Abstract
We synthesized silver nanoparticles using Rumex hymenosepalus root extract (Rh). Nanoparticles were subjected to a purification process and final product is a composite of Rh and silver nanoparticles (AgNPsC). Transmission electron microscopy (TEM), high-resolution transmission electron microscopy (HRTEM), X-ray diffraction (XRD), and X-ray photoelectron spectroscopy (XPS) were used to perform a microstructure study. Additionally, two fractions (RhA and RhB) were obtained from the original extract by filtration with tetrahydrofuran (THF); both fractions were analyzed using UV-Vis spectroscopy, Fourier transform infrared spectroscopy (FT-IR), and 2,2-diphenyl-1-picrylhydrazyl (DPPH); total polyphenol content was also determined. Separate inhibition tests for AgNPsC and RhA and RhB were applied to Gram-positive bacteria, Gram-negative bacteria, and yeast (Candida albicans) using the well diffusion method. Extract fractions were found to have inhibitory effects only over Gram-positive bacteria, and silver nanoparticles showed inhibitory effects over all the evaluated microorganisms. Cytotoxicity was evaluated using the tetrazolium dye (MTT) assay in mononuclear peripheral blood cells. In addition, we assessment AgNPsC in THP-1 monocyte cell line, using the cell viability estimation by trypan blue dye exclusion test (TB) and Live/Dead (LD) cell viability assays by confocal microscopy.
Graphical Abstract
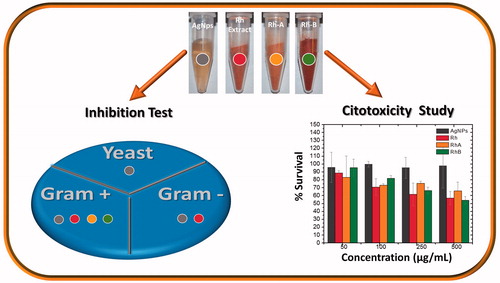
Introduction
Over the last years, infectious diseases have been associated with 25% of deaths worldwide. Although there is significant progress in the research and treatment of many communicable diseases by viruses, parasites, bacteria, and yeast, the number and distribution of pathogenic microorganisms resistant to antimicrobial drugs [Citation1–3] have also increased dramatically. In the case of Mexico, the levels of resistance to three of the most common antibiotics (penicillin, ampicillin and co-trimoxazole) are among some of the highest in Latin America, reaching values close to 70% for Staphylococcus pneumoniae (S. pneumoniae), Salmonella spp., and Shigella spp., respectively. For the specific cases of the most common pathogenic bacteria in Mexican hospitals, i.e. Klebsiella spp., Staphylococcus aureus (S. aureus), and Pseudomonas aeruginosa (P. aeruginosa), resistance to a wide range of antibiotics is between 28 and 50% [Citation4]. Given this global problem, several new alternatives have been proposed to aid the fight against pathogenic microorganisms, such as the use of natural agents extracted from plants that have remarkable antimicrobial activity, for instance the use of naturally occurring proteins or peptides with antimicrobial activity such as defensin, lysozyme, and lactoferrin, and the use of bacterial viruses or phages as bactericidal agents [Citation5–7]. Another approach propounds treatments based on the use of metallic nanoparticles as antimicrobial agents; the case of silver should be highlighted because of its great effectiveness as compared to other materials (0.5 nm) [Citation8–10]. In this regard, recent nanomaterial synthesis research is based on the use of biocompatible compounds to generate nanoscale products, a process termed green synthesis.
On the one hand, studies on the manufacturing of metallic nanoparticles using compounds extracted from plants are abundant, and they generally focus on the production of gold (Au), silver (Ag), palladium (Pd), or platinum (Pt) nanoparticles; all of these studies have reported antibacterial response [Citation11–19]. On the other hand, the most common antimicrobial compounds in extracts from plants are phenolics, terpenoids, essential oils, alkaloids, lectins, polypeptides, and polyacetylenes [Citation11,Citation20–22]. Solvents used for extractions include carbon dioxide, carbon dioxide/isopropyl alcohol, ethanol (70% v/v), and water [Citation23–25], while the methods employed in the evaluation of antimicrobial activities are disc diffusion, well diffusion, and broth dilution [Citation26–29]. Antimicrobial activity is difficult to determine using the same method for both agents, antibiotics and nanoparticles. The disc method is frequently used to test antibiotic action, whereas plant extracts and nanoparticles produce better outcomes when using the well diffusion method. Synthesized silver nanoparticles, stabilized with polyphenols, prevent these nanomaterials from rushing and conglomerating. Nanoparticle size and shape are important physical properties that will determine variations; specifically, angle-shaped nanomaterials have shown their effectiveness when used as nanomedicine [Citation30–32]. Another important property is pH, given that many microorganisms are highly sensitive to pH changes. The interaction mechanism between metallic nanoparticles and bacterial membranes appears to be transported through the pores; surface interaction between nanoparticles and membrane modifies or impairs bacterial cell operation. Another possible mechanism is related to nanoparticle electrical charges; silver nanoparticle-positive charges have shown more antibacterial activity due to the negative charge of the bacterial membrane [Citation33–35]. Some authors have observed an increased inhibitory effect produced by a mixture of antibiotics and nanoparticles, which act synergically together [Citation36,Citation37]. Finally, it is well known that plants have been used in traditional medicine as an alternative to treatments for infectious diseases provoked by Gram-negative and Gram-positive bacteria, as well as by yeast. Assessing the damage on healthy cell caused by nanoparticles is important, mainly on cells whose function plays a role in the immune response. Performing toxicological tests on peripheral blood mononuclear cells are essential to make sure nanoparticles inhibit microorganism development without damage to host cells. For this reason, it is important to minimize cellular toxicity and increase bactericidal effect. Considering all properties of the natural extracts that favour health, in this paper, we propose to study AgNPsC and test its toxicity in human peripheral blood mononuclear cells and THP-1 cell line, in addition to its microbicidal and fungal effects.
