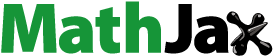
Abstract
A drug delivery system based on dual responsive units was developed. An appealing pH- and thermo-responsive triblock terpolymer as the drug carrier was synthesized by RAFT polymerization of N-isopropyl acrylamide and methacrylic acid monomers using PEG-RAFT agent. The Fe3O4 magnetic nanoparticles were synthesized by co-precipitation of Fe salts. Synthesized samples were characterized by FT-IR, XRD, GPC, SEM and TEM. The dual responsive behaviour and self-assembly of the triblock terpolymers in aqueous solution were investigated using UV–vis transmittance and DLS. Based on the results of DLS and TEM, the average size of micelles was 170, 125 and 30 nm. The triblock terpolymer was used as a chemotherapy drug carrier and doxorubicin as a model drug. The release rate of the drug at two different temperatures (37 °C and 42 °C) and pHs (5.8 and 7.4) was studied. The in vitro cytotoxicity assay of free doxorubicin and drug-loaded magnetic nanoparticles was studied. The MTT assay exhibited that these polymers are biocompatible and no toxicity. As well, IC50 of the DOX-loaded triblock terpolymer in MTT test demonstrated that these systems could be suitable for the treatment of cancer.
Introduction
Cancer is one of the most incurable human diseases in worldwide. There is a global effort to make progress in new technologies. They are trying to overcome the disadvantage of chemotherapy and effective destruction of tumour tissue without damaging the healthy body. Two important targets in cancer treatment are to diagnosis cancer in the early stages, deliver chemotherapy drug to the special sites with reducing of adverse effects and the increase in its efficacy. Doxorubicin (DOX) is the most common chemotherapeutic drugs that are an anthracycline antibiotic [Citation1]. DOX indicates antitumor activity through inhibition of topoisomerase II [Citation2]; as a result, it inhibits the replication of DNA and proteins synthesis [Citation3,Citation4]. In addition, DOX makes a complex with DNA of cells through penetration between base pairs. Besides that, the medical use of DOX is limited by reason of the very serious side effects on healthy tissue [Citation5]. In this study, because of its low cost, unique physicochemical and antitumor properties, doxorubicin was chosen as a chemotherapy drug model.
Recently, controlled drug delivery systems such as nanocarriers have attracted much attention because of its capability in reducing the serious side effects of the chemotherapy and increasing therapeutic efficacy [Citation6–8]. Nanocarriers may be designed from types of organic and inorganic materials such as polymers, lipid, dendrimer, or gold, iron oxide, ZnO, quantum dots, etc. [Citation9–14]. In medicinal applications such as imaging, thermotherapy of cancer and controlled drug delivery, Fe3O4 nanoparticles have been studied widely due to their distinctive physicochemical properties. Because it increases the therapeutic effects and reduces the adverse side effects of the drug [Citation15,Citation16].
Fe3O4 nanoparticles designed for medical applications are necessary to be non-toxic and with narrow size distribution, good colloidal stability under physiological conditions, and prolonged circulation in the bloodstream [Citation17]. Usually, Fe3O4 nanoparticles are trapped in a polymer matrix, which allows the coupling of an anticancer drug by electrostatic interaction to the polymer, or by dispersion in the polymeric matrix. One of the most important methods of surface modification is using biocompatible polymers or stimuli–responsive block copolymers [Citation18–21].
The stimuli–responsive block copolymer has attracted much attention because of their unique properties in response to environmental changes and the self-assembly behaviour [Citation22–26]. These stimuli–responsive block copolymers can release the trapped drug in their when a stimulus is present in the surroundings [Citation27–29]. Therefore, these micelles are perfectly suitable for the biomedical applications such as drug delivery. It was recently reported that pH of blood and normal tissue is around 7.4 and pH of the tumours is lower than it, also, the tumour temperature is slightly higher than body temperature [Citation30]. Thus, the pH- and thermoresponsive block copolymers are more attention because of changes of temperature and pH in tumour tissue for controlled release of the drug. Poly-(methacrylic acid) (PMA) and poly(N-isopropyl acrylamide) (PNIPAM) are usual polymers that can respond to changes in pH and temperature [Citation31].
One potential problem using PNIPAM in biomedical applications as a responsive polymer is that its lower critical solution temperature (LCST), hence, the cloud point (CP) of PNIPAM aqueous solutions can be easily modified by copolymerization with different monomers or by the addition of salts or surfactants [Citation32–34].
Polyethylene glycol (PEG) as usual hydrophilic polymers has numerous biomedical and pharmaceutical applications because of its specific properties such as non-toxicity, biocompatibility, biodegradability, and resistance to recognition by the immune system. Therefore, polymeric micelles consisting of PEG have also been attracted. Also, the neutral nature of the PEG could increase the efficiency of the particle internalization by target cells [Citation35,Citation36]. Commonly, it can be grafted onto a nanoparticle surface to form a hydrophilic outer layer that reduces protein adsorption and aggregation of nanoparticles. Targeting is to achieving effective therapy and reducing side effects of chemotherapy and increasing the chances of successful treatment.
In recent years, well-defined block copolymers have been efficiently synthesized using the controlled and living radical polymerizations [Citation37]. Living radical polymerizations produce polymers with controlled molecular weights and narrow molecular weight distributions. Unlike many other controlled radical techniques [Citation38], RAFT demonstrates the most useful and facile method. RAFT polymerization does not need any distinctive conditions and may be carried out in the same way as conventional free radical polymerization. It is able to polymerize a wide range of monomers such as the acidic monomers, acrylates, acrylamides in polar solvents such as ethanol [Citation39], Therefore, this method is the most broadly employed method to prepare water soluble polymers [Citation40].
The purpose of this study was to synthesis and evaluation of Poly [(PMA-PNIPAM)m-b-PEG-b-(PNIPAM-PMA)m]/Fe3O4 nanocomposite as nanocarriers for encapsulated and controlled release of doxorubicin. DOX has been incorporated into the polymeric micelle by electrostatic interaction to decrease its toxicity and improvement therapeutic efficacy. The physicochemical properties of the triblock terpolymer nanoparticles and the release of drug at different temperature and pH levels and cytotoxicity assessment were studied to determine the relation between pH/temperature and drug release, and the importance of designing an intelligent polymer in drug delivery system. The RAFT polymerization was used to produce controlled, narrow molecular weight distributions.
