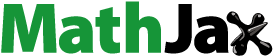
Abstract
In the present study, a sustainable green chemistry approach was established to fabricate magnetic Fe3O4 nanoparticles (Fe3O4NPs) using the aqueous fruit extract of edible C. guianensis (CGFE). Synthesized NPs were further confirmed with different high-throughput characterization techniques such as UV–visible spectroscopy, FT-IR, XPS, DLS and zeta potential analysis. Additionally, XRD, AFM, HRTEM and SQUID VSM demonstrate the generation of crystalline CGFe3O4NPs with mean diameter of 17 ± 10 nm. Interestingly, CGFe3O4NPs exhibit a stupendous bactericidal action against different human pathogens which depicts its antimicrobial value. A significant dose-dependent cytotoxic effect of CGFe3O4NPs was noticed against treated human hepatocellular carcinoma cells (HepG2).
Introduction
Magnetic nanoparticles (MNPs) are the foremost nanoscale materials with many practical applications such as ferrofluids, biosensors, catalysts, separation processes and environmental remediation [Citation1]. It plays a central role in wide range of biomedical applications such as cell therapy [Citation2], drug delivery [Citation3], photothermal effect [Citation4], tissue engineering [Citation5], regenerative medicine [Citation6], hyperthermia [Citation7], diagnosis [Citation8] and imaging [Citation9]. Especially, Fe3O4 (ferric oxide) belonging to the class of metal oxides was very well known for its unanimous photo catalytic and photo-oxidizing capacities. Due to their unique physio-chemical properties and capability to facilitate the cellular and molecular level of biological interactions, MNPs are being considered as the successful contrast agents of magnetic resonance imaging (MRI) in theranostics nanomedicine [Citation10].
Conventional physical and chemical methodologies for Fe3O4 fabrication were co-precipitation, thermal decomposition, microemulsion, sonochemical synthesis, sol–gel reactions, hydrothermal, decomposition of organometallic precursors and polyol methods [Citation11]. With the concern of environmental contaminations and energy consumption, a sustainable biosynthesis involving either unicellular or multicellular organisms has been deliberated as an eco-friendly and safe route for nanomaterial preparation [Citation12].
Nature has devised with various processes for the synthesis of nano, micro-length scaled inorganic materials that were considered as a new and largely unexplored area of research. A very wide range of biological resources from both prokaryotes and eukaryotes have been used for the synthesis of different nanoparticles such as gold (Au), silver (Ag), copper (Cu), platinum (Pt), palladium (Pd), iron (Fe), zinc (Zn) and titanium (Ti) [Citation13,Citation14]. While microbial based protocols suffered with microbial culture maintenance, tedious procedure, multiple purification and elongated down-stream processing [Citation15]. Different plant entities were successfully employed to synthesize nanostructures of various metals and metal oxides. Owing to its availability, rapid synthesis, and easy scaling-up process, phyto-mediated nanoparticles generation was considered as the most appropriate route. The bioreduction of metal was achieved by a combination of biomolecules existing in plant extract, resulting in the formation of metal NPs has also been extensively reviewed [Citation16].
The main rationale for nano-based therapeutics development was to find an effective drug candidate in order to overcome the persistence of deadliest diseases and prevalence of multiple drug resistance (MDR) [Citation17]. In particular, cancer continues to be the worldwide killer resulting in major mortality and morbidity, as per 2014 statistics reported by World Health Organization (WHO), more than 10 million new cancer cases arise, results in six million deaths per year and incidence rate will get two-fold increase by the end of 2020 [Citation18].
Multifunctional nanomaterials deliver several imaging options to localize the diseased tissues, also they help in the delivery of therapeutic agents at desired sites without causing adverse effects to normal tissues [Citation19]. In this prime time, development of theranostic agents based on the “all-in-one” approach shows great potential in the emerging field of personalized nanomedicine. It enables early diagnosis and monitoring of an individual patient and to deliver anticancer agents over an extended period for enhanced therapeutic efficacy [Citation20].
A new paradigm for cancer treatment was established by means of nanocrystallization of bioactive principles, and the enhanced permeation and retention effect (EPR) of tumour vessels, which improve the bioavailability of natural anticancer drugs. It also minimizes the adverse dose limiting systemic adverse side effects of the drugs [Citation21]. Magnetic NPs can serve as an efficient vehicle system to reach the tumour site as well as the tumour microenvironment, which is also called as the stromal tumour component. Hence, the tumour microenvironment controls tumour progression and the spread of cancer in the body. In addition, magnetic NPs can augment the contrast in tumour MRI [Citation22].
The plant material exploited to synthesize magnetic Fe3O4NPs was C. guianensis, commonly known as cannonball tree belonging to Lecythidaceae family. Different extracts from the leaf and fruit of this tree are known to possess numerous medicinal values such as antibiotic, antifungal, antiseptic, anticancer, antioxidant, antihelmintic, wound healing and analgesic qualities [Citation23]. Juice made from the leaves was used to cure skin diseases and malaria [Citation24]. It also holds antiulcer, antifertility, antibiofilm, antipyretic, immunomodulatory, antiarthritic, antistress, antidiarrheal, ovicidal, larvicidal activities and neuropharmacological action in nature [Citation25]. Hence, in the present study, we have approached for the green synthesis of magnetic Fe3O4NPs using C. guianensis aqueous fruit extract towards bactericidal and cancer theranostic applications.
Materials and methods
Materials
Ferric chloride hexahydrate (FeCl3·6H2O) was purchased from Merck Millipore and 25% ammonia solution was purchased from Sigma Aldrich (Alwar, India). Foetal bovine serum (FBS), Dulbecco’s Modified Eagle Medium (DMEM), antibiotics, trypsin–EDTA, phosphate buffer saline, nutrient broth, agar-agar, streptomycin sulphate, sterile paper discs and other chemicals were purchased from Himedia Laboratories (Mumbai, India). HepG2 cell line was procured from National Centre for Cell Sciences (NCCS) (Pune, India). Bacterial pathogens used in this study: S. aureus MTCC 96, E. coli MTCC 2939, S. typhi MTCC 3917, K. penumoniae MTCC 530 were obtained from Microbial Type Culture and Collection (MTCC) (Chandigarh, India). Fresh and healthy cannonball fruits were collected from Gokarnesvarar Temple (Pudukkottai, India).
