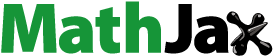
Abstract
The present study aimed to develop an improved oral delivery system for low-molecular-weight heparin (LMWH), novel polymer lipid hybrid nanoparticles were developed. LMWH loaded chitosan polymer lipid hybrid nanoparticles (LMWH-CS-PLNs) were developed using double emulsification and solvent evaporation method. The performance of developed formulations was evaluated by using in vitro and in vivo behavior, such as drug release studies, in vitro permeation study, in vivo venous thrombolytic study, in vitro uptake studies by using intestinal epithelium resembling Caco-2 cell lines. The new CS-PLNs might provide an effective strategy for oral delivery of LMWH with improved encapsulation efficiency as compared to CS-NPs and SA-LNPs.
Introduction
Low-molecular-weight heparins (LMWHs) are antithrombotic therapeutic moieties approved in various countries for clinical indications including venous thromboembolism (VTE) prophylaxis and treatment and acute coronary syndromes (ACS) (Anderson et al. Citation2007, Antman et al. Citation2004, Geerts et al. Citation2008, Kearon et al. Citation2008). Acute DVT, which has the symptom of calf pain and swelling frequently goes unseen until symptoms build up, and approximately 100,000 patients in the United States die of a DVT induced pulmonary embolism annually (Breddin et al. Citation2001, Lensing et al. Citation1999). LMWHs are of high molecular weight, negative surface charge, hydrophilic nature with short half-lives. These are administered by subcutaneous injections at various times a day because they are not competently absorbed through the gastrointestinal tract when taken orally (Kim et al. Citation2007b). The presence of carboxylic acid and sulfate groups in the glycosaminoglycan units of LMWH makes it a highly anionic molecule (Yang et al. Citation2012). Nowadays, LMWH that does not essentially require patient hospitalization is replacing unfractionated heparin (UFH) and warfarin is being used as an alternative in many countries, thus reducing the expenses for health care (Hull et al. Citation2004). Moreover, LMWH have many other benefits over UFH including a superior expectedness of the pharmacodynamic effect and a lesser risk of heparin-induced thrombocytopenia (Liautard et al. Citation2002). Therefore, there is a huge obligation for the development of an oral LMWH formulation (Hoffart et al. Citation2006). Various strategies have been explored by researchers including use of penetration enhancers (Hayes et al. Citation2006), microparticles (Javot et al. Citation2009), polymeric nanoparticles (Chen et al. Citation2009), dendrimeric nanocarriers (Bai and Ahsan Citation2009), chemical conjugates (Kim et al. Citation2007a), biomimetic solid lipid nanoparticles (Paliwal et al. Citation2011), alginate coated chitosan core shell nanoparticles (Bagre et al. Citation2013), nanoparticles in enterically coated tablets containing permeation enhancers (Goharzadeh et al.), nanocapsules as nanocarriers (Ramadan et al. Citation2011), etc. However, till date, no oral formulation of heparin exists in the market (Hwang et al. Citation2012, Kim et al. Citation2011). Nevertheless, the intestinal epithelium is a chief obstruction to the absorption of hydrophilic macromolecules like heparin because it cannot diffuse across the cells through the lipid-bilayer cell membranes, due to their high molecular weight and hydrophilicity (Hull et al. Citation2004, Kearon et al. Citation2008). A few technologies, based on using carrier molecules such as deoxycholic acid (DOCA) have been recently projected to overcome this restriction (Liautard et al. Citation2002). Studies have revealed that these hydrophobic carriers facilitate the systemic absorption of heparin via transcellular delivery and their oral bioavailability has been increased to 16.6% (Breddin et al. Citation2001, Lensing et al. Citation1999). However, chemical modification of heparin with DOCA has resulted in a significant decrease in its relative bioactivity, compared to the raw heparin (Paliwal et al. Citation2009).
Therefore, in the present study, the use of both cationic polymer and lipid was proposed. Lipid polymer hybrid system has been explored for the oral delivery of several thrombolytic agents. Previous reports demonstrated that chitosan and lipid nanoparticles increased the oral bioavailability of heparin. Lipid based carriers have also been reported to enhance the oral delivery of biomacromolecules to systemic circulation through intestinal lymphatic system and by evading first pass metabolism by the liver (Hallan et al. Citation2016, Vyas et al. Citation2008). Therefore, chylomicron being hydrophobic in nature, the largest (75–600 nm) lipid-carrying entity in the lymph, is too bulky to enter unswervingly into the blood capillaries of the mucosa and hence is transported via the lymph. Consequently, enclosing hydrophilic drugs in lipid particulates may promote their transport via the lymphatic system on absorption due to the size of the particulate carrier. Thus, the single polymer lipid hybrid nanoparticles can be used for two different purposes.