Experimental
Material and methods
PEG, biological grade, with a molecular weight (MW) of 4000 was supplied by the Sigma-Aldrich (St. Louis, MO). N-Isopropyl-acrylamide (Sigma-Aldrich, St. Louis, MO, >99%, NIPAM) was recrystallized from n-hexane. Methacrylic acid (Merck, Kenilworth, NJ, >99%, methacrylic acid (MA)) was distilled under vacuum before use. Azobisisobutyronitrile (AIBN, Merck, Kenilworth, NJ) is a free radical initiator, which, was recrystallized from methanol former to use. Tetrahydrofuran (Scharlau, Hamburg, Germany, >99%, THF), n-hexane (Merck, Kenilworth, NJ, >99%), dimethyl formamide (Sigma-Aldrich, St. Louis, MO, 99%, DMF), dichloromethane (Chem-Lab, Fishers, IN, >99.8%, DCM), chloroform (Mojallali, Tehran, Iran, >99%), ethanol (Merck, Kenilworth, NJ, 99.9%), methanol (Merck, Kenilworth, NJ, 99.9%) were used as purchased without further purification and used as received. 4-Cyano-4-((thiobenzoyl)sulfanyl)pentanoic acid as an RAFT agent was supplied by the Sigma-Aldrich (St. Louis, MO). Foetal bovine serum (FBS) (Gibco, Invitrogen, Paisley, UK), RPMI 1640, TRIzol reagent, Trypsin-EDTA and antibiotics were purchased from Invitrogen (Paisley, UK). (3-(4,5-Dimethylthiazol-2-yl)-2,5-diphenyl tetrazolium bromide (Sigma-Aldrich, St. Louis, MO, MTT) were purchased and used without purification. Lung cancer cell was prepared from Pasteur Institute Cell Bank of Iran. Doxorubicin hydrochloride (Sigma-Aldrich, St. Louis, MO, DOX) were used without purification.
Characterization and measurements
FT-IR characterization was recorded using Shimadzu FT-IR-8101 M spectrometer (Shimadzu, Kyoto, Japan). The entire spectrum was measured at a resolution 4 cm−1 and scanned from 4000 to 400 cm−1. The molecular weight and its distribution (PDI = Mw/Mn) of the triblock terpolymer were determined by gel permeation chromatography (GPC, Waters, Ventura, CA) equipped with a series of styragel columns. N,N-Dimethylformamide (DMF) was used as an eluent at a flow rate of 1 ml/min and pump pressure maintained was 3 mPa at 25 °C. UV–vis spectroscopy was performed using 1650 PC UV–vis spectrophotometer (Shimadzu, Kyoto, Japan). The pH-sensitivity and the lower critical solution temperature (LCST) were determined by UV–vis spectroscopy at a wavelength of 495 nm. The temperature of the sample cells was increased from 25 °C to 75 °C. Measurement of the average diameter of nanoparticles was performed in deionized water by the dynamic light scattering technology (DLS) (Nanotrac. 150, Microtrac Inc., Montgomeryville, PA). The size measurement was performed at acidic medium, room temperature (25 °C, pH 5) and natural medium, high temperature (58 °C, pH 7). The morphology of the polymeric nanoparticles was observed using a scanning electron microscopy (SEM, Leo Electron Microscopy Ltd, Cambridge, UK) and transmission electron microscopy (TEM, PHILIPS CM10 TEM EPSON HP8300, Philips, Andover, MA)
Synthesis of PEG-RAFT agent
The synthesis of the macro PEG-RAFT agent, chain transfer agent (CTA), was performed by the esterification method. Briefly, RAFT agent (0.4 g, 1.5 mmol) and dihydroxyl PEG (2 g, 0.5 mmol) were dissolved in 35 ml of DCM in a 250 ml round bottom flask equipped with a magnetic stirrer. Then, it was placed in an ice bath (0 °C); Next, DCC (0.25 g, 1.2 mmol) and DMAP (0.015 g, 0.12 mmol) were added. After 30 min of stirring at 0 °C, the reaction temperature was increased to 25 °C. The reaction mixture stirred for 2 d. The precipitated dicyclohexylurea was separated by filtration. The mixture was concentrated by rotary evaporator and then, was precipitated into excess diethyl ether to remove the unreacted RAFT agent. PEG-RAFT agent with bright yellow colour was achieved by filtering and drying under vacuum at room temperature for 24 h (1.7 g, 85%) (Scheme 1(I)).
Synthesis of triblock terpolymer by RAFT polymerization
PEG-RAFT agent was used as CTA in the RAFT polymerization of N-isopropyl acrylamide and MA monomers. For synthesis of poly [(PMA-PNIPAM)m-b-PEG-b-(PNIPAM-PMA)m] triblock terpolymer, PEG-RAFT agent (0.45 g, 0.11 mmol), NIPAM (2.0 g, 17.7 mmol), MA (1.5 g, 17.7 mmol) and AIBN (3.6 mg, 0.022 mmol) were dissolved in THF (4 ml) in a 100 ml round bottom flask. The reaction flask temperature is raised to 70 °C and stirred for 24 h, after deoxygenation of the solution. After 24 h, the reaction flask was cooled to ambient temperature and was opened to air in order to stop the polymerization reaction. The reaction mixture was decanted into excess diethyl ether. The produce was purified twice by dissolution/precipitation with dichloromethane/diethyl ether and then dried in a vacuum for 24 h. (2.34 g, 82%) (Scheme 1 (II)).
Preparation of the Fe3O4 nanoparticles
Fe3O4 nanoparticles were synthesized without any stabilizer according to the following methods [Citation41]. Briefly, 0.6 M of FeCl3.6H2O and 0.3 M of FeCl2.4H2O with a ratio of 2:1 were dissolved in 20 ml of 0.4 M HCl. The Fe salts solution was added dropwise into 200 ml of alkaline solution with pH >12 under stirring vigorously in an inert atmosphere. The obtained colloid solution was centrifuged at 10,000 rpm, washed with deionized water and dried under vacuum for 6 h at 60 °C. A black fine powder (Fe3O4) was achieved.
Preparation of doxorubicin hydrochloride-loaded nanocomposites
Doxorubicin was used as a model drug in drug loading and release tests. The DOX-loaded poly [(PMA-PNIPAM)m-b-PEG-b-(PNIPAM-PMA)m]/Fe3O4 were prepared at 25 °C and pH 7.5 by adding a specific volume of DOX solution dropwise into polymer and Fe3O4 solution under vigorous stirring. Hence, 200 mg of poly [(PMA-PNIPAM)m-b-PEG-b-(PNIPAM-PMA)m] triblock terpolymer and 5 mg of Fe3O4 nanoparticles were dispersed in phosphate buffer solution. Then, 20 mg of DOX was added dropwise to the solution, with ratios of 40:1:4, respectively. The solution was stirred at 4 °C for 2 d in the dark to reach the maximum loading content. Finally, to remove the unloaded DOX, the solution was centrifuged for 15 min at a rate 12,000 rpm.