Synthesis of CGFe3O4NPs
To synthesize CGFe3O4NPs, the collected cannonball fruit samples were scraped to remove the outer peel, chopped into small pieces and dried under shade condition for 8–10 days. Then, the dried fruit samples were ground into fine powder using commercial electrical stainless steel kitchen blender and the extract was prepared by simple decoction method. Briefly, 20 g of autoclaved fruit powder was mixed in 400 ml of sterile distilled water and boiled in 60 °C for 20 min to get maximum extraction. Subsequently, the extracts were filtered through Whatman no. 1 filter paper to remove the debris and stored at 4 °C for the further studies. The CGFe3O4NPs were prepared via co-precipitation method, for that 0.1 M FeCl3·6H2O was continuously mixed with CGFE extract in a 1:1 ratio at room temperature for 30 min. Then, the pH was adjusted to 10.5 with 25% ammonia solution, and heated at 80 °C for 30 min under constant stirring with a magnetic stirrer in N2 atmosphere. Finally, the precipitate was centrifuged at 20,000 rpm for 20 min at 10 °C (Eppendorf) then the pellet was re-suspended in N2 purged water and washed repeatedly for three times. The final pellet was dispersed in 5 ml of MilliQ water, frozen at −80 °C and lyophilized for two days to get fine magnetic powder for further studies.
Instrumentation
Generation of CGFe3O4NPs by reducing the respective metal ion Fe3+solution with aqueous CGFE was easily confirmed using the Perkin-Elmer Lamda-45 UV–Vis spectroscopy. The absorption spectra of metal ion precursor FeCl3·6H2O and CGFe3O4NPs were measured using a UV–Vis spectrophotometer in 300–700 nm range. FTIR measurement was carried out to identify the potential biomolecules in CGFE responsible for reduction and stabilization of CGFe3O4NPs. The samples were pelletized using KBr powder and dried, then the transmittance was recorded using JASCO 460 plus FTIR spectrometer in wavelength range between 4000 and 400 cm−1. Further, the X-ray diffraction pattern of CGFe3O4NPs was acquired using PAN analytical X pert PRO Model in conditions at 40 kV and 30 mA in Cu, Ka radiation and mean particles size (L) of CGFe3O4NPs was calculated using following Debye–Scherrer’s equation.
where λ is the wavelength of the X-ray, β is the full width and half maximum and θ is Bragg's angle. The surface oxidation state and presence of elemental composition were studied with XPS analysis using an omicron ESCA spectrometer with monochromatized Al Ka radiation. Dynamic light-scattering measurement was obtained to analyse the size dispersity of synthesized CGFe3O4NPs using Nano-ZS90 analyser apparatus at 25 °C and started 2 min after the cuvette was placed in the DLS apparatus to allow the temperature to equilibrate. In addition, the zeta potential analysis of CGFe3O4NPs was carried out using a Malvern Zetasizer (Malvern, UK). Further, the precise size, shape and distribution pattern of CGFe3O4NPs and EDAX were obtained using a (TECNAI-10) TEM operated at an accelerating voltage of 100 kVe. A VSM-BS2–11 Tesla was used to study the magnetic properties of synthesized CGFe3O4NPs. The field dependence of magnetization was recorded at 10 K and 300 K under circulate magnetic field ranging between 2 and –2 T.
In vitro anticancer activity
Cell lines and culture medium
The HepG2 cells were grown in DMEM supplemented with 10% inactivated FBS, penicillin (100 IU/ml), streptomycin (100 μg/ml) and amphotericin B (5 μg/ml) in humidified atmosphere of 5% CO2 at 37 °C until confluent. The cells were dissociated with trypsin–EDTA solution (0.2% trypsin, 0.02% EDTA, 0.05% glucose in PBS). The stock cultures were grown in 25 cm2 culture flasks, and all experiments were carried out in 96 microtiter plates (Tarsons India Pvt. Ltd., Kolkata, India).
Cell viability assay
The cytotoxic effect of CGFe3O4NPs and crude CGFE was tested by MTT (Sigma, St. Louis, MO) assay on HepG2 cells [Citation26]. For that, the cells were seeded in 96-well plates at a density of 1 × 104 cells per well. After incubation for 20–24 h, the cells with 70–80% confluency were treated with CGFe3O4NPs and crude CGFE at different concentrations (1, 5, 10, 25, 50, 75, 100, 150, 200 and 250 μg ml–1) and incubated for 24 h. Then, 20 μl of MTT (5 mg/ml) solution was added to cells per well, and the plate was further kept in 5% CO2 at 37 °C for another 4 h. After that, the medium was removed, and 150 ml of DMSO was added to the cells. The plate was gently shaken for 15 min to dissolve the formazan crystals generated by proliferating cells, and the measurement was performed using a Spectramax M2 Microplate Reader (Molecular Diagnostic, Inc., Tucson, AZ) at 570 nm with reference filter as 655 nm. Relative viability was calculated with non-treated cells as 100% control. The results were expressed as mean values (±SD) of six repeats.
Measurement of cytomorphological changes in HepG-2
HepG2 cells were pre-treated with different concentration of synthesized CGFe3O4NPs and crude CGFE for 24 h at 37 °C in 5% CO2 atmosphere. After the incubation of cells, the gross morphological changes in the cells were observed under an inverted phase contrast microscope (Nikon, Tokyo, Japan).