Experimental
Materials
Silver nitrate (AgNO3) (Sigma-Aldrich, purity ≥99.5%) was used as promotor of Ag+ ions and Rumex hymenosepalus root extract (Rh) as reducing agent in the synthesis of silver nanoparticles. Non-denatured ethyl alcohol (Sigma-Aldrich, purity ≥99.5%) and ultrapure Millipore water were employed as solvents in the extraction process, which used an 18.2 MΩ resistor. Also, Millipore water was used as AgNPsC dispersant and to extract fractions for antibiogram tests.
Synthesis of AgNPsC
Rumex hymenosepalus is an endemic plant from the southern United States and northern México. Traditionally, this tuber has been used in infusions as a remedy for throat infections. Remarkable among the root’s compounds are stilbenes and catechins [Citation38] characterized by the abundance of OH groups in their molecular structures, which are susceptible to deprotonate easily with a subsequent transfer of electrons in redox reactions. This property gives these molecules a strong antioxidant activity and extracts from Rh roots are suitable candidates as reducing agents in metallic nanoparticle synthesis. For all AgNO3 concentrations, the visual appearance of the samples changed shortly after adding the plant extract, which indicated that a reduction reaction was taking place [Citation39]. Plant extracts were prepared as follows: 50 g of Rumex hymenosepalus roots was dried and cut into 5 mm thick slices. All the contents were mixed to homogenize the sample, from which 15 g was randomly removed. This mass was placed in a glass culture flask with 100 ml of ethanol/water 70:30 (v/v) mix for extraction by maceration and covered with foil and left at room temperature for 3 weeks in a dark place to ensure that the extraction was completed. The recovered solution was filtered using a Whatman 40 filter (8 μm pore size) and then filtered using a 0.20-μm Acrodisc filter. The solution obtained from the final filtration was divided into two portions: 10 ml was used as a reducing agent to synthesize AgNPsC and 60 ml was used to obtain the extract fractions. For the synthesis of AgNPsC, 64 ml of ultrapure water, 4 ml of Rh, and 4 ml of AgNO3 (0.1 M) were mixed. The mixture was stirred gently (200 rpm) with the magnet for 24 h in the absence of light at 25 °C. Subsequently, a purification process was followed to recover the obtained nanomaterials.
Characterization of nanoparticles
The extract was characterized by UV-Vis spectroscopy and Fourier transform infrared spectroscopy (FT-IR). The AgNPsC were characterized with transmission electron microscopy (TEM). Particle size and abundance depended on AgNO3 concentration; for the antimicrobial assays, the average nanoparticle diameter was on the order of 7 nm. The AgNPsC purification method consisted of three 30-min centrifugation cycles at 5000 rpm and a temperature of 25 °C. The supernatant was decanted, and ultrapure water was added and sonicated for 5 min, and then the cycle was restarted. After the third cycle, we added 10 ml ethanol, sonicated for 5 min, and centrifuged at 5000 rpm for 30 min more, and the supernatant was retained. The precipitate was placed in a desiccator connected to a vacuum 7 pump for 24 h and the recovered dried powder was weighted in an analytical balance and dispersed in a solvent appropriate for characterization. This treatment was used to obtain the powder samples for characterization with X-ray diffraction (XRD) and X-ray photoelectron spectroscopy (XPS).
Separation of fractions
The extract solution was dried out to separate the solid part using a rotavapor and then placed in the desiccator connected to a vacuum pump to obtain a powdered extract. Afterwards, it was fractionated using tetrahydrofuran (THF). For this purpose, the powder was placed on a glass filter and 100 ml of THF was added drop by drop; the process was carried out three times to ensure a complete extraction of the soluble part (fraction B). The soluble part was extracted in the same way and the wet powder of the insoluble part (fraction A) was placed in the desiccator for 24 h.
Free radical scavenging activity and total polyphenol content
The 2,2′-diphenyl-1-picrylhydrazyl (DPPH) tests can determine free radical scavenging activity in percentage terms. The Rh extract and fractions Rh-A and Rh-B in ethanol/water were tested using DPPH with ethanol as solvent. The DPPH concentration was 300 μmol/L, of which 100 μL of DPPH was mixed with 100 μL of Rh at a concentration of 97.5 μg/ml, and the same was done for RhA and RhB. All processes were left in the dark for 30 min and the absorbance was measured at 520 nm. The samples were evaluated for 25, 12.5, 6.75, and 3.75 μg/mL. The controls used were vitamin C and catechins at a concentration of 70 μmol/L.