The loading efficiency of doxorubicin capsulated inside the magnetic polymer nanoparticles was estimated by the dividing the capsulated DOX and the total DOX used in the aqueous phase, using the following formula:
In vitro drug release
To study the drug release of synthesized nanocomposites were performed in phosphate buffered saline (PBS). The drug release process was carried out at pH 7.4 and 5.8 and two different temperatures 37 and 42 °C (representing the physiological body and tumour tissue temperature, respectively).At this step, 30 mg of the drug-loaded magnetic nanocomposite was suspended in a specified volume of buffer solution (pH =7.4 physiological body and 5.8 tumour tissue pH), for study pH-dependent and pH sensitivity of drug release kinetics.
Test tubes were placed in a shaker incubator and were shaken gently. At selected timescales, test tubes were centrifuged at 12,000 rpm for 5 min, then 2 ml of the supernatant was withdrawn and similar volume was reconstituted by adding 2 ml of fresh buffered solution to every sample. Next, the samples were investigated using UV–vis spectrophotometry to estimate the amount of doxorubicin released at a wavelength of 480 nm for doxorubicin measurement. The releasing content was calculated by the following formula:
Cell culture and in vitro cytotoxicity assay
The antitumor activity of the triblock terpolymer was measured by determining the lung cancer cell (A549) viability by 3-(4,5-dimethylthiazol-2-Yl)-2,5-diphenyltetrazolium bromide (MTT) assays in 24, 48 and 72 h. A549 cell lines were received from NCBI (National Cell Bank of Iran, Pasteur Institute) as the target cells. The cells were grown in RPMI 1640 culture medium containing 10% foetal bovine serum (FBS), 2 mg/ml sodium bicarbonate, 0.05 mg/ml penicillin, 0.05 mg/ml streptomycin and incubated at 37 °C with humidified air containing 5% CO2. The cells were dissociated using 0.025% trypsin in PBS (pH 7.4) [Citation42,Citation43].
The cells were seeded in 96-well plates at 8000 cells per well in 200 ml of complete RPMI 1640 with 10% FBS, 0.05 mg/ml penicillin and 0.05 mg/ml streptomycin, and incubated at 37 °C in 5% CO2. the lung cancer cell (A549) were treated with varying concentrations of the triblock terpolymer, DOX-loaded magnetic nanocomposite and free DOX then incubated for 24, 48 and 72 h. Control experiments were performed using PBS instead of triblock terpolymer/Fe3O4/DOX or free DOX solutions. Then, the medium of all wells was removed carefully and 60 μl of MTT was added to each well and plate was incubated for 4 h in dark. After incubation, wells content were removed and 100 μl pure DMSO and 25 μl Sorensen's glycine buffer was added to wells to dissolve the formazan crystals. Immediately, the absorbance of each well was read at 570 nm by ELISA plate reader at a reference wavelength of 630 nm [Citation44]. The half-maximal inhibitory concentration (IC50) was determined as the DOX concentration that resulted in a 50% reduction in cell viability and was calculated using the following equation:
where ODsample is the absorbance intensity of the cells treated with the triblock terpolymer, and ODcontrol is that of the cells treated with phosphate buffer solution (PBS) instead.
Results and discussion
Characterization of the Fe3O4 nanoparticles
The Fe3O4 nanoparticle was synthesized by addition 1 to 2 of Fe salts to an alkaline solution (pH >12), in an inert atmosphere at room temperature. For characterization of iron oxide nanoparticles, the XRD and FT-IR spectrum was employed. The XRD spectrum of Fe3O4 is shown in . As can be observed the Fe3O4 nanoparticles have a higher and narrower diffraction peaks which indicate a good crystallization of Fe3O4 were achieved. The Fe3O4 nanoparticles have high purity because there is no additional peak. The average size of the Fe3O4 nanoparticles was determined to be 15 nm from the peak width at half maximum (from 311 reflections) and Debye Sherrer’s formula (Dc = kλ/b cosθ). The equation uses the reference peak width at angle θ, where λ is the X-ray wavelength (1.54060 A˚), b is the width of the XRD peak at half height and K is a shape factor. In the FT-IR spectrum (), the peak at 3417 cm−1 is attributed to the stretching vibrations of −OH or water molecule adsorbed onto the surface of the Fe3O4 nanoparticles, and the peak at 565 cm−1 is attributed to the stretching vibration of Fe-O.
Characterization of poly [(PMA-PNIPAM)m-b-PEG-b-(PNIPAM-PMA)m] triblock terpolymer
Dual responsive poly [(PMA-PNIPAM)m-b-PEG-b-(PNIPAM-PMA)m] triblock terpolymers were obtained by RAFT polymerization of N-isopropylacrylamide (NIPAM) and MA) monomers in two steps as shown in Scheme 1. FT-IR and GPC analysis were employed to characterize the prepared polymer.
FT-IR studies of triblock terpolymer
shows the FT-IR spectrum of the triblock terpolymer. In the first stage, the PEG-RAFT agent is prepared via the esterification of the terminal hydroxyl group of PEG with the carboxyl group of 4-cyano-4-((thiobenzoyl)sulfanyl)pentanoic acid as RAFT agent. As clear from , two strong absorption peaks at 2912 and 1114 cm−1 are related to a characteristic of PEG which attributed to the C–H and C–O stretching vibration and the absorption bands at 1467 and 1345 cm−1 belong to C–H bending vibration. The peak at 3400 cm−1 is associated with the terminal hydroxyl groups of PEG. As can be observed in , the stretching vibration peak of hydroxyl (–OH) in PEG-RAFT agent has been disappeared at the band 3400 cm−1, which shows the progress of the esterification reaction has been successfully done.
Figure 2. FT-IR spectrum of (a) dihydroxyl-terminated PEG (HO-PEG-OH), (b) PEG-RAFT agent, (c) poly [(PMA-PNIPAM)m-b-PEG-b-(PNIPAM-PMA)m] triblock terpolymer and (d) DOX-loaded triblock terpolymer.
![Figure 2. FT-IR spectrum of (a) dihydroxyl-terminated PEG (HO-PEG-OH), (b) PEG-RAFT agent, (c) poly [(PMA-PNIPAM)m-b-PEG-b-(PNIPAM-PMA)m] triblock terpolymer and (d) DOX-loaded triblock terpolymer.](/cms/asset/0c8836ac-e8d1-4fe9-85f7-e370b45da6ea/ianb_a_1360323_f0002_c.jpg)
The structure of the triblock terpolymer was identified by FT-IR and GPC analysis ( and Citation3). shows FT-IR spectrum of Poly [(PMA-PNIPAM)m-b-PEG-b-(PNIPAM-PMA)m] triblock terpolymer. It is clear from that the poly [(PMA-PNIPAM)m-b-PEG-b-(PNIPAM-PMA)m] demonstrate peaks characteristic of PMA, PNIPAM and PEG.