Hence, in the present research, it has been investigated that by using cationic polymer, loading efficiency of heparin in lipid carrier could be increased. Further, chitosan itself has been reported to increase the transport of heparin through intestinal membrane by opening tight junctions. Here, use of chitosan as a component of hybrid system will not only increase the entrapment efficiency of lipid nanoparticle but also synergies the transport of heparin across the intestinal membrane with improved bioavailability.
To validate the efficacy of hybrid nanoparticles, the polymeric (chitosan) nanoparticles and cationic lipid (stearyl amine) were developed. The three optimized particles were compared on the basis of their physicochemical characteristics (including particle surface morphology, particle size and zeta potential, drug loading efficiency/capacity, and drug release profile). An optimized nanosized system with a high drug loading efficiency and long release time could be used as a carrier for the delivery of a negatively charged LMWH via oral route.
Materials and methods
Materials
LMWH (average molecular weight and anti-FXa activity about 4.26 kDa and 105.1 IU/mg, respectively) was provided as a kind gift sample by Dhruv Life Sciences Pvt. Ltd., Bangalore (India). Tristearin, dichloromethane, azure B certified dye, and tween 80 were purchased from Himedia (Mumbai, India). Chitosan, glyceryl monostearate (GMS) were purchased from CDH (New Delhi, India) and stearyl amine from Sigma Aldrich (St. Louis, MO). Other chemicals, solvents, and reagents were of analytical grade unless otherwise specified.
Methods
Three types of LMWH-containing nanoparticles were prepared by different methods.
Preparation of chitosan nanoparticles (CS-NPs)
The CS-NPs were prepared using ionotropic gelation method as reported earlier with slight modification (Bagre et al. Citation2013, Calvo et al. Citation1997, Paliwal et al. Citation2012). Chitosan was dissolved in aqueous acetic acid solution (1%, w/v). Aqueous solution of TPP with varied concentration was added dropwise into 18 mL chitosan solution under magnetic stirring at room temperature. CS-NPs were formed impulsively upon incorporation of TPP solution under agitation with stirring. LMWH solution was added to the chitosan solution in order to form LMWH loaded CS-NPs. Further, NPs were separated by centrifugation at 20,000 rpm for 20 min.
Optimization of CS-NPs is done by parameters like polymer to TPP ratio, optimization of stirring speed and time, amount of LMWH to chitosan ratio (as shown in ) and their effects are characterized on the drug entrapment efficiency and particle size of optimized formulation.
Table 1. Optimization of different parameters.
Preparation of chitosan polymer lipid hybrid nanoparticles (CS-PLNs)
Double emulsification and solvent evaporation method was used with little modification in the preparation of CS-PLNs (Cheow and Hadinoto Citation2011, Yang et al. Citation2012). In this method, tristearin (lipid) and soy lecithin (primary surfactant) are dissolved in a water-immiscible solvent (DCM) (O-oil phase), while the complex of drug–polymer is separately dissolved in deionized water (W1, internal aqueous phase). The two solutions are emulsified under homogenization to form W1/O nanoemulsion, which is subsequently added to a second aqueous phase containing tween 80 as secondary surfactant (W2, external aqueous phase) to form W1/O/W2 nano-emulsion. Afterwards, the nano-emulsion is stirred overnight at room temperature to evaporate off DCM, and the resultant nanoparticulate suspension is centrifuged twice at 11,000 rpm to remove the non-encapsulated drug and excess lipid.
Preparation of stearyl amine lipid nanoparticles (SA-LNPs)
Double emulsification and solvent evaporation method (Cheow and Hadinoto Citation2011) has been used to prepare the SA-LPNs. In this method, tristearin and soy lecithin are dissolved in a water-immiscible solvent (DCM) (O-oil phase), while the complex of drug–lipid (counter ionic lipid) is separately dissolved in deionized water above 85 °C (W1, internal aqueous phase) (Rawat et al. Citation2008). The two solutions are emulsified under homogenization to form W1/O nanoemulsion, which is subsequently added to a second aqueous phase containing tween 80 (W2, external aqueous phase) to form W1/O/W2 nano-emulsion. Afterwards, the nanoemulsion is stirred overnight at room temperature to evaporate off DCM, and the resultant nanoparticulate suspension is centrifuged twice at 11,000 rpm to remove the non-encapsulated drug and excess lipid.