Analysis of total phenolic content
Folin–Ciocalteu reagent (0.25 N) was used to determine total polyphenol content in Rh, RhA, and RhB samples. Samples were dissolved in distilled water (80 μl). We used 40 µL of Folin–Ciocalteu and added 60 µL of sodium carbonate solution at 5%. The final volume of the system was obtained by adding distilled water to a volume of 280 µL. A Multiskan Spectrum (Thermo Electron) spectrophotometer was used to measure a wavelength of 750 nm after maintaining the sample in the dark for at least 1 h. The total concentration of phenolic contents was expressed as gallic acid equivalents (milligrams of gallic acid per gram of dried extract) for Rh, RhA, and RhB.
Antibacterial activity
In order to study the antimicrobial activity of Rh extract and AgNPsC solutions, we employed the well diffusion method. This method started with the mass culturing of a known inoculum and subsequently a diameter was drilled over the agar plates to add a fixed quantity of the antimicrobial agent for assessment. Finally, using a vernier scale (resolution of 0.01 mm), we measured the inhibition halo diameter around microorganisms. Toxicity was tested in cultures of Gram-positive bacteria ATCC (S. aureus ATCC 25923, Listeria monocytogenes (L. monocytogenes) ATCC 7644), Gram-negative bacteria Escherichia coli (E. coli) ATCC 11229, E. coli O157:H7 ATCC 43895, Salmonella serovar typhi (S. serovar typhi) ATCC 6539, Pseudomonas aeruginosa (P. aeruginosa) ATCC 15442), and yeast Candida albicans (C. albicans) ATCC 90028.
Cytotoxicity MTT assay
The cytotoxic activity of the AgNPsC was evaluated in peripheral blood mononuclear cells using the 3-[4,5-dimethylthiazol-2-y1]-2,5-dipheniltetrazolium bromide (MTT) assay, in which MTT is reduced by the action of mitochondrial enzymes. Mononuclear cells from human peripheral blood (our study follow the principles of the Declaration of Helsinki) were purified by centrifugation at 500 g of Lymphoprep™ for 20 min. The cells were recovered and three times washed with PBS (135 mM of NaCl, 2.5 mM of KCl, 10.2 mM of Na2HPO4, and 1.7 mM of KH2PO4 in Milli-Q water at pH 7.4). The pellet was resuspended in RPMI-1640 medium (Sigma-Aldrich, San Luis, MO) supplemented with 10% foetal bovine serum (GibcoBRL, Grand Island, NY), l-glutamine (Sigma-Aldrich) at 2 mM, penicillin at 100 μ/mL, streptomycin (Sigma-Aldrich) at 100 μg/ml, and sodium pyruvate (Sigma-Aldrich) at 2 mM. Cells were counted in a Neubauer chamber and their viability was determined by trypan blue exclusion (Sigma-Aldrich). They were adjusted to 100,000 cells/mL, and 100 μL per well was placed in 96-well plates. 10 μL of AgNPsC, Rh, RhA, and RhB was used to obtain the final concentrations of 500, 250, 100, and 50 μg/mL. Concentrations were incubated for 24 h at 37 °C, 5% of CO2, and 90% relative humidity. After 24 h, we added 10 µL per well of an MTT solution at 5 mg/mL (Sigma-Aldrich) and incubated again for 4 h. 100 ml of an isopropanol mixture (Sigma-Aldrich) and 0.4 mM of 1% HCl/SDS were added to each well to dissolve the formed crystals. The plate was stirred and the complete dissolution of the crystals was microscopically verified. The absorbance was measured at 570 nm on a multimode plate reader (Synergy HTX, BioTek, Winooski, VT) using the Gen5 software. Dose–response curves were generated using the Origin software.
Cytotoxicity trypan blue dye exclusion test
THP-1 cells (ATCC TIB-202) were cultured in modified RPMI-1640 complete medium with 2.05 mM l-glutamine, supplemented with 10% heat-inactivated FBS at 37 °C in a 5% CO2 atmosphere. Cell counts and viability estimation by trypan blue dye exclusion test (TB) were performed before and after every assay. THP-1 cells were maintained in a logarithmic growth phase at a concentration of 2 × 105 cells/mL. The change of RPMI-1640 on cell culture was changed every 2 or 4 days.
THP-1 cells in the exponential growth phase were used for cytotoxicity. THP-1 cells were seeded in 0.5-ml tubes (Eppendorf) at a density of 1 × 105 cells/tube containing RPMI-1640 medium. Once the cell concentration was adjusted, treatment with the AgNPsC at the concentrations of 100, 250, and 500 µg/mL was carried out. AgNPsC were produced originally in ultrapure water, and then to reach the final concentration, a part of the nanomaterial was resuspended in complete RPMI-1640. The treatment was carried out in a humidified incubator at 37 °C and 5% CO2 for 24 h in constant agitation on a test tube rocker (United Products & Instruments, Inc., Dayton, NJ) at 25 cycles per minute in order to avoid the agglomeration and precipitation of the silver material and ensure a homogeneous distribution of nanomaterials in the culture medium. The assay was performed in triplicate, where the controls consisted of untreated cells and cells treated with the metal precursor.