Fluorescent imaging
Acridine orange (AO) and ethidium bromide (EtBr) staining was used to detect the morphological features of apoptotic and necrotic cell death. The IC50 concentration of CGFe3O4NPs and crude CGFE was treated with HepG2 cells (1 × 105 cells/ml). After 24 h, the cells were washed with ice-cold 1× PBS and stained with a mixture of AO (100 mg/ml) and EtBr (100 mg/ml) at room temperature for 5 min. After washing with PBS, the stained cells were observed under a fluorescence microscope (Model: OLYMPUS IX70, Olympus Optical Co., Ltd., Tokyo, Japan) at 100× magnification via a particular filter at 510–590 nm and photographs were acquired.
Haemocompatibility assay
To assess the haemocompatibility of synthesized CGFe3O4NPs, fresh blood was collected from healthy volunteers in sterile lithium heparin vacutainers. The samples were individually suspended in 10 mM HEPES buffered saline and red blood cells (RBCs) were separated by Ficoll density gradient centrifugation (1500 rpm for 10 min at 4 °C). Collected RBCs were further diluted in 20 mM HEPES buffered saline (pH 7.4) to form a 5% v/v solution. Then, the RBC suspension was added to HEPES saline, 1% Triton X-100 and samples with different concentrations and incubated at 37 °C for 60 min. All the samples were prepared in triplicate, after being slightly vortexed the suspension was incubated under static conditions for 4 h at 37 °C. Then, all the samples were centrifuged (Heraeus table top centrifuge 5805R, Hanau, Germany) at 12,000 rpm at 4 °C and the supernatants were transferred to a 96-well plate. The haemolytic activity was determined by measuring the absorbance at 570 nm (Biorad microplate reader model 550, Tokyo, Japan). Control samples of 0% lysis (in HEPES buffer) and 100% lysis (in 1% Triton X-100) were employed in the experiment. The percentage of haemolysis was calculated as follows:
This study was approved by the Institutional Ethics Committee (IEC) of Bharathidasan University (Ref no. DM/2014/ 101/54). A consent form with all the mandatory information was collected from the healthy volunteers for their participation in this study, and the form was submitted to IEC, Bharathidasan University.
Antibacterial activity
The antimicrobial effect of synthesized CGFe3O4NPs and crude CGFE was evaluated against different bacterial human pathogens such as S. aureus MTCC 96, E. coli MTCC 2939, S. typhi MTCC 3917 and K. penumoniae MTCC 530 by disc diffusion method [Citation27]. Briefly, the bacterial strains were sub-cultured in nutrient broth for 24 h at 30 °C. Each strain was swabbed into the separate nutrient agar plates using sterile cotton swabs. Using sterile micropipette, different volumes (25, 50 and 75 μg/ml) of CGFe3O4NPs and crude CGFE were loaded onto sterile discs and allowed to dry. The sample loaded discs and standard antibiotic discs (streptomycin 1 mg/ml) were impregnated in the nutrient agar medium. In this study, the doses were fixed based on the preliminary data obtained from the earlier studies in our laboratory. After 24 h incubation at 37 °C, the different levels of zone of inhibition were measured.
Results and discussion
The essential phytochemicals such as terpenoids, flavones, ketones, aldehydes, amides and carboxylic acids are mainly responsible for the instant direct reduction of metallic ions and produce nano-sized particles [Citation28]. In this study, we have developed a new facile route to fabricate magnetic CGFe3O4NPs functionalized with active constituents of C. guianensis for therapeutic applications.
Synthesis of CGFe3O4NPs
After the addition of CGFE into precursor FeCl3 solution, formation of CGFe3O4NPs was noticed with a change in the colour of reaction medium (). Synthesized NPs exhibit light red to dark brown colour whereas the precursor FeCl3 was golden yellow in colour. Water-soluble fractions such as phenols and phenolic acids of CGFE mainly act as reducing and surface functionalization agents. The possible reduction mechanism of CGFe3O4NPs formation can be elucidated by comparison with the related mechanism through reduction of FeCl3 using bioactive phyto-molecules [Citation29]. Based on this mechanism, the hydroxyl and amine groups of bioactive molecules in plant extracts tend to bind with Fe3+ and form ferric hydroxide, which is partially reduced by other bioactive materials to produce Fe3O4 particles.
Figure 1. Synthesis of CGFe3O4NPs (a). Formation of dark brown color in tube 3 indicates the generation of CGFe3O4NPs (b). UV?visible spectroscopic analysis of CGFe3O4NPs produces the absorbance spectra around 250–300 nm whereas FeCl3 generates no absorbance spectra (c). FTIR spectrum of CGFE (bottom) and CGFe3O4NPs (top) specifies the functional groups active bio constituents responsible for reduction and stabilization.
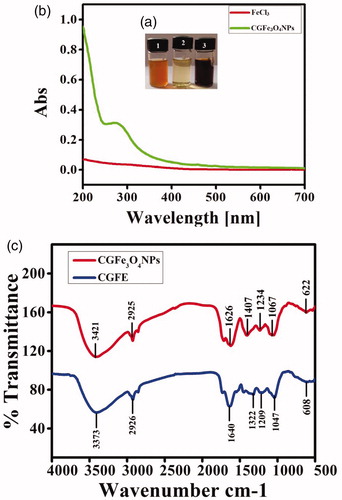
Characterization of CGFe3O4NPs
UV–visible spectrum of synthesized CGFe3O4NPs produces a strong absorbance in the visible region at around 250–350 nm (), confirms that the particles were stable and well dispersed in the solution. This result also clearly depicts that the hydroxyl group containing plant biomaterials are in strong coordination with transition metals. Our results highly corroborate with Zhang et al. [Citation30] where folate-conjugated polymer coated supermagnetic iron oxide nanoparticles have shown the absorbance values in visible region.