The stretching vibration at 1730 cm−1 is attributed to the C = O of the carbonyl group. The intense absorption bands between 960 cm−1 and 1400 cm−1 are related to PEG chain. The peak of –NH of PNIPAM at 3289 cm−1 and a new peak of the carbonyl group (–OH) became visible at 3440 cm−1 in the spectrum of poly [(PMA-PNIPAM)m-b-PEG-b-(PNIPAM-PMA)m], entirely the C–H stretching bands are detected at 2912 cm−1. All these signals prove that the poly [(PMA-PNIPAM)m-b-PEG-b-(PNIPAM-PMA)m] is prepared properly. Also, exhibits the absorbance of amide C = O groups in triblock terpolymer at 1670 cm−1 and the bending vibration of the amide N–H appeared at 1550 cm−1. These results show that the monomer of NIPAM and MAA were successfully polymerized by RAFT.
Gel permeation chromatography of triblock terpolymer
GPC analysis was performed to ascertain the molecular weights and molecular weight distributions of poly [(PMA-PNIPAM)m-b-PEG-b-(PNIPAM-PMA)m] triblock terpolymer. depicts the GPC curves of poly [(PMA-PNIPAM)m-b-PEG-b-(PNIPAM-PMA)m]. According to the analysis of GPC, the polydispersity index (PDI = Mw/Mn) values are 1.16 that indicates good control of the poly [(PMA-PNIPAM)m-b-PEG-b-(PNIPAM-PMA)m] triblock terpolymer during polymerization. It shows that the polymerization of poly [(PMA-PNIPAM)m-b-PEG-b-(PNIPAM-PMA)m] via RAFT is controlled and its PDI is almost close to 1. The living nature of the polymerization using the PEG-RAFT agent is confirmed by the results of GPC analysis. After extension of chain or polymerization reaction, the molecular weight grows up from 4000 (PEG) to 13,800 g/mol (triblock terpolymer), indicating that the monomer of NIPAM and MAA was successfully polymerized by RAFT.
Morphological of triblock terpolymer and Fe3O4 nanoparticles
Morphological studies of poly [(PMA-PNIPAM)m-b-PEG-b-(PNIPAM-PMA)m] triblock terpolymer micelles and Fe3O4 nanoparticles were done by TEM, SEM. The SEM images of pure Fe3O4 nanoparticles and poly [(PMA-PNIPAM)m-b-PEG-b-(PNIPAM-PMA)m] are shown in . As for being observed in , Fe3O4 nanoparticles were aggregated strongly, which was attributable to the nanoscale of the Fe3O4, and the average size of nanoparticles was estimated about 15–35 nm.
The surface morphology of the poly [(PMA-PNIPAM)m-b-PEG-b-(PNIPAM-PMA)m] triblock terpolymer is shown in . SEM image of poly [(PMA-PNIPAM)m-b-PEG-b-(PNIPAM-PMA)m] triblock terpolymer exhibits the nanoscale and irregularly structured morphology with an average diameter of 80–160 nm. This morphology is recommended that originated from the controlled growth of the PNIPAM and PMA from PEG-RAFT agent.
The morphology and the mean size distribution of Fe3O4 nanoparticles were determined by TEM (). reveals that pure Fe3O4 nanoparticles are spherical and having a uniform size about 20–35 nm. It presents the Fe3O4 particles that were synthesized by co-precipitation method that shows low dispersion with high aggregation because of the large specific surface area.
The TEM image () of poly [(PMA-PNIPAM)m-b-PEG-b-(PNIPAM-PMA)m] triblock terpolymer shows the self-assembly properties of polymeric micelles. The TEM images showed that well-defined spherical micelle aggregates the structure of the core/shell by self-assembly of poly [(PMA-PNIPAM)m-b-PEG-b-(PNIPAM-PMA)m] in an aqueous solution of pH =5 with the range of 25–70 nm (). In this case, PMA form cores of the micelle. It is known that size is dependent on environmental pH. The size of micelles increases with decreasing pH. Intra- and intermolecular hydrophobic interactions is formed due to protonation of polycarboxylate anions of PMA, in acidic solutions with pH < pKa [Citation45]. It was noted that the nanoparticles are of a sphere shape with a loose core–shell structure at room temperature and pH = 5.
Magnetism test
The magnetic properties of the nanoparticles and magnetic nanocomposite were studied by vibrating sample magnetometer at 25 °C. shows the magnetic curve of the Fe3O4 nanoparticles and Fe3O4/poly [(PMA-PNIPAM)m-b-PEG-b-(PNIPAM-PMA)m]/DOX nano-composite. The saturation magnetization was found to be 20 emu/g for doxorubicin-loaded Poly [(PMA-PNIPAM)m-b-PEG-b-(PNIPAM-PMA)m] magnetic nanocomposite, less than for the pure Fe3O4 nanoparticles (75 emu/g). This difference offers that a large amount of Fe3O4 was trapped by triblock terpolymer and doxorubicin. According to magnetism curve of the doxorubicin-loaded triblock terpolymer, magnetic nanoparticle is obvious that was separated easily by the magnetic field in the reaction medium.
Figure 5. Magnetic behaviour of (a) Fe3O4 nanoparticles and (b) poly [(PMA-PNIPAM)m-b-PEG-b-(PNIPAM-PMA)m]/Fe3O4/DOX magnetic nanocomposite.
![Figure 5. Magnetic behaviour of (a) Fe3O4 nanoparticles and (b) poly [(PMA-PNIPAM)m-b-PEG-b-(PNIPAM-PMA)m]/Fe3O4/DOX magnetic nanocomposite.](/cms/asset/3061ce5f-9a80-41e6-8631-84afeb4969de/ianb_a_1360323_f0005_c.jpg)
These magnetic properties are vital for application in the biomedical and bioengineering. The high saturation magnetization for the DOX composite nanoparticle can produce suitable heat in an alternative magnetic field that is essential for hyperthermia therapy.
Self-assembly behaviour of poly [(PMA-PNIPAM)m-b-PEG-b-(PNIPAM-PMA)m]
Change of the micelle arrangement and self-assembly behaviours of poly [(PMA-PNIPAM)m-b-PEG-b-(PNIPAM-PMA)m] triblock terpolymer caused by temperature and pH was studied with UV–vis spectroscopy and DLS analysis. This triblock terpolymer encompasses hydrophilic PEG block, thermoresponsive PNIPAM and pH-responsive PMA. So, it is expected that the triblock terpolymer is sensitive to temperature and pH. In this study, the PEG block can act as a steric stabilizer for the polymer and allows the formation of stable micellar at middle pH and temperatures. Due to the high compatibility of PEG in the bloodstream, it is used to prevent the kidney or hepatic removal and to lead to a prolonged circulation of the drug in the blood.