Characterization of CS-NPs, CS-PLNs, and SA-LNPs
FTIR
IR spectrum is an important record which gives sufficient information about the structure of a compound and checks its purity. IR study was performed for identification and structural analysis of the procured drug using Fourier transformed infrared spectrophotometry (Thermo Scientific, Waltham, MA). IR spectrum of the drug sample was found to be concordant with the reference spectrum as the observed peaks and reported peaks reported. The drug sample, chitosan, stearyl amine, and their combination have been scanned by using IR spectrophotometer in order to check their compatibility.
Particle size and zeta potential
Size, size distribution (PDI), and zeta potential of optimized formulations were determined by laser diffractometry using Beckman Coulter Delsa TM Nano C Particle analyzer (Mumbai, India). For the measurement of size and polydispersity index (PDI), 2 mL of nano lipid carrier was placed into cuvettes of Beckman coulter and measurements were recorded. Size and PDI of LMWH loaded CS-PLNs, SA-LNPs, and CS-NPs were determined. All the analysis was carried out in triplicate.
Shape and surface morphology
The LMWH loaded preparations were characterized for their shape and surface morphology at Panjab University Chandigarh using transmission electron microscope (TEM) Hitachi (H-7500) operating at 100 kV. For the TEM observation, the CS-PLNs were deposited directly on carbon coated TEM copper grids. Morphology of optimized formulations was determined by TEM photomicrographs.
Entrapment efficiency
The entrapment efficiency of LMWH in CS-NPs, CS-PLNs, and SA-LNPs was quantified by measuring the concentration of unentrapped drug in supernatant followed by centrifugation of dispersion using Azure B colorimetric method (Jiao et al. Citation2002). The estimation was done spectrophotometrically with a UV spectrophotometer at 517.55 nm by reacting 500 μL supernatant with 4.5 mL of Azure B solution (0.001% w/v). The entrapment efficiency was calculating using the following equation:
In vitro drug release study
As earlier reported method, the in vitro release study was performed in different GIT simulated conditions (SGF pH 1.2 and SIF pH 7.4) (Jiao et al. Citation2002). Briefly, LMWH-CS-NPs, CS-PLNs, and SA-LNPs dispersion were placed in 10 mL of medium in a flask incubated in a water bath at 37 °C under magnetic stirring at 100 rpm. After regular time intervals, samples were withdrawn with replacement by equal volume of fresh medium. The samples were assayed for LMWH using an Azure II based colorimetric method.
In vitro studies
Permeation studies using Caco-2 cell lines
Cell cultures: Caco-2 cell culture was used to check entry of nanoparticles into the cells, CS-Nps, Cs-PLNs, and SA-LNPs were labeled with the fluorescent dye named fluorescein isothiocyanate (FITC). Although, FITC have negative charge and hydrophilic nature they can easily form complex with chitosan. Briefly, 2 mg of FITC was incorporated in the aqueous phase by replacing LMWH and the preparation continued as aforementioned.
Caco-2 cells were grown in RPMI-1640 medium supplemented with 10% (v/v) inactivated fetal bovine serum, 1% (v/v) trypsin-EDTA solution, at 37 °C under a 10% CO2/90% air atmosphere. Caco-2 cells were poured into a 24-well plate and cultivated over 24 h. The permeability of LMWH across intestinal in vitro models was evaluated by comparing free LMWH with CS-NPs, CS-PLNs, and SA-LNs, in Caco-2 cells.
In vitro permeation study across the intestine (everted intestinal sac model)
The permeation of nanoparticles across the intestine was evaluated by using everted intestinal sac method (Agarwal and Khan Citation2001, Bagre et al. Citation2013). In brief, isolated intestinal segment from wistar rat was everted carefully using glass rod and rinsed with saline solution. Then the segment was cut and affix to the tip of a 1 mL disposable syringe barrel. Modified Kreb’s Ringer phosphate bicarbonate (KRPB) solution with pH 7.4 was filled within the gut sac as the serosal fluid. Then gut sac was placed in a bath containing 50 mL of nanoparticulate suspension (1 mg/mL) in modified KRPB solution on mucosal side. The fluid was regularly bubbled with a mixture of 95% O2 and 5% CO2. The temperature of organ bath was maintained at 37 ± 0.5 °C using water jacket. Samples were withdrawn at regular time intervals and analyzed quantitatively for the presence of LMWH using azure II colorimetric method (Jiao et al. Citation2002).