FTIR spectroscopic analysis was used to study the interactions metal ions (Fe) with major functional groups of CGFE biocompounds as well as the functionalization of CGFe3O4NPs. As shown in , for CGFE, a broad characteristic peak from 3600 to 3100 cm–1 could be assigned to the O–H or N–H stretching vibration arising from hydroxyl groups of polyphenols and some aromatic heterocyclic organic compounds [Citation31]. A band at 1640 cm–1 attributes the presence of carbonyl groups C=O (1610–1780 cm–1) and existence of C–H was confirmed by the stretching vibrations at 2925 cm–1. FTIR spectrum of synthesized CGFe3O4NPs shows variation in the intensities of transmittance due to the interaction of metal ions with CGFE biocompounds, which leads to the reduction and stabilization of the Fe3O4 nanoparticles [Citation32]. The intensities of transmittance at 3421, 2925, 1626, 1407, 1234 and 1067 cm–1 were increased dramatically for Fe3O4 nanoparticles. Interestingly, a new band at 590 and 622 cm–1 attributes the intrinsic stretching vibrations of the metal at a tetrahedral site [Citation33]. In addition, the transmittance located at 1067 cm–1 represents the presence of C–O stretching in the region of 1300 and 1000 cm–1 which might be due to the existence of covalent linking of ether or ester groups with NPs [Citation34]. Consequently, the FTIR results indicate the vital role of plant bioactive metabolites such as polyphenols, proteins and reducing sugars in reduction and stabilization of CGFe3O4NPs.
XPS spectra of CGFe3O4NPs prepared via bioreduction method show distinct spectra for O1s, C1s and N1s region at 529.6, 283.8 and 398.5 eV, respectively (), which represents the existence of different biomoieties onto the surface of Fe3O4 modified with CGFE. The binding energy values for the main peak maxima Fe 2p3/2 and 2p1/2 were found at 711.1 and 724.5 eV, respectively (). Our results are in good agreement with the values reported for Fe3O4 in the literature [Citation35]. The main C1s peak at 283.8 eV () can be assigned to the carbon atoms within phenyl and carbonyl groups residues of the polyphenolic constituents. The O1s core level () decomposed into three chemically distinct components at 529.8 eV denotes the existence of carboxylate groups. The N1s core level centred at 398.5 attributes the amine groups present in the indole derivatives and protein moieties. From these results, we speculate that the Fe3+ ions are in strong coordination with water-soluble heterocyclic metabolites of CGFE. During nucleation, these active bioconstituents could be preferentially adsorbed onto the surface, also it controls the nucleation position through special confinement [Citation36].
The crystalline structure of CGFe3O4NPs was characterized by XRD. As shown in , the interplanar distances for biosynthesized CGFe3O4NPs were noticed at 2θ = 30.08°, 35.54°, 43.07°, 53.4357°, 56.95° and 62.54°, corresponding to each Bragg reflection (220), (311), (400), (422), (511) and (440) can be well indexed as the inverse cubic spinel structure of magnetite Fe3O4 in accordance with Joint Committee on Powder Diffraction Standards (JCPDS File No.: 75-1610). As per Scherrer’s equation, D=kλ/B cos θ, the average crystallite size was calculated to be 7.2 nm. Similar results were obtained by Silva et al. [Citation37] for fucan polysaccharides coated magnetite MNPs.
Figure 3. XRD pattern of synthesized CGFe3O4NPs: (a) diffraction planes of CGFe3O4NPs denote the cubic spinel structure and (b) zeta potential analysis of CGFe3O4NPs.
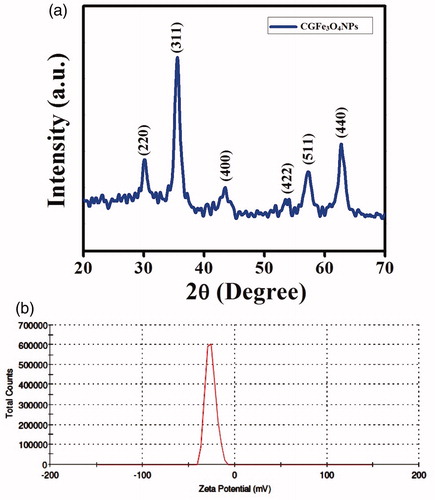
The stability of CGFE-stabilized Fe3O4 colloidal solution has been investigated based on the zeta potential (ζ), which is a measure of electrostatic potential on the surface of nanoparticles, and it is associated with the electrophoretic mobility of colloidal suspension. The zeta potential of CGFe3O4NPs was measured in the growth medium as −26.0 mV and the surface of CGFe3O4NPs was negatively charged (). Due to the low surface charge, Fe nanoparticles get easily aggregated and become unstable, the synthesized CGFe3O4NPs exhibit a large negative zeta value (−26.0 mV) mainly because of the surface coating with plant biomolecules which generates a repulsive forces between nanoparticles [Citation38].
Surface topography of synthesized CGFe3O4NPs was studied with AFM images. Unlike the electron microscopic analysis, which provide a two-dimensional projection or a two-dimensional image of a sample, AFM provides a three-dimensional surface profile (). Although, the lateral dimensions are influenced by probe shape, the height measurements can provide the height of nanoparticles with a high degree of accuracy and precision. As shown in the histogram of AFM (), the size distribution of magnetic CGFe3O4NPs was between 7 and 77 nm. Our data highly match with the previous report by Banerji et al. (2011) [Citation39] where Fe3O4NPs conjugated with 5-fluorouracil show highly stable, oval shaped Fe3O4 with nearly 10 nm diameter. It was also observed that the free iron oxide nanostructures are prone to quick agglomeration whereas the 5-FU coated NPs are stable to coagulation because of their lack of free reactive surface for agglomeration [Citation39].
Figure 4. AFM images show the topography and size distribution of synthesized CGFe3O4NPs (a) 2D-micrographs, (b) 3D-micrographs, (c) 2D-roughness, (d) 3D-roughness and (e) size distribution.