UV–vis spectroscopy measurement
The transmittance of poly [(PMA-PNIPAM)m-b-PEG-b-(PNIPAM-PMA)m] aqueous solutions (1 mg mL−1) at pH =7 was measured in the temperature range of 25–70 °C (). Below 58 °C, the PNIPAM segment is hydrophilic and the solution is transparent and above it, the expanded PNIPAM chains collapse to form the cores of the micelles. The 58 °C described as lower critical solution temperature (LCST). The PNIPAM chains collapse to form a nanoparticle core, while the PEG and PMA chains remain soluble and form a shell to stabilize the micelles. As can be observed in , the critical aggregation temperature of poly [(PMA-PNIPAM)m-b-PEG-b-(PNIPAM-PMA)m] is 58 °C, whereas LCST of the homo-PNIPAM is ∼32 °C. This temperature difference is attributed to the presence of hydrophilic polymethacrylic acid and PEG blocks in the synthesized terpolymer, because the MA and PEG are inherently more hydrophilic than PNIPAM [Citation46].
As mentioned above, the poly [(PMA-PNIPAM)m-b-PEG-b-(PNIPAM-PMA)m] exhibits pH-responsive property. demonstrates transmittance changes of the triblock terpolymer with changes pH. In this figure, it can be realized that the poly [(PMA-PNIPAM)m-b-PEG-b-(PNIPAM-PMA)m] shows a sharp transition behaviour at pH 5.7, which is very close to the pKa (about 5.6) of polymethacrylic acid. It has been proved that PMA has hypercoiled structure at pH <5.6. As a result, the polymer aggregates to form the micelle due to protonation of PMA and the creation of intramolecular hydrogen bonding [Citation47]. When pH of the solution is increased above 7, poly [(PMA-PNIPAM)m-b-PEG-b-(PNIPAM-PMA)m] chains are in extended chain conformation due to the electrostatic repulsion between the –COO− of carboxylate in PMA after [Citation48] and the solution becomes transparent, and the micelles are dissociated into unimers. The change in the pH would cause the conformation change of PMA segments from extended chain to hypercoil, and conversely, which in turn results in the aggregation of PMA chain segments into micelle cores [Citation49–59].
DLS experiments
The structure of pH- and thermo-responsive micelles from poly [(PMA-PNIPAM)m-b-PEG-b-(PNIPAM-PMA)m] triblock terpolymer is investigated by DLS experiment that was carried out in aqueous solution and various pH and temperature with a concentration of 200 mg L−1, as shown in .
shows the distributed sizes and DLS analysis of poly [(PMA-PNIPAM)m-b-PEG-b-(PNIPAM-PMA)m] triblock terpolymer nanoparticles and DOX-loaded triblock terpolymer at different conditions of temperature and pH. It was found that the triblock terpolymer nanoparticles showed narrowly distributed sizes at both pH = 5, 25 °C () and pH = 7, 60 °C (). The average size of poly [(PMA-PNIPAM)m-b-PEG-b-(PNIPAM-PMA)m] triblock terpolymer nanoparticles micelles at pH = 5, 25 °C, and pH = 7, 60 °C were obtained 170 nm (PDI = 0.61) and 125 nm (PDI = 0.64), respectively. These results are compatible with a higher degree of protonation of the PMA in water at pH < pKa, which leads to a stronger repulsive interaction between –COOH in PMA segment, also, H-bonding interactions between polar groups of PNIPAM and water disappears at higher temperatures of LCST (∼58 °C). Clearly, poly[(PMA-PNIPAM)m-b-PEG-b-(PNIPAM-PMA)m] triblock terpolymer induced by changing temperature or pH show micellar properties. In addition to, the average size of DOX-loaded triblock terpolymer magnetite nanocomposite was 210 nm in , which indicate the drug and Fe3O4 encapsulated in micelles resulting in the increase of micelle size than that of pure micelles.
Load and release of doxorubicin hydrochloride from magnetite nanocomposites
DOX molecule has an amino group with a pKa of 8.6 [Citation60], and the pKa of carboxylic groups of polymethacrylic acid is around 5.8 [Citation61]. At pH 7.2, the protonated amino nitrogen of DOX with the positive charge provided the driving force to attach with the negatively charged carboxylic acid groups, which led to the expansion of Fe3O4/DOX/poly [(PMA-PNIPAM)m-b-PEG-b-(PNIPAM-PMA)m] complex micelles by electrostatic interaction [Citation62]. The preparation of complex poly [(PMA-PNIPAM)m-b-PEG-b-(PNIPAM-PMA)m] and drug-loading capability depends on to a high extent on the interaction between blocks and incorporated drugs
Therefore, loaded DOX should form an electrostatic interaction with the nanocarriers, which was established by the much higher loading capacity of poly [(PMA-PNIPAM)m-b-PEG-b-(PNIPAM-PMA)m]-Fe3O4 nanoparticles. exhibits the FT-IR spectrum of the DOX-loaded triblock terpolymer. The appearance of the broad peak at 3400 cm−1 (–OH and –NH of doxorubicin), 1585 cm−1 (C = O, stretching of anthracene ring) and 1184 cm−1 (C–O, stretching vibration of doxorubicin), characteristic peaks of doxorubicin in the triblock terpolymer matrix spectrum shows the presence of doxorubicin in the polymer structure.
The drug content and release of the complex micelles was analyzed by measuring UV–vis absorbance at 485 nm. Encapsulation efficiency for doxorubicin encapsulated in the polymer was found to be 64.8%.
To study the potential application of poly [(PMA-PNIPAM)m-b-PEG-b-(PNIPAM-PMA)m] triblock terpolymer nanoparticles with pH- and thermo-sensitive groups including a chemotherapy drug, we have studied the drug release performance from this system under physiological conditions. The release rate experiments of the DOX-loaded magnetic nanocomposite in two different solutions PBS (pH = 5.8 and 7.4) were performed at 37 and 42 °C, representatively shown in . As can be understood from , the release rate of doxorubicin from the nanocomposite in pH = 7.4 is slower than its release rate in an acid solution (pH = 5.8), both at 37 °C and 42 °C. Under acidic conditions (pH < pKa), protonation of carboxylate anions in the PMA chain, leading to breaking of the electrostatic interaction between PMA and DOX, as a result, accelerated drug release from the magnetic nanocomposite. The release rate of drug depends not only on pH and temperature of the dissolution medium but also on the chemical structure of nanocomposite, drug–matrix interactions and physical factors such as diffusion [Citation63]. On the contrary, it was observed that the release of DOX from poly [(PMA-PNIPAM)m-b-PEG-b-(PNIPAM-PMA)m] triblock terpolymer nanocomposites are a pH- and thermo-dependent process, where the cumulative fraction of DOX release increased with decreasing pH and increasing temperature in PBS buffer. As well as, at the beginning, a marked jump in the amount of DOX released in the solution is visible. The maximum concentration of released DOX after 100 h at 37 °C is about 47% at pH 7.4 and 74% at pH = 5.8 and at 42 °C 68% at pH 7.4 and 84% at pH 5.8. Therefore, in the case of a mildly acidic environment, the amount of released DOX is relatively higher. The faster release rate was achieved at pH 5.8 due to the lower electrostatic binding compared to pH 7.4.