In vivo studies
The in vivo studies were performed on wistar rats of either sex weighing in the range of 250–300 g. The studies were carried out under the guidelines compiled by CPCSEA (Committee for the Purpose of Control and Supervision of Experiments on Animal, Ministry of Culture, Government of India) in meeting no. 9/2014/Protocol No. 154. All the study protocols were approved by the local animal ethics committee.
Venous thrombosis model
The animal model for thrombosis inhibition study was performed on wistar rats (Kim et al. Citation2007b). Wistar rats were kept under a 12:12 h in light–dark cycle and were used after fasting them for 12 h. In brief, developed LMWH formulations equivalent to 50 mg/kg dose were administered by oral cannula to different groups. A group of animal received 1 mg/kg intravenous injection of marketed LMWH formulation, second group served as control and received PBS plain pH 7.4 equivalent volume, third group was treated with oral LMWH solution, fourth and fifth group administered with CS-PLNs and SA-LNPs. After drug administration, animals were anaesthetized with ketamine administered intra-peritoneal injection. The animals were dissected at abdominal site and jungular vein was carefully isolated for thrombus induction. Thrombosis was induced by applying two pieces of cotton swab saturated with 5% w/v ferric chloride solution. The pieces of cotton swab were placed on the opposite sides of vein (one beneath and one above) in contact with the adventitial surface of vessel. The filter paper was applied for 3 min and then removed. At 120 min, after finishing the surgical operation, the jungular vein was segregated and opened in a petri dish filled with 3.8% sodium citrate. Thrombus formation was evaluated by measuring the dry weight of the thrombus.
Gastrointestinal toxicological study
Animals were divided into three groups and were administered formulations orally with the help of cannula with maximum care and negligible tissue damage. Groups I and II received 0.2 mL of LMWH loaded chitosan polymer lipid hybrid nanoparticles (LMWH-CS-PLNs) and LMWH-SA-LNPs formulations, respectively. Group III served as control and received an equivalent volume (0.2 mL) of PBS buffer solution of pH 7.4 (Paliwal et al. Citation2011). After 1 h of oral administration, animals were sacrificed to isolate different GIT tissue sections, which were fixed in neutral buffer formalin, followed by microtome processing and slide preparation. A minimum of three animals were screened each time. H&E stained tissue were examined carefully under optical microscope for any tissue damage at 40 × (Nikon, Eclipse E200, New Delhi, India).
Results
Characterization of CS-NPs, CS-PLNs, and SA-LNPs
FTIR
Infrared spectra result from transitions between the quantized energy states of the bond or group that vibrates. Modifications specifically derived from chemical interactions and/or electrostatic interactions contribute to band shifts changes in the peak intensity, and peak broadening in the FTIR spectra (Markovich and Pidgeon Citation2007). The IR spectra of LMWH obtained showed peaks at 1636.77 cm−1, which correspond to the stretching vibrations of the C=O in the carboxylate, and at 1235.10 cm−1, which probably results from the S=O stretching of the sulfate ions. The peak for the stretching vibrations of the C–N groups of chitosan could be seen at 1636.83 cm−1 and 1597 cm−1 in stearyl amine. There were clear shifts and changes in the intensity and broadening of the peaks for the stretching vibrations of the C=O and S=O groups of the negatively charged functional groups in LMWH with the increased amounts of chitosan.
Morphology, size, PDI, and entrapment efficiency
The characteristics of the prepared formulations are summarized in in terms of particle size, PDI, zeta potential, and entrapment efficiency. CS-NPs have smaller size and entrapment efficiency as compared to SA-LNPs and CS-PLNs. Results of TEM of different formulations confirm the spherical shape of homogeneous shading with uniformity in particle size as shown in . The entrapment efficiency of formulation acts as a determining factor for dose volume to be administered for desired therapeutic effect. Among the formulations, hybrid CS-PLNs showed maximum %EE.
Table 2. Summary of drug entrapment efficiency, zeta potential, particle size, and PDI of developed formulations.
In vitro release study
The in vitro release behavior of developed formulations was observed in different simulating GIT fluids resembling transit of orally administered formulations. In case of SGF, a higher release of LMWH from CS-NPs, SA-LNPs, and CS-PLNs within 2 h was observed in all cases as compared to release in SIF after 4 h as shown in .