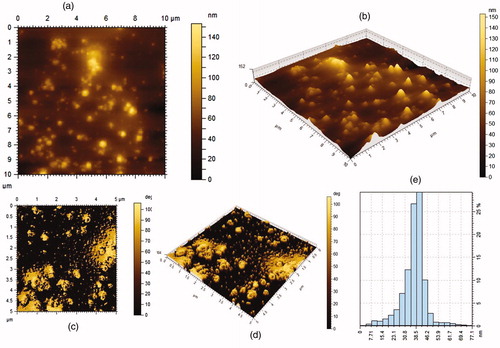
The TEM micrographs show spherical and polydispersed NPs with sizes ranging between 7 and 80 nm; the mean size was found to be 17 ± 10 nm. The HRTEM micrograph shows the fine configuration of the synthesized CGFe3O4NPs. A particle size distribution histogram based on the size of 50 particles was measured from the TEM image as shown in . The mean particle size was 17 nm with the standard deviation of 10, it was quite close to the calculated crystallite size of 7 nm, suggesting that the majority of the observed spherical nanoparticles might be single crystals [Citation40]. Besides, exposes the EDX spectrum of CGFe3O4NPs, a strong signal with the highest percentage at 6.2 keV region was noticed for Fe indicating the purity and stability of synthesized NPs. It was also revealed that the existence of iron and oxygen confirmed the formation of Fe3O4 nanoparticles, and a typical optical absorption peak at B 0.5 keV due to surface plasmon resonance was observed. The presence of Cu was attributed to the carbon coated copper grid used for sample preparation. According to many [Citation41] earlier studies, our results clearly confirm the reduction and formation of highly stable, surface modified spherical Fe3O4NPs, via direct reduction, using C. guianensis fruit extract.
Figure 5. HRTEM measurements of synthesized CGFe3O4NPs: (a) micrographs display well dispersed spherical CGFe3O4NPs with an average size of (b) EDAX analysis of CGFe3O4NPs.
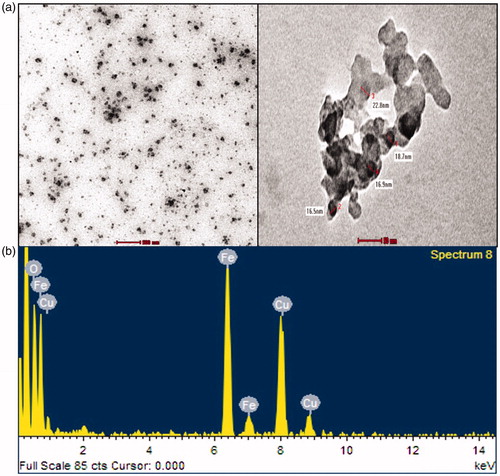
Interestingly, the magnetic properties of the NPs were not damaged by the plant biomolecules, in contrast they protect them from intraparticle interaction and make the properties more stable against the environment. Magnetic parameters like saturation magnetization (MS), coercivity (HC) and remanence magnetization (MR) have been evaluated. At room temperature, the synthesized CGFe3O4NPs exhibit superparamagnetism with negligible hysteresis loop (), coercivity (HC) of 48 Oe and MR of 0.1 emu g−1, and MS of 0.11 emu g−1, respectively. Our result highly corroborates with the previous report [Citation42] where pristine Fe3O4 exhibits superparamagnetic behaviour at room temperature, it was also hypothesized that the low MS value could be due to crystalline defects and alignment of magnetic moment in nanocrystals. On the contrary, at low temperature, synthesized CGFe3O4NPs show transitional behaviour from superparamagnetic to ferromagnetic with increased symmetric hysteresis loop. The increased magnetic behaviour and translation of superparamagnetic to ferromagnetic was mainly because of the long-range magnetic dipole–dipole interaction between the particles at low temperature [Citation10].
In vitro anticancer activity
MTT assay was carried out to assess the cytotoxic ability of CGFE and CGFe3O4NPs on HepG2 cells and to identify the IC50 values at 24 h. The present findings revealed that both CGFE and CGFe3O4NPs significantly inhibited the growth of HepG2 cells in a dose-dependent manner. The IC50 values of CGFE and CGFe3O4NPs were 120 and 44.51 μg ml−1 for a 24 h treatment period, respectively (). Cell viability assay clarifies that the toxicity of NPs mainly depends on their surface morphology and size. Taken together, polyol adsorbed Fe3O4NPs surface may offer a synergistic cytotoxicity effect against the treated human hepatocellular carcinoma cells (HepG2).
Figure 7. In vitro anticancer activity of CGFE and CGFe3O4NPs against human hepatocellular carcinoma cells (HepG2) cells: (a) cell viability assay, (b) phase contrast images show the gross cytomorphological changes and AO-EB staining displays the CGFe3O4NPs induced apoptotic effect in treated HepG2 cells (A-Control, B-CGFE and C-CGFe3O4NPs) and (c) haemocompatibility assay of CGFE and CGFe3O4NPs.
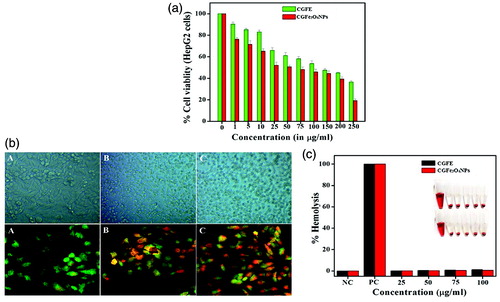
As described in many earlier studies, nanoscale materials were easily internalized by cells through either fluid phase pinocytosis or clathrin-mediated endocytosis [Citation43]. Also, it is now well established that the enhanced permeation retention (EPR), leaky vasculature and poor lymphatic drainage of cancer cells easily let the intravenously injected NP systems extravasate and preferentially accumulate in the tumour tissue [Citation44]. Isatin (1H-indole-2,3-dione), an endogenous compound isolated from the fruits of C. guianensis Aubl. possesses a wide range of biological activities such as anxiogenic, sedative, anticonvulsant activities and also act as a potent antagonist agent [Citation45]. Derivatives of isatin are known to have cytotoxicity against human carcinoma cell lines [Citation46]. We found that the active constituents of CGFE were being functionalized with Fe3O4NPs, which was confirmed by FT-IR and XPS analysis.