Figure 8. Release of DOX from triblock terpolymer (a) pH =5.8, T = 42 °C, (b) pH =5.8, T = 37 °C, (c) pH =7.4, T = 42 °C and (d) pH =7.4, T = 37 °C.
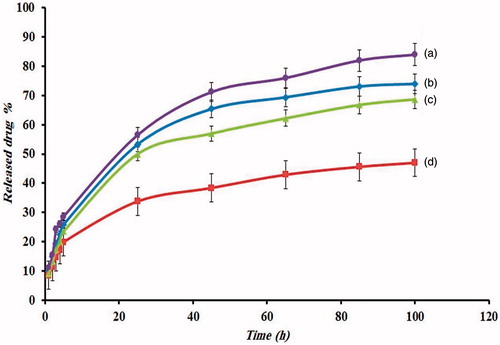
It is also shown that the release of drug at higher pH (7.4) does not become significantly faster at high temperature, comparing with the release at a pH of 5.8. This shows that the decreasing pH has more effect on the speed of drug release than increasing temperature. According to the results obtained, we can conclude that the most DOX is conjugated to the –COOH group of PMA blocks, which can release it only in an acidic environment.
Results for MTT assay
The biocompatibility of the synthesized triblock terpolymer and cytotoxic effects of the DOX-loaded triblock terpolymer/Fe3O4 were evaluated using MTT assay against A549 cells, as illustrated in . A549 cells were exposed to variable concentrations of DOX-loaded triblock terpolymer/Fe3O4 for 48 and 72 h and IC50 of 2 and 1.2 obtained for 48 and 72 h, respectively. The results of cytotoxicity assay exhibited that IC50 of DOX-loaded triblock terpolymer/Fe3O4 on A549 cell line was time and dose dependent ().
Figure 9. The cytotoxicity for A549 cells induced by the treatment with DOX-loaded triblock terpolymer/Fe3O4 and free DOX with different concentrations for 48 and 72 h.
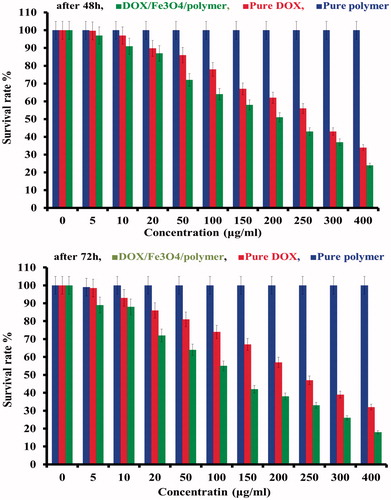
As can be realized in , after 48 and 72 h, the cell viability was over 98% even at high concentration of poly [(PMA-PNIPAM)m-b-PEG-b-(PNIPAM-PMA)m] triblock terpolymer (500 μg mL−1), demonstrating that this polymer is biocompatible, and can be suitable as a biomaterial for nanomedicine applications.
These results represented that DOX-loaded triblock terpolymer/Fe3O4 can be considered as an efficient anticancer drug delivery nanosystem. Because in the equal concentration the DOX-loaded triblock terpolymer was exhibited higher cytotoxic properties in comparison with free DOX. Therefore, the dose of the chemotherapy drug can be decreased; as a result, it is expected to be less the side effects of drug-loaded triblock terpolymer in compared with free drugs.
Conclusion
In this study, we have successfully synthesized a dual responsive and intelligent nanocomposite from PEG, PMA, PNIPAM and Fe3O4 nanoparticles using reversible addition fragmentation chain transfer (RAFT) polymerization. The poly [(PMA-PNIPAM)m-b-PEG-b-(PNIPAM-PMA)m] and Fe3O4 nanoparticles were characterized by FT-IR, VSM, GPC, SEM and TEM. GPC analysis proved the successful synthesis of triblock terpolymers with well-controlled molecular weight. The polydispersity and molecular weight obtained 1.16 and 13,800 g/mol, respectively. The synthesized triblock terpolymer presented the micellization properties with pH- and thermo-responsive behaviors. UV–vis spectroscopy, DLS and TEM were confirmed that the synthesized triblock terpolymers were self-assembled into core–shell micelles by changes in pH and temperature. The thermo-sensitivity of triblock terpolymer reduced at physiological pH and temperature, because of the existence of carboxylic groups in MA segment and hydrophilic PEG. The average sizes of polymeric micelles and nanocomposites were obtained from 25 to 70 nm in different conditions of temperature and pH.
The poly [(PMA-PNIPAM)m-b-PEG-b-(PNIPAM-PMA)m] triblock terpolymer micelles were able to encapsulate DOX (64.8%). The drug release studies showed that the drug release rate increasing with the decrease of pH from 7.4 to 5.8 in both conditions at 37 and 42 °C. Higher release rate of the drug ascribed to protonation of PMA section and reduction of the electrostatic interactions between DOX and triblock terpolymer in mildly acidic conditions [Citation64]. The biocompatibility and suitability of the triblock terpolymer as drug carriers was confirmed by MTT assay on A549 cell line. MTT results showed the strong anti-growth effects and high anticancer performance of DOX-loaded triblock terpolymer/Fe3O4 on A549 cell line in comparison with pure DOX. Based on the results of this study, the synthesized poly [(PMA-PNIPAM)m-b-PEG-b-(PNIPAM-PMA)m]/Fe3O4 nanocomposite can be perfect carriers for a powerful chemotherapeutic agent for lung cancer patients. These nanoparticles can be considered a suitable candidate for drug development by reason of its unique physicochemical and biocompatibility characteristics.
Abbreviations | ||
DOX | = | doxorubicin |
PMA | = | poly (methacrylic acid) |
PNIPAM | = | poly (N-isopropyl acrylamide) |
CP | = | Cloud point |
LCST | = | lower critical solution temperature |
PEG | = | poly (ethylene glycol) |
RAFT | = | reversible addition–fragmentation chain transfer |
THF | = | tetrahydrofuran |
DMF | = | dimethylformamide |
DCM | = | dichloromethane |
FBS | = | foetal bovine serum |
TEM | = | transmission electron microscopy |
SEM | = | scanning electron microscopy |
GPC | = | gel permeation chromatography |
AIBN | = | azobisisobutyronitrile |
FT-IR | = | Fourier transform infrared spectroscopy |
UV–vis | = | ultraviolet–visible |
PDI | = | polydispersity index |
DLS | = | dynamic light scattering |
CTA | = | chain transfer agent |
DMAP | = | 4-dimethylaminopyridine |
PBS | = | phosphate-buffered saline |
A549 | = | lung cancer cell |
MTT | = | 3-(4,5-dimethylthiazol-2-Yl)-2,5-diphenyltetrazolium bromide |
Acknowledgements
The authors are grateful for support from the Tabriz Payame Noor University and National Institute for Medical Research Development (NIMAD), Grant no. 958659.