In order to determine the kinetics and mechanism of LMWH release from formulations, various mathematical models of release kinetics were employed such as zero order, first order, Peppas model, and Higuchi model. The value of correlation coefficient (R2) was calculated to determine the results of model fitting to the release data. The value of correlation coefficient for release data in SGF and SIF is given in . The R2 values of all formulations suggest that the drug release data in all cases was best fitted to first order of kinetics and zero order of kinetics in case of SGF and SIF, respectively.
Table 3. Determination coefficient (R2) after fitting the release data.
In vitro studies
Cell uptake via Caco-2 cell lines
The particulate uptake of drug solution, CS-NPs, SA-LNPs, and CS-PLNs by Caco-2 cell lines resembling intestinal epithelium was visualized using fluorescence microscopic analysis. Fluorescein isothiocyanate (FITC) was used as a fluorescence marker and loaded into the nanoparticles by replacing LMWH (Bagre et al. Citation2013) viewed under fluorescence microscope (Nikon Eclipse E 600 microscope equipped with video camera with ECD, software used: Image Proplus, New Delhi, India) at a magnification of 40 × (). The particulate uptake of LMWH, SA-LNPs, and CS-PLNs was visualized using fluorescence microscopic analysis.
In vitro permeation study across the intestine (everted intestinal sac model)
To assess the permeability of formulation across the intestine, everted intestinal sac model was used. In the results, 67.60% and 69.45% of LMWH were observed to reach across the intestine into the serosal fluid from SA-LNPs and CS-PLNs within 2.5 h. Release data are shown in .
In vivo studies
Venous thrombosis model
The formation of thrombus in the coronary or cerebral arteries is the major cause of morbidity and mortality. Animal models of thrombosis critically mimic the human disease and have been widely implemented in antithrombotic drug discovery, ferric-chloride induced thrombosis is a widely used model of thrombosis in various species, including mice, rats, and rabbits.
After 120 min of induction of thrombus, formulations were administered by oral route and their potential for thrombus inhibition was compared with 1 mg/kg, parenteral LMWH dose showed minimum thrombus formation. The developed CS-PLNs and SA-LNPs showed a comparative effectiveness to parenteral LMWH. Consequently, CS-PLNs show maximum reduction in thrombus weight. Moreover, developed oral formulations have greater potential toward thrombus inhibition as compared to simple oral solution and control group as shown in (n=3).
Histological examination of gastrointestinal tract
Photomicrograph () showed H and E staining analysis of the jejunum after single dose oral administration of LMWH loaded CS-PLNs and SA-LNPs and control in order to identify the extent of disruption caused to tissues, if any and also to evaluate safe use of formulation for the purpose. It is clear from the photomicrograph that absorption of LMWH along with NPs did not induce any toxicity to GIT. These results are comparable to control. At the same time, no evidence of damage in villi structure was recorded in any case. Additionally, membrane damage such as mucosa hemorrhage or intestinal villi damage such as occasional epithelial cell shedding, villi fusion, congestion of mucosal capillary with blood and focal trauma was not detected.
Discussion
A new oral delivery system using LMWH-CS-PLNs was precisely developed based on the hypothesis that complex of chitosan and LMWH will neutralize the charge on heparin. Further, chitosan will increase the permeability of complex by reacting with tight junctions to open up and can be an effective tool to prevent H. pylori infection in stomach. In addition, lipid coat increases the lymphatic uptake by increasing its size in order to make it unable to cross the blood capillaries ultimately preventing the hepatic first pass metabolism. Thus this system can be useful for drug delivery in both stomach as well as intestine. The comparison of CS-NPs, lipid nanoparticles, and polymer lipid hybrid nanoparticles was studied and it can be concluded that PLNs have better characteristics and thus PLNs might be the best option.
Further, the study exhibited the effect of different lipids on particle size and PDI. The particle size and particle size distribution are critical factors in the performance of nanoparticles, as batches with wide particle size distribution may show significant variations in drug loading, drug release, bioavailability, and efficacy. Formulation of nanoparticles with a narrow size distribution will be a challenge if emulsion cannot be produced with a narrow droplet size distribution. As nanoparticles are internalized into cells by endocytosis, an increase in particle size will decrease uptake and potentially affect bioavailability of the drug.