The first and most readily noticeable effect following exposure of cells to toxic materials was the alteration in cell shape or morphology in a monolayer culture. Microscopic observations of treated cells showed distinct morphological changes indicating unhealthy cells, whereas the control appeared normal. Diverse morphological alterations were observed in CGFE and CGFe3O4NPs treated HepG2 cells, however no such effects were seen in untreated control cells. It was also shown that the morphological variations observed such as loss of membrane integrity, inhibition of cell growth, cytoplasmic condensation and cell clumping () results indicate that the CGFE and CGFe3O4NPs treated cells undergo cell death, whereas the non-treated cells were active.
Initially CGFE and CGFe3O4NPs induced apoptosis was noticed with the morphological changes in the cell shape and chromatin condensation. The ability of CGFE and CGFe3O4NPs to induce apoptosis was determined by AO/EtBr staining method. Fluorescence microscopic observations showed viable (light green), early apoptotic (bright green fluorescence and condensed chromatin), late apoptotic cells (orange fluorescence) and nonviable cells (red coloured fluorescence) (). Additionally, we also observed that CGFE and CGFe3O4NPs treated HepG2 cells showed different characteristic features like condensed nuclei, membrane blebbing and apoptotic bodies of nuclear morphology whereas the untreated control cells remain intact. The above results strongly support that the formulated CGFe3O4NPs trigger HepG2 cell death via caspase–cascade mediated mitochondrial dysfunction and apoptosis [Citation47].
Haemocompatibility assay
Moreover, the haemocompatibility assay of synthesized CGFe3O4NPs and CGFE was evaluated upon calculating the damage to the human RBCs. The results showed that the CGFe3O4NPs exhibit comparatively lower red haemoglobin release than crude CFGE (). As per the result, compared to the positive control (Triton-1X) haemolytic activity of NPs was less considerable, implying its safe nature in chemotherapeutic application. The mechanisms of direct haemolytic activity for different toxic agents were found to be non-specific. Our results demonstrated that the active biocompounds capped on the surface of CGFe3O4NPs largely strengthen its biocompatibility. Many earlier studies have proved that the surface passivation of nanomaterials with active biomolecules will improve their biocompatibility [Citation48]. Eventually, this can be easily used for different pharmacological applications, including drug release. According to International Organization for Standardization/Technical Report 7406, for bio-based materials the haemolytic permissible level was fixed as less than 5% [Citation49]. The exposure of phenol functionalized nanomaterials shows meager level of haemolysis, revealing their biocompatibility and suitability for clinical trials.
Antibacterial activity
The bacterial culture plates were impregnated with CGFe3O4NPs loaded discs showing different levels of zone of inhibition in dose-dependent manner (). CGFe3O4NPs intrinsically exhibit highest level zone of inhibition against gram negative E. coli MTCC2939, S. typhi MTCC3917 and K. penumoniae MTCC 530 than gram positive S. aureus MTCC 96. This difference can be attributed to differences in cell wall structure inherent in gram-negative and gram-positive bacteria. Gram-positive and gram-negative bacteria have similar internal, but very different external structures. Gram-positive bacterial cells possess thick peptidoglycan layer that contains teichoic and lipoteichoic acids whereas gram-negative bacteria have thin peptidoglycan layer and an outer membrane that contains lipopolysaccharide, phospholipids and proteins [Citation50].
Figure 8. Bactericidal effect of CGFe3O4NPs: (a) disc diffusion assay shows clear zone of inhibition in dose-dependent manner against gram negative: (1) E. coli MTCC 1687, (2) S. typhi MTCC 3917, (3) K. pneumonia MTCC 530 and gram positive and (4) S. aureus MTCC 96(A- Positive control, B,C & D-25,50 &75?g/ml of CGFe3O4NPs) (b) Zone of inhibition of synthesized CGFe3O4NPs against tested bacterial pathogens.
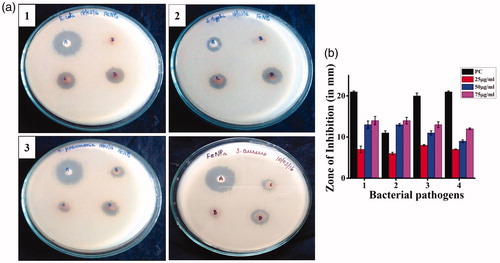
This gives a clear view on the bactericidal effect of synthesized CGFe3O4NPs which was totally relying on cell wall thickness, morphology of the cell envelope and resistance of outer membrane to the ROS produced by the metal ions. However, the antimicrobial activity of nanoparticles was explained by several mechanisms such as cell membrane damage, generation of reactive oxygen species, release of toxic metals and DNA damage. When these nanoparticles enter the bacterial cell and interact with sulphur containing proteins of cytoplasm, ribosomes and phosphorus containing DNA, they disrupt the respiratory chain and cell division [Citation51]. Internalization may result in either cell rupture and exocytosis or intercalation with DNA/inactivation of key enzymes, thereby, causing cell damage. Further studies are required to understand the exact mode of action of these nanomaterials and the mechanism of growth inhibition in bacterial cells [Citation52].