Cytotoxicity Live/Dead assay by confocal microscopy
After 24 h of incubation with AgNPsC, we proceeded to make the cell count using trypan blue exclusion assay (Sigma) to establish the percentage of cellular viability, and fluorescence staining using Live/Dead viability/cytotoxicity kit (Thermo Fisher, USA) for later analysis by confocal microscopy (LSM800, Carl Zeiss, Göttingen, Germany). For both dyes, the manufacturer’s recommendation was used. This assay was realized in PBMC and THP-1 cell lines for AgNPsC and in PBMC for Rh, RhA, and RhB.
Result and discussion
Characterization of nanoparticles
Silver nanoparticle synthesis consists on an oxidation–reduction process, where Rh polyphenolic compounds work as reducing agents by transferring electrons to the metal ions. shows the chemical structure of the (−)-epigallocatechin gallate (EGCG), one of the most abundant compounds in Rh. EGCG has two rings which have the same local structure, B-ring with pyrogallol-type structure and D-ring with gallate group. Both rings have three hydroxyl groups that may potentially participate in the synthesis [Citation40], so the synthesis reaction is described considering a single ring. The involved process is a deprotonation mechanism of two hydroxyl groups in the ring (), followed by an electron transfer which give rise to two unpaired electrons (). Protons react with two nitrate ions forming nitric acid, which is consistent with the acidification of the synthesis reaction (Figure S3) and two silver ions are reduced to metallic silver by electronic transfer, while the aromatic ring is reconfigured to the stable quinone form (). In the case of t-resveratrol, another abundant polyphenol in Rh, hydroxyl of the phenolic ring is involved in oxidation–reduction reaction by a mechanism analogous to that described above for EGCG [Citation41] obtaining a metallic silver atom for each molecule of oxidized t-resveratrol. AgNPsC are stabilized through the carbonyl groups of the quinones formed by the oxidation of polyphenols. Polyphenol molecules that are in excess in the synthesis promote the material stability by phenolic hydroxyls. shows an AgNPsC stabilized by EGCG and t-resveratrol oxidized molecules and not oxidized. Regions in green and yellow correspond to hydroxyl and carbonyl groups (respectively) that interact with the nanoparticle [Citation42,Citation43]. Figure S1 describes EGCG and t-resveratrol molecules in their oxidized and normal form. The kinetic of synthesis of AgNPs (absence of light) was monitored by UV-Vis spectroscopy (Figure S2). It was found an increase of intensities until reaching stability at 24 h. For the same synthesis, pH was measured as a function of time (Figure S3). Nanoparticles in ultrapure water have a pH of 7 and a zeta potential of −45 mV (high stability) measured with Zetasizer NS (Malvern, PA), and the zeta potential for Rh dissolved in ultrapure water is around −27 mV (Figure S4). Nanoparticle sizes were measured by DLS of Zetasizer NS (resolution of 0.5 nm). On ultrapure water, the mean size is 10 nm and the mean size in RPMI is 230 nm (Figure S5). As we can see, it is possible to note a significant increase in the size of the nanomaterial that was incubated with complete RPMI-1640 medium. Several articles have studied this phenomenon of growth of nanomaterials when they are exposed to cell culture medium, a phenomenon that is called a corona effect [Citation44,Citation45]. This effect is a direct result of the interaction of the particles with components of the culture medium, such as proteins, which can form a soft or a hard corona, depending on the affinity of the proteins for the nanomaterial. The nanomaterial itself is able to interact with proteins of the medium; however, it is important to remember that this nanomaterial was synthesized with Rh, which is rich in polyphenols, where we found as major components resveratrol and EGCG, which are also known to be capable of interacting with proteins, so that they may be potentiating the corona effect.
UV-Vis spectroscopy
In this study, we used Rumex hymenosepalus extracts as reducing agents to synthesize silver nanoparticles. These extracts are rich in polyphenol, antioxidant molecules that allow the green synthesis of AgNPsC. We were interested in verifying whether nanoparticles could be stabilized by antioxidant molecules in the extract after the nanoparticle cleaning process. To accomplish this, UV-Vis spectra from the original sample, from the supernatant (first centrifugation cycle), and from purified nanoparticles were recorded on a Perkin-Elmer Lambda 20 spectrometer, with resolution 0.5 nm. The ratio between the absorption bands of polyphenols (λ = 278 nm) and nanoparticles (λ = 450 nm) decreased after nanoparticles were cleaned, indicating that part of the extract had been removed, as shown in . However, the remaining extract formed a complex with the nanoparticles, which was impossible to remove after three centrifugation and sonication cycles. We suppose that this action stabilizes the system and prevents nanoparticle aggregation.