Disclosure statement
No potential conflict of interest was reported by the authors.
Reference
- Kim JH, Chae M, Kim WK, et al. Salinomycin sensitizes cancer cells to the effects of doxorubicin and etoposide treatment by increasing DNA damage and reducing p21 protein. Br J Pharmacol. 2011;162:773–784.
- Wu C-C, Li T-K, Farh L, et al. Structural basis of type II topoisomerase inhibition by the anticancer drug etoposide. Science. 2011;333:459–462.
- Hasinoff BB, Herman EH. Dexrazoxane: how it works in cardiac and tumor cells. Is it a prodrug or is it a drug? Cardiovasc Toxicol. 2007;7:140–144.
- Deng S, Yan T, Jendrny C, et al. Dexrazoxane may prevent doxorubicin-induced DNA damage via depleting both topoisomerase II isoforms. BMC Cancer. 2014;14:842.
- Sugiyama T, Sadzuka Y. Theanine and glutamate transporter inhibitors enhance the antitumor efficacy of chemotherapeutic agents. Biochim Biophys Acta. 2003;1653:47–59.
- Sun C, Lee JS, Zhang M. Magnetic nanoparticles in MR imaging and drug delivery. Adv Drug Deliv Rev. 2008;60:1252–1265.
- Egusquiaguirre SP, Igartua M, Hernández RM, et al. Nanoparticle delivery systems for cancer therapy: advances in clinical and preclinical research. Clin Transl Oncol. 2012;14:83–93.
- Zhang G, Zeng X, Li P. Nanomaterials in cancer-therapy drug delivery system. J Biomed Nanotechnol. 2013;9:741–750.
- Nicolas J, Mura S, Brambilla D, et al. Design, functionalization strategies and biomedical applications of targeted biodegradable/biocompatible polymer-based nanocarriers for drug delivery. Chem Soc Rev. 2013;42:1147–1235.
- Chauhan VP, Jain RK. Strategies for advancing cancer nanomedicine. Nat Mater. 2013;12:958–962.
- Ye F, Barrefelt Å, Asem H, et al. Biodegradable polymeric vesicles containing magnetic nanoparticles, quantum dots and anticancer drugs for drug delivery and imaging. Biomaterials. 2014;35:3885–3894.
- Khan A, Rashid R, Murtaza G, et al. Gold nanoparticles: synthesis and applications in drug delivery. Trop J Pharm Res. 2014;13:1169–1177.
- Allen TM, Cullis PR. Liposomal drug delivery systems: from concept to clinical applications. Adv Drug Deliv Rev. 2013;65:36–48.
- Rasmussen JW, Martinez E, Louka P, et al. Zinc oxide nanoparticles for selective destruction of tumor cells and potential for drug delivery applications. Expert Opin Drug Deliv. 2010;7:1063–1077.
- Mou X, Ali Z, Li S, et al. Applications of magnetic nanoparticles in targeted drug delivery system. J Nanosci Nanotechnol. 2015;15:54–62.
- Mody VV, Cox A, Shah S, et al. Magnetic nanoparticle drug delivery systems for targeting tumor. Appl Nanosci. 2014;4:385–392.
- Saadatjou N, Fattahi H. Core–shell pH-responsive poly (vinylpyrrolidone)-block-poly (ethylene glycol)-block-poly (methacrylic acid) coated magnetic nanoparticle as a carrier for delivery of poorly water-soluble lovastatin. Pharm Chem J. 2017;51:1–10.
- Alimohammadi YH, Joo SW. PLGA-based nanoparticles as cancer drug delivery systems. Asian Pac J Cancer Prev. 2014;15:517–535.
- Venkatasubbu GD, Ramasamy S, Avadhani G, et al. Surface modification and paclitaxel drug delivery of folic acid modified polyethylene glycol functionalized hydroxyapatite nanoparticles. Powder Technol. 2013;235:437–442.
- Rösler A, Vandermeulen GW, Klok H-A. Advanced drug delivery devices via self-assembly of amphiphilic block copolymers. Adv Drug Deliv Rev. 2012;64:270–279.
- Santhosh PB, Ulrih NP. Multifunctional superparamagnetic iron oxide nanoparticles: promising tools in cancer theranostics. Cancer Lett. 2013;336:8–17.
- Su Y, Hu Y, Du Y, et al. Redox-responsive polymer–drug conjugates based on doxorubicin and chitosan oligosaccharide-g-stearic acid for cancer therapy. Mol Pharm. 2015;12:1193–1202.
- Zhang C, Pan D, Luo K, et al. Dendrimer–doxorubicin conjugate as enzyme-sensitive and polymeric nanoscale drug delivery vehicle for ovarian cancer therapy. Polym Chem. 2014;5:5227–5235.
- Fu L, Sun C, Yan L. Galactose targeted pH-responsive copolymer conjugated with near infrared fluorescence probe for imaging of intelligent drug delivery. ACS Appl Mater Interfaces. 2015;7:2104–2115.
- Liu H, Lin S, Feng Y, et al. CO2-responsive polymer materials. Polym Chem. 2017;8:12–23.
- Lin S, Schattling P, Theato P. Thermo-and CO2-responsive linear polymers and hydrogels as CO2 capturing materials. Sci Adv Mater. 2015;7:948–955.
- Mura S, Nicolas J, Couvreur P. Stimuli-responsive nanocarriers for drug delivery. Nat Mater. 2013;12:991–1003.
- Zhang CY, Yang YQ, Huang TX, et al. Self-assembled pH-responsive MPEG-b-(PLA-co-PAE) block copolymer micelles for anticancer drug delivery. Biomaterials. 2012;33:6273–6283.
- Cheng R, Meng F, Deng C, et al. Dual and multi-stimuli responsive polymeric nanoparticles for programmed site-specific drug delivery. Biomaterials. 2013;34:3647–3657.
- Gerweck LE, Seetharaman K. Cellular pH gradient in tumor versus normal tissue: potential exploitation for the treatment of cancer. Cancer Res. 1996;56:1194–1198.
- Kozlovskaya V, Kharlampieva E, Mansfield ML, et al. Poly (methacrylic acid) hydrogel films and capsules: response to pH and ionic strength, and encapsulation of macromolecules. Chem Mater. 2006;18:328–336.
- Xue W, Champ S, Huglin MB. Thermoreversible swelling behaviour of hydrogels based on N-isopropylacrylamide with a zwitterionic comonomer. Eur Polym J. 2001;37:869–875.