Conclusions
In conclusion, we have successfully developed a facile, green chemistry and an economically viable route to fabricate multifunctional magnetic Fe3O4 nanoparticles. The fabricated nanomaterials were well-characterized with different exclusive instrumentation, which demonstrated the generation of highly stable, crystalline, spherical-shaped magnetic Fe3O4 in co-ordination with functional groups of the active biocompounds. Furthermore, in vitro anticancer studies show that the synthesized CGFe3O4NPs induce significantly enhanced cytotoxicity effect at a minimal dosage of 44.5 μg ml−1 comparatively with CGFE 120 μg ml−1 against HepG2 cells. In comparison with crude CGFE, the synthesized CGFe3O4NPs possess a stupendous bactericidal effect against different bacterial human pathogens. Last but not least, the synthesized CGFe3O4NPs showed excellent magnetic properties and biocompatibility which let them to explore for MRI and their serum stability encourages further in vivo preclinical studies. Overall, the magnetic CGFe3O4NPs could be a prominent multifunctional nanomaterial for concurrent theranostics, drug delivery and image guided real time therapies to treat human cancer and other infectious diseases.
Disclosure statement
No potential conflict of interest was reported by the author(s).
References
- Mahdavi M, Ahmad MB, Haron MJ, et al. Synthesis, surface modification and characterization of biocompatible magnetic iron oxide nanoparticles for biomedical applications. Molecules. 2013;18:7533–7548.
- Gao Z, Liu X, Deng G, et al. Fe3O4@mSiO2-FA-CuS-PEG nanocomposites for magnetic resonance imaging and targeted chemo-photothermal synergistic therapy of cancer cells. Dalton Trans. 2016;45:13456–13465.
- Huang S, Li C, Cheng Z, et al. Magnetic Fe3O4@mesoporous silica composites for drug delivery and bioadsorption. J Colloid Interface Sci. 2012;376:312–321.
- Sadat ME, Baghbador MK, Dunn AW, et al. Photoluminescence and photothermal effect of Fe3O4 nanoparticles for medical imaging and therapy. Appl Phys Lett. 2014;105:1–5.
- Maleki-Ghaleh H, Aghaie E, Nadernezhad A, et al. Influence of Fe3O4 nanoparticles in hydroxyapatite scaffolds on proliferation of primary human fibroblast cells. J Mater Eng Perform. 2016;25:2331–2339.
- Jiang P, Zhang Y, Zhu C, et al. Fe3O4/BSA particles induce osteogenic differentiation of mesenchymal stem cell under static magnetic field. Acta Biomater. 2016;46:141–150.
- Jiang QL, Zheng SW, Hong RY, et al. Folic acid-conjugated Fe3O4 magnetic nanoparticles for hyperthermia and MRI in vitro and in vivo. Appl Surf Sci. 2014;307:224–233.
- Barick KC, Singh S, Bahadur D, et al. Carboxyl decorated Fe3O4 nanoparticles for MRI diagnosis and localized hyperthermia. J Colloid Interface Sci. 2014;418:120–125.
- Lin LS, Cong ZX, Cao JB, et al. Multifunctional Fe3O4@polydopamine core–shell nanocomposites for intracellular mRNA detection and imaging-guided photothermal therapy. ACS Nano. 2014;8:3876–3883.
- Ling Y, Wei K, Luo Y, et al. Dual docetaxel/superparamagnetic iron oxide loaded nanoparticles for both targeting magnetic resonance imaging and cancer therapy. Biomaterials. 2011;32:7139–7150.
- Wu W, Wu Z, Yu T, et al. Recent progress on magnetic iron oxide nanoparticles: synthesis, surface functional strategies and biomedical applications. Sci Technol Adv Mater. 2015;16:1–43.
- Hussain I, Singh NB, Singh A, et al. Green synthesis of nanoparticles and its potential application. Biotechnol Lett. 2016;38:545–560.
- Kharissova OV, Dias HR, Kharisov BI, et al. The greener synthesis of nanoparticles. Trends Biotechnol. 2013;31:240–248.
- Sankar R, Maheswari R, Karthik S, et al. Anticancer activity of Ficus religiosa engineered copper oxide nanoparticles. Mater Sci Eng C. 2014;44:234–239.
- Narayanan KB, Sakthivel N. Green synthesis of biogenic metal nanoparticles by terrestrial and aquatic phototrophic and heterotrophic eukaryotes and biocompatible agents. Adv Colloid Interface Sci. 2011;169:59–79.
- Mittal AK, Chisti Y, Banerjee U. Synthesis of metallic nanoparticles using plant extracts. Biotechnol Adv. 2013;31:346–356.
- Mohanty S, Mishra S, Jena P, et al. An investigation on the antibacterial, cytotoxic, and antibiofilm efficacy of starch-stabilized silver nanoparticles. Nanomedicine. 2012;8:916–924.
- Ferlay J, Soerjomataram I, Dikshit R, et al. Cancer incidence and mortality worldwide: sources, methods and major patterns in GLOBOCAN 2012. Int J Cancer. 2015;136:359–386.
- Misra R, Acharya S, Sahoo SK. Cancer nanotechnology: application of nanotechnology in cancer therapy. Drug Discov Today. 2010;15:842–850.
- Kelkar SS, Reineke TM. Theranostics: combining imaging and therapy. Bioconjug Chem. 2011;22:1879–1903.
- Sathishkumar G, Bharti R, Jha PK, et al. Dietary flavone chrysin (5, 7-dihydroxyflavone ChR) functionalized highly-stable metal nanoformulations for improved anticancer applications. RSC Adv. 2015;109:89869–89878.
- Peng J, Qi T, Liao J, et al. Mesoporous magnetic gold “nanoclusters” as theranostic carrier for chemo-photothermal co-therapy of breast cancer. Theranostics. 2014;4:678–692.
- Al-Dhabi NA, Balachandran C, Raj MK, et al. Antimicrobial, antimycobacterial and antibiofilm properties of Couroupita guianensis Aubl. fruit extract. BMC Complement Altern Med. 2012;12:1–8.
- Kulkarni M, Wakade A, Ambaye R, et al. Phytochemical and pharmacological studies of the leaves of Couroupita guianensis Aubl. Pharmacology. 2011;3:809–814.
- Vimala RT, Sathishkumar G, Sivaramakrishnan S. Optimization of reaction conditions to fabricate nano-silver using Couroupita guianensis Aubl. (leaf & fruit) and its enhanced larvicidal effect. Spectrochim Acta A Mol Biomol Spectrosc. 2015;135:110–115.