FT-IR
A Fourier transform infrared spectroscopy (FT-IR) from the non-fractionated extract was obtained by a Perkin-Elmer FT-IR System Spectrum GX using a CaF2 cell. The spectrum was obtained on transmittance mode at a resolution of 0.3 cm−1, from 4500 cm−1 to 370 cm−1. shows IR spectrum for Rh and AgNPsC. Peak at 3391 cm−1 corresponds to the stretch mode of the phenolic OH group ring present in the molecules of the extract. In IR spectrum of AgNPsC, the peak at 1648 cm−1 is more intense than in Rh. Some authors report peaks of the carbonyl group (C = O) between 1620 and 1690 cm−1 [Citation46,Citation47], so this increase in the signal can be attributed to the presence of quinones generated by oxidation of polyphenolic molecules of Rh in the synthesis reaction. In general, IR of AgNPsC is almost identical to the IR spectrum of Rh, even after cleaning the AgNPsC which confirms that nanoparticles are stabilized by the polyphenolic molecules involved in the reduction of silver ions. shows the shifts at 2974, 1651, and 1384 cm−1, which corresponds to the stretch modes of the C–H, C = C, and C–O binds of the aromatic ring, respectively. The signal at 1049 cm−1 is associated with the C–O stretch mode of the catechin ester group molecules (formula below), and the shift at 799 cm−1 corresponds to the bending out of the OH par plane in the phenolic rings (). Fractions A and B presented almost identical phenol OH band spectra, although wider for C–H at 2990 and 1690 cm−1, and they also presented a C = O stretching elongation. These bands are not very evident in the other spectra; however, they do exist in the carbonyl groups. The signals at 1608, 1520, and 1458 cm−1 are attributed to C = C aromatic rings, and 767 cm−1 corresponds to C–H out of the plane ().
TEM, HRTEM, and EDS
Nanoparticles were observed with TEM, using a Jeol 2010 F apparatus (JEOL Ltd., Akishima-shi, Japan) operating at 200 kV, with point-to-point resolution of 0.19 nm. We deposited 10 µl of the nanoparticle suspension on a formvar/carbon-coated copper TEM grid (300 Mesh). The sample was vacuum-dried for 24 h before observation. TEM micrographs () show that nanoparticle geometry is quasi-spherical, stable and surrounded by a matrix that can be associated with the extract, and this confirms the results of UV-Vis and IR spectroscopy for AgNPsC. shows the EDS obtained using a QUANTAX 200-TEM X-ray spectrometer (Bruker, Rheinstetten, Germany) with XFlash 4010 detector maximum energy resolution at 100 Kcps [eV] for MnKα ≤125) . The AgNPsC produced signals at 2.984 and 22.166 KeV, which correspond to Ag atoms. The absence of oxygen in the EDS spectrum indicates that nanoparticles do not correspond to oxidized silver. The Cu signal corresponds to the TEM grid. In , we show a nanoparticle population histogram, which was determined from 400 particles obtained from the TEM micrographs. The size and abundance of the resulting particles depended on AgNO3 concentration. For the antimicrobial assays, the average nanoparticle diameter was on the order of 7 nm, and 60% of the population was under 15 nm. Agnihotri et al. have shown that the inhibition of E. coli using AgNPsC using the disc diffusion method is only feasible for nanoparticles under 15 nm [Citation48]. corresponds to a high-resolution image of an AgNPsC such as the ones used in our inhibition test. The interplanar distance of 2.34 Å corresponds to (100) in terms of Miller indices ()).
XDR and XPS
The XRD in was generated using a Rigaku D/max2100 micro-diffractometer, using CuKα radiation (λ = 1.5406 Å) and recorded from 10° to 80° in steps of 0.02° at room temperature. Diffraction shifts match diffraction peaks at 2θ = 38.24°, 44.42°, 64.44°, and 77.40°, which correspond to (111), (200), (220), and (311) metallic silver fcc (face-centred cubic) planes, respectively. These data were obtained from JCPDS file No. 04–0783. The XPS experiment was carried out using a Perkin-Elmer (Model PHI 5100; resolution based on the FWHM of the Ag3d5/2 peak =0.80 eV; XR source: dual standard anode (Mg/Al); 15 K v, 300 W, 20 mA), shows high-resolution XPS spectra of AgNPsC. Binding energies were located at 374.08 eV for Ag3d3/2 and at 368.12 eV for Ag3d5/2. The Ag3d region presented well-separated spin-orbit components of 5.98 eV, which correspond to metallic silver Ag0 [Citation49].
DPPH and total polyphenol content
shows the results for fractions RhA and RhB. It can be observed that RhB has a greater capacity for removing free radicals than RhA. This result is consistent with the data in , wherein the total polyphenol content is also higher for RhB.
Table 1. The content of total polyphenols for Rh, RhA, and RhB.
