarikkal N, Thomas S. 2014. A facile and rapid method for the black pepper leaf mediated green synthesis of silver nanoparticles and the antimicrobial study. Appl Nanosci. 4:809–818.
- Barapatre A, Aadil KR, Jha H. 2016. Synergistic antibacterial and antibiofilm activity of silver nanoparticles biosynthesized by lignin-degrading fungus. Bioresour Bioprocess. 3:1–13.
- Barhe TA, Feuya Tchouya GR. 2016. Comparative study of antioxidant activity of the total polyphenols extracted from Hibiscus sabdariffa L., Glycine max L. Merr., yellow tea and red wine through reaction with DPPH free radicals. Arabian J Chem. 9:1–8.
- Bonilla JJA, Guerrero DJP, Sua´rez CIS, Lo´pez CCO, Sa´ez RGT. 2015. In vitro antifungal activity of silver nanoparticles against fluconazole-resistant Candida species. World J Microbiol Biotechnol. 31:1801–1809.
- Chanda S. 2013. Silver nanoparticles (medicinal plants mediated): a new generation of antimicrobials to combat microbial pathogens – a review. In: Mendez-Vilas A, Ed. Microbial Pathogens and Strategies for Combating Them: Science, Technology and Education. Badajoz, Spain: FORMATEX Research Center, pp. 1314–1323.
- Chiang LC, Chiang W, Liu MC, Lin CC. 2003. In vitro antiviral activities of Caesalpinia pulcherrima and its related flavonoids. J Antimicrob Chemother. 52:194–198.
- Ciobanu CS, Iconaru SL, Le Coustumer P, Predoi D. 2013a. Vibrational investigations of silver-doped hydroxyapatite with antibacterial properties. J Spectrosc. http://dx.doi.org/10.1155/2013/471061.
- Ciobanu CS, Iconaru SL, Chifiriuc MC, Costescu A, Le Coustumer P, Predoi D. 2013b. Synthesis and antimicrobial activity of silver-doped hydroxyapatite nanoparticles. BioMed Res Int http://dx.doi.org/10.1155/2013/916218.
- Collins AR. 2004. The comet assay for DNA damage and repair: principles, applications, and limitations. Mol Biotechnol. 26: 249–260.
- De Aragão AP, de Oliveira TM, Quelemes PV, Perfeito MLG, Araújo MC, Santiago JAS, et al. 2016. Green synthesis of silver nanoparticles using the seaweed Gracilaria birdiae and their antibacterial activity. Arabian J Chem. http://dx.doi.org/10.1016/j.arabjc.2016.04.014 (In press).
- Dhaked PS, Panigrahy RN, Kshirsagar SN. 2011. In vitro evaluation of anthelmintic activity of Caesalpinia pulcherrima (Linn) flower extracts in Indian earthworm. Int J Pharm Sci Rev Res. 7:89–91.
- European Committee for Antimicrobial Susceptibility Testing (EUCAST). 2003. Determination of minimum inhibitory concentrations (MICs) of antibacterial agents by broth dilution. Clin Microbiol Inf. 9:1–7.
- Farah MA, Ali MA, Chen SM, Li Y, Al-Hemaid FM, Abou-Tarboush FM, Al-Anazi KM, Lee J. 2016. Silver nanoparticles synthesized from Adenium obesum leaf extract induced DNA damage, apoptosis and autophagy via generation of reactive oxygen species. Colloids Surf B Biointerfaces 141:158–169.
- Groza A, Ciobanu CS, Popa CL, Iconaru SL, Chapon P, Luculescu C, Ganciu M, Predoi D. 2016. Structural properties and antifungal activity against Candida albicans biofilm of different composite layers based on Ag/Zn doped hydroxyapatite-polydimethyl siloxanes. Polymers 8:131.
- Gurunathan S, Raman J, Malek SNA, John PA, Vikineswary S. 2013. Green synthesis of silver nanoparticles using Ganoderma neo-japonicum Imazeki: a potential cytotoxic agent against breast cancer cells. Int J Nanomed. 8:4399–4413.
- He Y, Du Z, Lv H, Jia Q, Tang Z, Zheng X, Zhang K, Zhao F. 2013. Green synthesis of silver nanoparticles by Chrysanthemum morifolium Ramat. extract and their application in clinical ultrasound gel. Int J Nanomed. 8:1809–1815.