- Mosmann T. Rapid colorimetric assay for cellular growth and survival: application to proliferation and cytotoxicity assays. J Immunol Methods. 1983;65:55–63.
- Sathishkumar G, Rajkuberan C, Manikandan K, et al. Facile biosynthesis of antimicrobial zinc oxide (ZnO) nanoflakes using leaf extract of Couroupita guianensis Aubl. Mater Lett. 2017;188:383–386.
- Jha AK, Prasad K, Prasad K, et al. Plant system: nature’s nanofactory. Colloids Surf B Biointerfaces. 2009;73:219–223.
- Venkateswarlu S, Kumar BN, Prasad CH, et al. Bio-inspired green synthesis of Fe3O4 spherical magnetic nanoparticles using Syzygium cumini seed extract. Phys B: Condens Matter. 2014;449:67–71.
- Zhang Q, Wang C, Qiao L, et al. Superparamagnetic iron oxide nanoparticles coated with a folate-conjugated polymer. J Mater Chem. 2009;19:8393–8402.
- Cai Y, Shen Y, Xie A, et al. Green synthesis of soya bean sprouts-mediated superparamagnetic Fe3O4 nanoparticles. J Magn Magn Mater. 2010;322:2938–2943.
- Demir A, Topkaya R, Baykal A. Green synthesis of superparamagnetic Fe3O4 nanoparticles with maltose: its magnetic investigation. Polyhedron. 2013;65:282–287.
- Lu W, Shen Y, Xie A, et al. Green synthesis and characterization of superparamagnetic Fe3O4 nanoparticles. J Magn Magn Mater. 2010;322:1828–1833.
- Yew YP, Shameli K, Miyake M, et al. Green synthesis of magnetite (Fe3O4) nanoparticles using seaweed (Kappaphycus alvarezii) extract. Nanoscale Res Lett. 2016;11:1–7.
- Tuo Y, Liu G, Dong B, et al. Microbial synthesis of Pd/Fe3O4, Au/Fe3O4 and PdAu/Fe3O4 nanocomposites for catalytic reduction of nitroaromatic compounds. Sci Rep. 2015;5:13515.
- Cheng KK, Chan PS, Fan S, et al. Curcumin-conjugated magnetic nanoparticles for detecting amyloid plaques in Alzheimer's disease mice using magnetic resonance imaging (MRI). Biomaterials. 2015;44:155–172.
- Silva VA, Andrade PL, Silva MP, et al. Synthesis and characterization of Fe3O4 nanoparticles coated with fucan polysaccharides. J Magn Magn Mater. 2013;343:138–143.
- Cheng J, Tan G, Li W, et al. Preparation, characterization and in vitro photodynamic therapy of a pyropheophorbide-a-conjugated Fe3O4 multifunctional magnetofluorescence photosensitizer. RSC Adv. 2016;6:37610–37620.
- Banerji B, Pramanik SK, Mandal S, et al. Synthesis, characterization and cytotoxicity study of magnetic (Fe3O4) nanoparticles and their drug conjugate. RSC Adv. 2012;2:2493–2497.
- Marin T, Montoya P, Arnache O, et al. Influence of surface treatment on magnetic properties of Fe3O4 nanoparticles synthesized by electrochemical method. J Phys Chem B. 2016;120:6634–6645.
- Latorre-Sanchez M, Primo A, Garcia H. Green synthesis of Fe3O4 nanoparticles embedded in a porous carbon matrix and its use as anode material in Li-ion batteries. J Mater Chem. 2012;22:21373–21375.
- Jayabharathi J, Ramanathan P, Thanikachalam V, et al. Optical and theoretical studies on Fe3O4–imidazole nanocomposite and clusters. New J Chem. 2015;39:3801–3812.
- Zhang S, Gao H, Bao G. Physical principles of nanoparticle cellular endocytosis. ACS Nano. 2015;9:8655–8671.
- Jain TK, Morales MA, Sahoo SK, et al. Iron oxide nanoparticles for sustained delivery of anticancer agents. Mol Pharm. 2005;2:194–205.
- Premanathan M, Radhakrishnan S, Kulangiappar K, et al. Antioxidant & anticancer activities of isatin (1H-indole-2,3-dione), isolated from the flowers of Couroupita guianensis Aubl. Indian J Med Res. 2012;136:822–826.
- Vine KL, Matesic L, Locke JM, et al. Cytotoxic and anticancer activities of isatin and its derivatives: a comprehensive review from 2000–2008. Anticancer Agents Med Chem. 2009;9:397–414.
- Xue Y, Zhang T, Zhang B, et al. Cytotoxicity and apoptosis induced by silver nanoparticles in human liver HepG2 cells in different dispersion media. J Appl Toxicol. 2016;36:352–360.
- Kumar KP, Paul W, Sharma CP. Green synthesis of gold nanoparticles with Zingiber officinale extract: characterization and blood compatibility. Process Biochem. 2011;46:2007–2013.
- Ruden S, Hilpert K, Berditsch M, et al. Synergistic interaction between silver nanoparticles and membrane-permeabilizing antimicrobial peptides. Antimicrob Agents Chemother. 2009;53:3538–3540.
- Naeimi H, Nazifi ZS, Amininezhad SM. Preparation of Fe3O4 encapsulated-silica sulfonic acid nanoparticles and study of their in vitro antimicrobial activity. J Photochem Photobiol B Biol. 2015;149:180–188.
- Arakha M, Pal S, Samantarrai D, et al. Antimicrobial activity of iron oxide nanoparticle upon modulation of nanoparticle-bacteria interface. Sci Rep. 2015;5:14813.
- Sangeetha J, Philip J. Synthesis, characterization and antimicrobial property of Fe3O4-Cys-HNQ nanocomplex, with l-cysteine molecule as a linker. RSC Adv. 2013;3:8047–8057.