Silver nanoparticle microbicidal and fungicidal activity testing
The microbicidal and fungicidal activities were evaluated for AgNPsC, RhA, and RhB. Both AgNPsC and fractions were dissolved in ultrapure water to get a concentration of 100 μg/ml. These inhibitory agents were applied to E. coli ATCC 11229, E. coli O157:H7 ATCC 43895, S. serovar typhi ATCC 6539, P. aeruginosa ATCC 15442, S. aureus ATCC 25923, and C. albicans ATCC 90028. The tests were carried out using a modification of the disc diffusion method [Citation50]. We used an amount of culture equivalent to 0.5 McFarland standard (about 1.5 × 108 CFU/mL), which was inoculated on the surface of Mueller-Hinton agar plates where 6-mm-diameter wells were drilled. We added for tests 30 μl of AgNPsC suspended in ultrapure water (silver nanoparticles concentration forming the composite with the molecules of Rh is 100 µg/mL and the concentration of silver is 5.76 μg/mL verified by Atomic Absorption Spectroscopy, see Table S1), 30 μl of RhA, and 30 μl of RhB; as a negative control, was used ultrapure water. The plates were incubated at 37 °C for 24 h. To verify the microbicide and fungicide effect, we measured inhibition halos. All experiments were performed in triplicate. and show the microbicide activity of the whole extract by the disk and well methods. shows the S. aureus (Gram-positive bacteria) inhibition test using disc method, as control was used AgNO3 at concentrations of 0.1 and 0.01 M. We tested AgNPsC and Rh, then the same test was performed using the well diffusion method ()). The best results were obtained using the well diffusion method, which favoured the diffusion of materials while that in disc method materials are partially or totally retained in the cellulose matrix. We also used 0.1 and 0.01 M AgNO3 as positive controls. ) shows a comparison between well diffusion and disc methods for E. coli (Gram-negative bacteria). RhA and RhB did not show inhibition in the case of E. coli, whereas the non-fractionated extract did show inhibition. AgNO3 is a well-known antibacterial, so it was used as a positive control in our tests. We verified that the effect of silver ions was larger than the effect of nanoparticles; however, AgNPsC are made functional with the aim of increasing their biocompatibility as they interact with human cells and act as microorganism inhibitors: their effect should be differentiated and specific. Both RhA and RhB extracts showed microbicidal activity against S. aureus, but not against E. coli. The microorganism most affected by AgNPsC was C. albicans, with a mean halo of 23.66 mm (). The least affected bacterium was the generic E. coli ATCC 11229, which showed a mean inhibition halo of 11.33 mm. We observed that Gram-positive bacteria S. aureus and L. monocytogenes were more affected than Gram-negative bacteria, especially by RhA and RhB, which did not show any adverse effect over Gram-negative bacteria. show inhibition halo results.
Figure 8. Antibacterial activity of AgNPsC and Rh on E. coli ATCC25922 disc method (a), and well diffusion method with two different nanoparticle sizes (b) and (c).
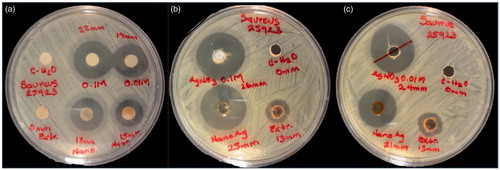
Figure 9. Antibacterial activity of AgNPsC and Rh extract for S. aureus ATCC25923 disc method (a), and well diffusion method with two different sizes of nanoparticles (b) and (c).
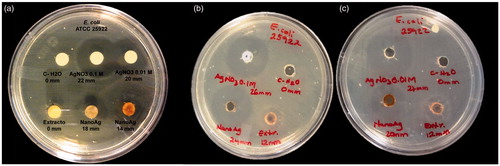
Figure 10. Antibacterial activity of AgNPsC for RhA, and RhB for S. aureus ATCC 25923 (a). Only the inhibition over C. albicans by AgNPsC is shown (b) and E. coli 0157:H7 ATCC 43895 (c).
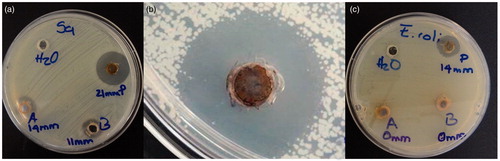
Table 2. Inhibition halo of E. coli ATCC 25922 (Gram-negative) and S. aureus ATCC 25923 (Gram-positive) using AgNPsC and Rh by the well and disc diffusion methods.
Table 3. Inhibition halo of Gram-positive, Gram-negative, and yeast using AgNPsC.
Table 4. Inhibition halo of Gram-positive using fraction RhA and fraction RhB.
In the supplementary information (Figure S6), we tested AgNPsC and both Rh fractions for different volumes. In this case, the inhibitory materials were dispersed in ultrapure water. We did not observe significant changes in the sizes of inhibition halos when volumes varied when they were tested on S. aureus and S. typhi. In preliminary tests, we obtained good results for RhA and RhB dissolved in 10% dimethyl sulphoxide; in these tests, both fractions showed an inhibition halo of 22 mm. This halo is greater than the halo with RhA and RhB dissolved in ultrapure water for S. aureus ATCC 25923 by 8 mm (see Supplementary information, Figure S7).