- Huang J, Zhan G, Zheng B, Sun D, Lu F, Lin Y, et al. 2011. Biogenic silver nanoparticles by Cacumen platycladi extract: synthesis, formation mechanism and antibacterial activity. Ind Eng Chem Res. 50:9095–9106.
- Hungerford DA. 1965. Leukocytes cultured from small inocula of whole blood and the preparation of metaphase chromosomes by treatment with hypotonic KCl. Stain Technol. 40:333–338.
- Ibrahim HMM. 2015. Green synthesis and characterization of silver nanoparticles using banana peel extract and their antimicrobial activity against representative microorganisms. J Radiat Res Appl Sci. 8:265–275.
- Islam NU, Amin R, Shahid M, Amin M. 2016. Gummy gold and silver nanoparticles of apricot (Prunus armeniaca) confer high stability and biological activity. Arabian J Chem. http://dx.doi.org/10.1016/j.arabjc.2016.02.017 (In press).
- Jin R, Cao CA, Mirkin KL, Kelly GC, Schatz JG, Zheng JG. 2001. Photoinduced conversion of silver nanospheres to nanoprisms. Science 294:1901–1903.
- Kaneria M, Kanani B, Chanda S. 2012. Assessment of effect of hydroalcoholic and decoction methods on extraction of antioxidants from selected Indian medicinal plants. Asian Pac J Trop BioMed. 2:195–202.
- Kathiravan V, Ravi S, Ashokkumar S. 2014. Synthesis of silver nanoparticles from Melia dubia leaf extract and their in vitro anticancer activity. Spectrochim Acta A Mol Biomol Spectrosc. 130:116–121.
- Khan AU, Yuan Q, Wei Y, Khan ZH, Tahir K, Khan SU, et al. 2016. Ultra-efficient photocatalytic deprivation of methylene blue and biological activities of biogenic silver nanoparticles. J Photochem Photobiol B. 159:49–58.
- Khatami M, Nejad MS, Pourseyedi S. 2015. Biogenic synthesis of silver nanoparticles using mustard and its characterization. Int J Nanosci Nanotechnol. 11:281–288.
- Labieniec M, Gabryelak T. 2003. Effects of tannins on Chinese hamster cell line B14. Mutat Res. 539:127–135.
- Li D, Liu Z, Yuan Y, Liu Y, Niu F. 2015. Green synthesis of gallic acid-coated silver nanoparticles with high antimicrobial activity and low cytotoxicity to normal cells. Process Biochem. 50:357–366.
- Li S, Shen Y, Xie A, Yu X, Qiu L, Zhang L, Zhang Q. 2007. Green synthesis of silver nanoparticles using Capsicum annuum L. extracts. Green Chem. 9:852–858.
- Marambio-Jones C, Hoek EMV. 2010. A review of the antibacterial effects of silver nanomaterials and potential implications for human health and the environment. J Nanopart Res. 12:1531–1551.
- Mata R, Nakkala JR, Sadras SR. 2015. Catalytic and biological activities of green silver nanoparticles synthesized from Plumeria alba (frangipani) flower extract. Mat Sci Eng C. 51:216–225.
- Mittal AK, Bhaumik J, Kumar S, Banerjee UC. 2014. Biosynthesis of silver nanoparticles: elucidation of prospective mechanism and therapeutic potential. J Colloid Interface Sci. 415:39–47.
- Moteriya P, Chanda S. 2014a. Biosynthesis of silver nanoparticles using flower extract of Cassia roxburghii DC and its synergistic antibacterial efficacy. Sci Iran. 21:2499–2507.
- Moteriya P, Chanda S. 2014b. Low cost and ecofriendly phytosynthesis of silver nanoparticles using Cassia roxburghii stem extract and its antimicrobial and antioxidant efficacy. Am J Adv Drug Deliv. 2:557–575.
- Moteriya P, Padalia H, Jadeja R, Chanda S. 2016. Review: screening of silver nanoparticle synthetic efficacy of some medicinal plants of Saurashtra region. In: Gupta VK, Ed. Natural Products: Research Review, vol. 3. New Delhi: Daya Publishing House, pp. 63–83.