- Shibayama M, Mizutani S-y, Nomura S. Thermal properties of copolymer gels containing N-isopropylacrylamide. Macromolecules. 1996;29:2019–2024.
- Li Z, Guan J. Thermosensitive hydrogels for drug delivery. Expert Opin Drug Deliv. 2011;8:991–1007.
- Veronese FM, Pasut G. PEGylation, successful approach to drug delivery. Drug Discov Today. 2005;10:1451–1458.
- Wu H, Zhu L, Torchilin VP. pH-sensitive poly (histidine)-PEG/DSPE-PEG co-polymer micelles for cytosolic drug delivery. Biomaterials. 2013;34:1213–1222.
- Glaied O, Delaite C, Riess G. Synthesis of PCL-b-PVAc block copolymers by combination of click chemistry, ROP, and RAFT polymerizations. Polym Bull. 2012;68:607–621.
- Zhang H. Controlled/”living” radical precipitation polymerization: a versatile polymerization technique for advanced functional polymers. Eur Polym J. 2013;49:579–600.
- Moad G, Chiefari J, Krstina J, et al. Living free radical polymerization with reversible addition–fragmentation chain transfer (the life of RAFT). Polym Int. 2000;49:993–1001.
- Lowe AB, McCormick CL. Reversible addition–fragmentation chain transfer (RAFT) radical polymerization and the synthesis of water-soluble (co) polymers under homogeneous conditions in organic and aqueous media. Prog Polym Sci. 2007;32:283–351.
- Reddy KR, Park W, Sin BC, et al. Synthesis of electrically conductive and superparamagnetic monodispersed iron oxide-conjugated polymer composite nanoparticles by in situ chemical oxidative polymerization. J Colloid Interface Sci. 2009;335:34–39.
- Rajan M, Murugan M, Ponnamma D, et al. Poly-carboxylic acids functionalized chitosan nanocarriers for controlled and targeted anti-cancer drug delivery. Biomed Pharmacother. 2016;83:201–211.
- Zou Y, Liu P, Liu C-H, et al. Doxorubicin-loaded mesoporous magnetic nanoparticles to induce apoptosis in breast cancer cells. Biomed Pharmacother. 2015;69:355–360.
- Mohammad P, Nosratollah Z, Mohammad R, et al. The inhibitory effect of Curcuma longa extract on telomerase activity in A549 lung cancer cell line. Afr J Biotechnol. 2010;9:912.
- Guo M, Yan Y, Zhang H, et al. Magnetic and pH-responsive nanocarriers with multilayer core–shell architecture for anticancer drug delivery. J Mater Chem. 2008;18:5104–5112.
- Yin X, Hoffman AS, Stayton PS. Poly(N-isopropylacrylamide-co-propylacrylic acid) copolymers that respond sharply to temperature and pH. Biomacromolecules. 2006;7:1381–1385.
- Chao G, Deng H, Huang Q, et al. Preparation and characterization of pH sensitive semi-interpenetrating network hydrogel based on methacrylic acid, bovine serum albumin (BSA), and PEG. J Polym Res. 2006;13:349–355.
- Roman MJ, Decker EA, Goddard JM. Fourier transform infrared studies on the dissociation behavior of metal-chelating polyelectrolyte brushes. ACS Appl Mater Interfaces. 2014;6:5383–5387.
- Khalilov RI, Ahmadov IS, Kadirov SG. Two types of kinetics of membrane potential of water plant leaves illuminated by ultraviolet light. Bioelectrochemistry. 2002;58:189–191.
- Khalilov RI, Khomutov GB, Tikhonov AN. Effect of ultraviolet radiation on structural-functional characteristics of the thylakoid membrane. Russ Plant Physiol. 1993;3:338–342.
- Youssef NA, Gurbanov EM, Haciyeva SR, et al. Antioxidant enzymes, fluctuating asymmetry and morphological changes of urban trees as an ecological indicator of heavy metal stress. Int J Pharm Sci Health Care. 2013;1:1–18.
- Khalilov RI, Nasibova AN, Serezhenkov VA, et al. Accumulation of magnetic nanoparticles in plants grown on soils of Apsheron peninsula. Biophysics. 2011;56:316–322.
- Khalilov RI, Akhmetov IS, Goldfeld M. Binary response of the membrane-potential in the leaf-cells of Vallisneria Spiralis on pulsed UV excitation. Doklady Akademii Nauk. 1992;4:850–852.
- Asghari F, Samiei M, Adibkia K, et al. Biodegradable and biocompatible polymers for tissue engineering application: a review. Artif Cells Nanomed Biotechnol. 2017;45:185–192.
- Eskandari L, Akbarzadeh A, Zarghami N, et al. Gold nanoprobe-based method for sensing activated leukocyte cell adhesion molecule (ALCAM) gene expression, as a breast cancerBiomarker. Artif Cells Nanomed Biotechnol. 2017;45:277–282.
- Mohammadian F, Abhari A, Nejati-Koshki K, et al. New state of nanofibers in regenerative medicine. Artif Cells Nanomed Biotechnol. 2017;45:204–210.
- Saeid Shabestari K, Masoud F, Abolfazl A, et al. Magnetic nanoparticles: preparation methods, applications in cancer diagnosis and cancer therapy. Artif Cells Nanomed Biotechnol. 2017;45:6–17.
- Sonia Fathi K, Majid M, Yunes P, et al. Magnetic nanoparticles in cancer diagnosis and treatment: a review. Artif Cells Nanomed Biotechnol. 2017;45:1–5.
- Mehdizadeh A, Somi MH, Darabi M, et al. Liposome-mediated RNA interference delivery against Erk1 and Erk2 does not equally promote chemosensitivity in human hepatocellular carcinoma cell line HepG2. Artif Cells Nanomed Biotechnol. 2017. DOI:10.1080/21691401.2016.1269117
- Harrigan P, Wong K, Redelmeier T, et al. Accumulation of doxorubicin and other lipophilic amines into large unilamellar vesicles in response to transmembrane pH gradients. Biochim Biophys Acta (BBA)-Biomembranes. 1993;1149:329–338.
- Schu¨wer N, Klok H-A. Tuning the pH sensitivity of poly(methacrylic acid) brushes. Langmuir. 2011;27:4789–4796.
- Tian Y, Bromberg L, Lin S, et al. Complexation and release of doxorubicin from its complexes with pluronic P85-b-poly(acrylic acid) block copolymers. J Control Release. 2007;121:137–145.
- Bialik-Wąs K, Pielichowski K. Poly (acrylic acid-co-methyl methacrylate)/metronidazole systems: synthesis and complexation. Acta Biochim Pol. 2013;60:835–838.
- Kanamala M, Wilson WR, Yang M, et al. Mechanisms and biomaterials in pH-responsive tumour targeted drug delivery: a review. Biomaterials. 2016;85:152–167.