The inhibitory response of AgNPsC and Rh extract is at least 25% higher than that reported by Ahmed et al. [Citation51] for E. coli and S. aureus, and of the same magnitude as that reported by Nayak et al. [Citation52] for E. coli and Pseudomona in the presence of silver nanoparticles obtained by phytosynthesis. It is interesting to note that, despite high Z potential values, for AgNPsC and Rh (Figure S5), the electrostatic interaction with bacteria is not favourable. The inhibitory effect obtained on the evaluated microorganisms is relevant, so there is probably a mechanism associated with the polyphenolic compounds that complex the silver particle is responsible for the inhibition. The presence of bioactive molecules, which stabilize the AgNPsC, confers certain additional properties associated with the type of complexed molecule. In previous work, we have identified glycated t-resveratrol (piceid) and epigallocatechin gallate as two of the most important antioxidant constituents of Rumex hymenosepalus [Citation38,Citation39]. A possible mechanism of action of our nanocomposite with the evaluated microorganisms is through the interaction of the EGCG that acts destabilizing the Gram-positive peptidoglycan structure and generating pores in the Gram-negative, due to the production of H2O2 [Citation53,Citation54] and by the effect of t-resveratrol, which causes DNA fragmentation, as in the case of E. coli [Citation55]. In addition, the mechanism of ROS generation is associated with the degradation of silver nanoparticles in Ag+ ions, once internalized to the cell [Citation51]. Interestingly, this idea is supported by the results obtained where inhibitory response of the AgNPsC is greater than that of the Rh and their fractions, suggesting a synergistic effect between the polyphenolic molecules of the extract and the nanoparticulate silver.
Cytotoxicity MTT assay
AgNPsC have a low cytotoxic effect on mononuclear cells from human peripheral blood at the concentrations () used in the assay. It was found that AgNPsC cytotoxicity at doses from 50 to 500 µg/mL was about 5%, and there were no statistically significant differences between doses.
When analyzing the Rh fractions, RhB () had a similar dose-dependent cytotoxic effect on the whole extract, contrary to what was shown by RhA (), which had a weak cytotoxic effect without correlation with the dose. and shows that PBMC extract cytotoxicity increased with total Rh and RhB. PBMC extracts were stimulated for 24 h with increasing concentrations of AgNPsC, Rh, RhA, or RhB. When the MTT cytotoxicity test was performed, the Rh-stimulated cells showed significant differences between the 50 µg/mL dose as compared to the 500 µg/mL of dose (p = .0038). Concerning RhA-stimulated cells, there were no differences between doses, but the difference as compared to the unstimulated control for cytotoxicity was near 25%. RhB-stimulated cells showed significant differences from 50 to 250 µg/mL with p = .0096 and from 50 to 500 µg/mL with p = .0001. A two-way ANOVA was performed, followed by Tukey’s multiple comparisons.
Figure 12. LD assay by monocytes of PBMC for Rh (a–c), RhA (e–g), RhB (i–k), and MTT assay for Rh (d), RhA (h), and RhB (l).
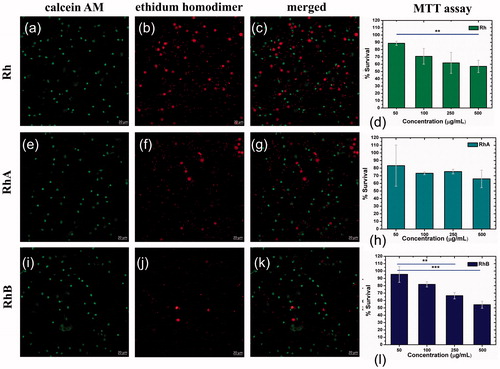
Of particular interest is the cytotoxic response of the different materials to 100 μg/mL since the inhibition tests were performed at this concentration. The MTT assay in PBMC for AgNPsC, Rh, RhA, and RhB has proliferation values of 100%, 70%, 75%, and 80%, respectively.
Cytotoxicity Live/Dead by confocal microscopy, trypan blue by exclusion tests (AgNPsC)
shows LD assay for Rh, the proliferation is about 65%, shows RhA proliferation is 78%, and shows RhB proliferation is 90%, and the confocal microscopy was realized with an objective of 40×. The LD assay in corresponds to 100 µg/mL and viability results are in accordance with MTT assay.
Figure 13. LD assay by monocytes of THP-1 for AgNPsC (a–c), positive control using AgNO3 (d,f), and TB test with AgNPsC over monocytes of THP-1 (g).
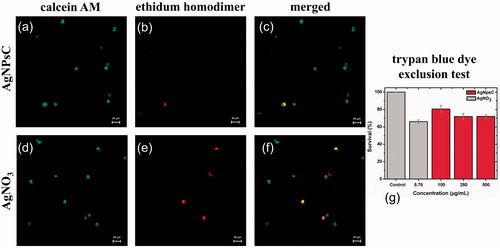
When analyzing the cytotoxic effect of the particles in human peripheral blood mononuclear cells, they were found to be non-toxic to cells. However, it is necessary to study the effect of the nanomaterial on independent cell populations. This is why we opted to work with the monocytic cell line THP-1. 100 µg/mL of AgNPsC (5.76 µg/mL of silver) shows a viability of 80% ()) and the AgNO3 control shows a viability of 66% at same silver concentration ()). The results obtained are similar to those found in the TB test (). Greulich et al. have previously shown that silver nanoparticles are less cytotoxic at the same concentrations as ionized silver [Citation56].
It has been reported that when the cell line RAW. 264.7 is treated with silver nanoparticles modified with tannic acid, cell proliferation decreases by 50% [Citation57], which could be an agglomeration effect. However, Lankoff et al. reported that the aggregation of the material has no effect on the cell viability of the THP-1 line; in addition to that there is no significant difference in cell viability when the nanomaterial concentration is increased [Citation58]. This result is similar to what we found in our work, since the viability becomes insensitive to the increase in the concentration of the material, which may be due to a corona effect caused by both the particle and polyphenol compound. Several works have reported this process and it has been found that when the particle size increases, the entrance pathway and the amount of material that is internalized are affected, which could be reflected in a greater cellular viability, since the material agglomerated in the culture medium could not be internalized [Citation59–61].