FTIR spectroscopy records the specific absorption wavelength and intensity of functional groups present in a molecule. When the environment of a functional group changes, such as an interaction with an oppositely charged group or the formation of new bonds after a chemical reaction, some degree of change in absorption bands occurs (Markovich and Pidgeon Citation2007). LMWH is composed of repeating disaccharide units of d-glucosamine and uronic acid linked by 1,4-interglycosidic bonds. The main charged functional groups in the disaccharide unit of LMWH are repeating carboxylate (COO=) and sulfate ester (SO3=) groups, which contribute to the highly negative charges of the LMWH molecule. The main functional groups in chitosan and stearyl amine are the NH2 groups or its cationic charged form, NH3+. In this study, there were distinct differences between the IR spectra of the LMWH–chitosan and LMWH–stearyl amine complexes and those of LMWH or chitosan/stearyl amine alone. The positions, intensities, and the broadening of the peaks for the COO= and SO3= groups of LMWH were altered. Overall, the spectra of LMWH, chitosan, and LMWH–chitosan complex suggest an electrostatic interaction between the cationic amino group of chitosan and the anionic sulfate or carboxylic acid group of LMWH. This type of interaction could be used to form LMWH nanoparticles by polyelectrolyte complexation.
The hybrid CS-PLNs gave better entrapment efficiency, lesser particle size and optimum PDI and potential due to charge stabilizing complex formation and as mentioned earlier that the ability of lipid to form fine emulsion. The behavior of lipid concerning the EE % could be explained by the fact that increasing the amount of lipid, EE % is bound to increase because of the increased concentration of mono-, di-, and triglycerides that act as solubilizing agents for drug–polymer complex (Mukherjee et al. Citation2007).
Zeta potential is a measure of the surface electrical changes of particles and has often been used to characterize the colloidal drug delivery system. The magnitude of zeta potential gives an indication of the potential stability of colloidal system. LMWH loaded LNPs and PLNs had a negative surface charge that could arise from the adsorption of hydroxyl ions of lipid, at the o/w interface. The significant negative value clearly indicated that the concentration of polymer was the major factor in stabilizing AZT-LPN dispersions. This could be the result of electro-steric stabilization by the polymer.
The release studies were carried out at SGF (pH 1.2) and SIF (pH 7.4). Totally 40% release was observed in 2–3 h. The reason for fast release could be degradation of lipid layer in gastric environment due to instability of lipid in stomach and may be also due to release of un-trapped drug due to loss of lipid layer which was present inside lipid coat which did not make complex with chitosan. During this short drug release in stomach, chitosan–LMWH complex has permeated by opening the tight junctions. In intestine, particle showed slower release because of stability of lipid layer, where very short release particle will be entered in lymphatic duct and acts as chylomicron. CS-PLNs showed better sustained release in both medium, i.e., SGF and SIF by releasing minimum amount of drug as compared to others.
CS-PLNs showed better uptake as compared to SA-LNPs. The cellular uptake of NLCs was size-dependent. This finding is consistent with Rejman et al. (Citation2004) who also reported a tendency to decreased internalization with increased particle size. These authors studied the pathway of entry and subsequent fate of commercial latex nanoparticles inside the cell and concluded that particles with a diameter of less than 200 nm enter the cell via clathrin-mediated endocytosis whereas larger particles (200 nm to 1 μm) enter preferentially via caveolae-mediated endocytosis (Rejman et al. Citation2004). Moreover, the surface hydrophobicity of the nanoparticles may also determine nanoparticle entrance into Caco-2 cell because Caco-2 cell lines mimic the intestinal epithelia which well accepted for lipid particles. In our study, nanoparticle size and surface hydrophobicity were the major factors influencing PLNs entrance into the cell. Further, transfer of 70% LMWH across the intestinal epithelium after 2.5 h in the in vitro permeation study performed using everted intestinal sac model showed better qualitative correlation between in vitro Caco-2 call line and ex vivo intestinal sac of wistar rat for probable potential of oral delivery.
This potential is further explored by the pharmacodynamic studies performed using venous thrombosis model in wistar rats. The developed CS-PLNs and SA-LNPs showed a comparative effectiveness to parenteral LMWH. Moreover, developed oral formulations have greater potential toward thrombus inhibition as compared to simple oral solution and control groups. Moreover, no damage detected in histological examination of jejunum proved the safety and efficacy of the formulation. Therefore, the LPN is an attractive vehicle with good performance of efficient cellular uptake, entrapment efficiency, in vivo and ex vivo behavior, sustained release as per requirement of every drug delivery system.
Conclusions
In this study, we report the use of both polymer and lipid in hybrid nanoparticulate system for oral delivery of LMWH. The newly developed PLNs had excellent performance features by smaller size, acceptable biocompatibility, higher drug encapsulation efficiency especially for anionic hydrophilic drugs, sustained release characteristics than NPs and LNPs alone. The present study might provide a new strategy to deliver water soluble drugs for oral delivery.