- Muniyappan N, Nagarajan NS. 2014. Green synthesis of silver nanoparticles with Dalbergia spinosa leaves and their applications in biological and catalytic activities. Process Biochem. 49:1054–1061.
- Nayak D, Minz AP, Ashe S, Rauta PR, Kumari M, chopra P, Nayak B. 2016. Synergistic combination of antioxidants, silver nanoparticles and chitosan in a nanoparticle based formulation: characterization and cytotoxic effect on MCF-7 breast cancer cell lines. J Colloid Interface Sci. 470:142–152.
- Padalia H, Moteriya P, Chanda S. 2015. Green synthesis of silver nanoparticles from marigold flower and its synergistic antimicrobial potential. Arabian J Chem. 8:732–741.
- Palomino JC, Martin A, Camacho M, Guerra H, Swings J, Portaels F. 2002. Resazurin microtiter assay plate: simple and inexpensive method for detection of drug resistance in Mycobacterium tuberculosis. Antimicrob Agents Chemother. 46:2720–2722.
- Patel SS, Verma NK, Chatterjee C, Gauthaman K. 2010. Screening of Caesalpinia pulcherrima Linn. flowers for analgesic and anti-inflammatory activities. Int J Appl Res Nat Prod. 3:1–5.
- Patlolla AK, Hackett D, Tchounwou PB. 2015. Genotoxicity study of silver nanoparticles in bone marrow cells of Sprague–Dawley rats. Food Chem Toxicol. 85:52–60.
- Pawar CR, Mutha RE, Landge AD, Jadhav RB, Surana SJ. 2009. Antioxidant and cytotoxic activities of Caesalpinia pulcherrima wood. Indian J Biochem Biophys. 46:198–200.
- Rajkuberan C, Sudha K, Sathishkumar G, Sivaramakrishnan S. 2015. Antibacterial and cytotoxic potential of silver nanoparticles synthesized using latex of Calotropis gigantea L. Spectrochim Acta Part a Mol Biomol Spectrosc. 136:924–930.
- Rani PVA, Mun GLK, Hande MP, Valiyaveettil S. 2009. Cytotoxicity and genotoxicity of silver nanoparticles in human cells. ACS Nano 3:279–290.
- Roopan SM, Rohit Madhumitha G, Rahuman AA, Kamaraj C, Bharathi A, Surendra TV. 2013. Low-cost and ecofriendly phyto-synthesis of silver nanoparticles using Cocos nucifera coir extract and its larvicidal activity. Ind Crops Prod. 43:631–635.
- Singh NP, McCoy MT, Tice RR, Schneider EL. 1988. A simple technique for quantitation of low levels of damage in individual cells. Exp Cell Res. 175:184–191.
- Sudhakar M, Rao CV, Rao PM, Raju DB, Venkateswarlu Y. 2006. Antimicrobial activity of Caesalpinia pulcherrima, Euphorbia hirta and Asystasia gangeticum. Fitoterapia 77:378–380.
- Uddin R. Akrema 2016. Extracellular synthesis of silver dimer nanoparticles using Callistemon viminalis (bottlebrush) extract and evaluation of their antibacterial activity. Spectrosc Lett. 49:268–275.
- Valodkar M, Nagar PS, Jadeja RN, Thounaojam MC, Devkar RV, Thakore S. 2011. Euphorbiaceae latex induced green synthesis of non-cytotoxic metallic nanoparticle solutions: a rational approach to antimicrobial applications. Colloids Surf a: Physicochem Eng Asp. 384:337–344.
- Vasanth K, Ilango K, Mohan Kumar R, Agrawal A, Dubey GP. 2014. Anticancer activity of Moringa oleifera mediated silver nanoparticles on human cervical carcinoma cells by apoptosis induction. Colloids Surf B: Biointerfaces. 117:354–359.
- Vimala RTV, Sathishkumar G, Sivaramakrishnan S. 2015. Optimization of reaction conditions to fabricate nano-silver using Couroupita guianensis Aubl. (leaf & fruit) and its enhanced larvicidal effect. Spectrochim Acta Part a Mol Biomol Spectrosc. 135:110–115.
- Wang Z, Chen J, Yang P, Yang W. 2007. Biomimetic synthesis of gold nanoparticles and their aggregates using a polypeptide sequence. Appl Organometal Chem. 21:645–651.