Acknowledgements
The authors would like to thank Dhruv Life Sciences Pvt. Ltd., Bangalore (India) for providing gift sample of LMWH for the research work and ISF College of Pharmacy, Moga for providing research facilities.
Disclosure statement
The authors declared that they have no conflict of interest.
References
- Agarwal V, Khan MA. 2001. Current status of the oral delivery of insulin. Pharm Technol. 10:76–90.
- Anderson JL, Adams CD, Antman EM, Bridges CR, Califf RM, Casey DE, et al. 2007. ACC/AHA 2007 Guidelines for the Management of Patients With Unstable Angina/Non-ST-Elevation Myocardial Infarction. A Report of the American College of Cardiology/American Heart Association Task Force on Practice Guidelines (Writing Committee to Revise the 2002 Guidelines for the Management of Patients With Unstable Angina/Non-ST-Elevation Myocardial Infarction) Developed in Collaboration with the American College of Emergency Physicians, the Society for Cardiovascular Angiography and Interventions, and the Society of Thoracic Surgeons Endorsed by the American Association of Cardiovascular and Pulmonary Rehabilitation and the Society for Academic Emergency Medicine. J Am Coll Cardiol. 50:e1–e157.
- Antman EM, Anbe DT, Armstrong PW, Bates ER, Green LA, Hand M, et al. 2004. ACC/AHA guidelines for the management of patients with ST-elevation myocardial infarction: a report of the American College of Cardiology/American Heart Association Task Force on Practice Guidelines (Committee to Revise the 1999 Guidelines for the Management of Patients with Acute Myocardial Infarction). J Am Coll Cardiol. 44:E1–E211.
- Bagre AP, Jain K, Jain NK. 2013. Alginate coated chitosan core shell nanoparticles for oral delivery of enoxaparin: in vitro and in vivo assessment. Int J Pharm. 456:31–40.
- Bai S, Ahsan F. 2009. Synthesis and evaluation of pegylated dendrimeric nanocarrier for pulmonary delivery of low molecular weight heparin. Pharm Res. 26:539–548.
- Breddin HK, Hach-Wunderle V, Nakov R, Kakkar VV. 2001. Effects of a low-molecular-weight heparin on thrombus regression and recurrent thromboembolism in patients with deep-vein thrombosis. N Engl J Med. 344:626–631.
- Calvo P, Remunan‐Lopez C, Vila‐Jato J, Alonso M. 1997. Novel hydrophilic chitosan‐polyethylene oxide nanoparticles as protein carriers. J Appl Polym Sci. 63:125–132.
- Chen MC, Wong HS, Lin KJ, Chen HL, Wey SP, Sonaje K, et al. 2009. The characteristics, biodistribution and bioavailability of a chitosan-based nanoparticulate system for the oral delivery of heparin. Biomaterials. 30:6629–6637.
- Cheow WS, Hadinoto K. 2011. Factors affecting drug encapsulation and stability of lipid-polymer hybrid nanoparticles. Colloids Surf B Biointerfaces. 85:214–220.
- Geerts WH, Bergqvist D, Pineo GF, Heit JA, Samama CM, Lassen MR, et al. 2008. Prevention of venous thromboembolism. American College of Chest Physicians Evidence-Based Clinical Practice Guidelines. CHEST J. 133:381S–453S.
- Hallan SS, Kaur P, Kaur V, Mishra N, Vaidya B. 2016. Lipid polymer hybrid as emerging tool in nanocarriers for oral drug delivery. Artif Cells Nanomed Biotechnol. 44:334–349.
- Hayes PY, Ross BP, Thomas BG, Toth I. 2006. Polycationic lipophilic-core dendrons as penetration enhancers for the oral administration of low molecular weight heparin. Bioorg Med Chem. 14:143–152.
- Hoffart V, Lamprecht A, Maincent P, Lecompte T, Vigneron C, Ubrich N. 2006. Oral bioavailability of a low molecular weight heparin using a polymeric delivery system. J Control Release. 113:38–42.
- Hull RD, Pineo GF, Raskob GE. 2004. The economic impact of treating deep vein thrombosis with low-molecular-weight heparin: outcome of therapy and health economy aspects. Pathophysiol Haemost Thromb. 28(Suppl. 3):8–16.
- Hwang SR, Seo DH, Al-Hilal TA, Jeon OC, Kang JH, Kim SH, et al. 2012. Orally active desulfated low molecular weight heparin and deoxycholic acid conjugate, 6ODS-LHbD, suppresses neovascularization and bone destruction in arthritis. J Control Release. 163:374–384.
- Javot L, Lecompte T, Rabiskova M, Maincent P. 2009. Encapsulation of low molecular weight heparins: influence on the anti-Xa/anti-IIa ratio. J Control Release. 139:8–14.
- Jiao Y, Ubrich N, Marchand-Arvier M, Vigneron C, Hoffman M, Lecompte T, et al. 2002. In vitro and in vivo evaluation of oral heparin-loaded polymeric nanoparticles in rabbits. Circulation. 105:230–235.
- Kearon C, Kahn SR, Agnelli G, Goldhaber S, Raskob GE, Comerota AJ. 2008. Antithrombotic therapy for venous thromboembolic disease. American College of Chest Physicians evidence-based clinical practice guidelines. CHEST J. 133:454S–545S.
- Kim CY, Kim J, Han K, Kim S, Park K, Byun Y. 2007a. Toxicity screening after repeated dose of a newly developed oral heparin derivative in male cynomolgus monkeys. J Toxicol Sci. 32:411–420.
- Kim SK, Huh J, Kim SY, Byun Y, Lee DY, Moon HT. 2011. Physicochemical conjugation with deoxycholic acid and dimethylsulfoxide for heparin oral delivery. Bioconjug Chem. 22:1451–1458.
- Kim SK, Lee DY, Kim CY, Nam JH, Moon HT, Byun Y. 2007b. A newly developed oral heparin derivative for deep vein thrombosis: non-human primate study. J Control Release. 123:155–163.
- Lensing AW, Prandoni P, Prins MH, Büller H. 1999. Deep-vein thrombosis. The Lancet. 353:479–485.
- Liautard C, Nunes A-MC, Vial T, Chatillon F, Guy C, Ollagnier M, et al. 2002. Low-molecular-weight heparins and thrombocytosis. Ann Pharmacother. 36:1351–1354.
- Markovich RJ, Pidgeon C. 2007. Introduction to Fourier transform infrared spectroscopy and applications in the pharmaceutical sciences. Pharm Res. 8:663–675.
- Mukherjee S, Ray S, Thakur R. 2007. The current status of solid lipid nanoparticles. Pharmabit XV. 1:53–60.
- Paliwal R, Paliwal SR, Agrawal GP, Vyas SP. 2011. Biomimetic solid lipid nanoparticles for oral bioavailability enhancement of low molecular weight heparin and its lipid conjugates: in vitro and in vivo evaluation. Mol Pharm. 8:1314–1321.
- Paliwal R, Paliwal SR, Agrawal GP, Vyas SP. 2012. Chitosan nanoconstructs for improved oral delivery of low molecular weight heparin: in vitro and in vivo evaluation. Int J Pharm. 422:179–184.
- Paliwal R, Rai S, Vaidya B, Khatri K, Goyal AK, Mishra N, et al. 2009. Effect of lipid core material on characteristics of solid lipid nanoparticles designed for oral lymphatic delivery. Nanomed: Nanotechnol Biol Med. 5:184–191.
- Ramadan A, Lagarce F, Tessier-Marteau A, Thomas O, Legras P, Macchi L, et al. 2011. Oral fondaparinux: use of lipid nanocapsules as nanocarriers and in vivo pharmacokinetic study. Int J Nanomed. 6:2941.
- Rawat A, Majumder QH, Ahsan F. 2008. Inhalable large porous microspheres of low molecular weight heparin: in vitro and in vivo evaluation. J Control Release. 128:224–232.
- Rejman J, Oberle V, Zuhorn I, Hoekstra D. 2004. Size-dependent internalization of particles via the pathways of clathrin- and caveolae-mediated endocytosis. Biochem J. 377:159–169.
- Vyas S, Rai S, Paliwal R, Gupta PN, Khatri K, Goyal AK, et al. 2008. Solid lipid nanoparticles (SLNs) as a rising tool in drug delivery science: one step up in nanotechnology. Curr Nanosci. 4:30–44.
- Yang T, Nyiawung D, Silber A, Hao J, Lai L, Bai S. 2012. Comparative studies on chitosan and polylactic-co-glycolic acid incorporated nanoparticles of low molecular weight heparin. AAPS PharmSciTech. 13:1309–1318.