Conclusions
The synthesis of silver nanoparticles obtained with the polyphenol molecules of Rh produces AgNPsC of size below 10 nm and highly stable (zeta potential of −45 mV in water). Significant inhibitory effects on Gram-positive, Gram-negative, and yeast were obtained by AgNPsC at 100 µg/mL (5.76 µg/mL Ag concentration) with a more effective response over C. albicans and Gram-positive. Rh, RhA, and RhB produce an inhibitory effect of way specific and differentiated, for S. aureus (Rh, RhA, and RhB) and E. coli (Rh) and for C. albicans do not produce inhibition. It is suggested that EGCG and piced present in Rumex hymenosepalus are responsible for the inhibitory activity of the extract and may be acting in synergy with silver on inhibitory response of AgNPsC. Cytotoxicity assays in PBMC and THP-1 cell line for AgNPsC indicate that nanoparticles do not present a marked cytotoxicity for concentration range of study, presumably because molecules that stabilizing the nanoparticles enhance their biocompatibility.
Supplementary_material.docx
Download MS Word (2.1 MB)Acknowledgements
The authors wish to thank Dr. Lorena Armenta, from the University of Sonora, for supporting our XRD experiments and for Ms. Kareen Krizzan Encinas Soto of Departamento de Ingenierıa Quımica y Metalurgia, Universidad de Sonora, for access and the use of AAS. The authors also thank the laboratory of transmission electron microscopy from the Physics Department of the University of Sonora for providing TEM micrographs and laboratory of biomaterials from the Physics Department of the University of Sonora for providing the confocal microscopy. This research study was partially funded by Consejo Nacional de Ciencia y Tecnología (Conacyt–Mexico) through projects PROINNOVA 221252 and 221258, project PRODEP and project DSA/103.5/14/10945.
Disclosure statement
The authors report no conflicts of interest in this work.
Additional information
Funding
References
- Laxminarayan R, Duse A, Wattal C, et al. Antibiotic resistance—the need for global solutions. Lancet Infect Dis. 2013;13:1057–1098.
- Fears R, ter Meulen V. What do we need to do to tackle antimicrobial resistance? Lancet Glob Health. 2014;2:e11–e12.
- Zowawi HM, Harris PNA, Roberts MJ, et al. The emerging threat of multidrug-resistant Gram-negative bacteria in urology. Nat Rev Urol. 2015;12:570–584.
- Amabile-Cuevas C. Antibiotic resistance in Mexico: a brief overview of the current status and its causes. J Infect Dev Ctries. 2010;4:126–131.
- Baindara P, Kapoor A, Korpole S, et al. Cysteine-rich low molecular weight antimicrobial peptides from Brevibacillus and related genera for biotechnological applications. World J Microbiol Biotechnol. 2017;6:1–7.
- Bensman TJ, Jayne JG, Sun M, et al. Efficacy of rhesus theta (θ)-defensin-1 in experimental models of Pseudomonas aeruginosa lung infection and inflammation. Antimicrob Agents Chemother. Forthcoming 2017.
- Wang J, Tang L, Somasundaran P, et al. Highly effective antibacterial activity by the synergistic effect of three dimensional ordered mesoporous carbon-lysozyme composite. J Colloid Interface Sci. 2017;503:131–141.
- Le Ouay B, Stellacci F. Antibacterial activity of silver nanoparticles: a surface science insight. Nano Today. 2015;10:339–354.
- Singh R, Shedbalkar UU, Wadhwani SA, et al. Bacteriagenic silver nanoparticles: synthesis, mechanism, and applications. Appl Microbiol Biotechnol. 2015;99:4579.
- Panáček A, Smékalová M, Večeřová R, et al. Silver nanoparticles strongly enhance and restore bactericidal activity of inactive antibiotics against multiresistant Enterobacteriaceae. Colloids Surf B Biointerfaces. 2016;142:392–399.
- Tan JBL, Lim YY. Critical analysis of current methods for assessing the in vitro antioxidant and antibacterial activity of plant extracts. Food Chem. 2015;172:814–822.
- Kuppusamy P, Yusoff MM, Maniam GP, et al. Biosynthesis of metallic nanoparticles using plant derivatives and their new avenues in pharmacological applications–an updated report. Saudi Pharm J. 2016;24:473–484.
- Ravichandran V, Vasanthi S, Shalini S, et al. Green synthesis of silver nanoparticles using Artocarpus altilis leaf extract and the study of their antimicrobial and antioxidant activity. Mater Lett. 2016;180:264–267.
- Saravanakumar A, Peng MM, Ganesh M, et al. Low-cost and eco-friendly green synthesis of silver nanoparticles using Prunus japonica (Rosaceae) leaf extract and their antibacterial, antioxidant properties. Artif Cells Nanomed Biotechnol. 2016;45:1165–1171.
- Surendra TV, Roopan SM, Arasu MV, et al. RSM optimized Moringa oleifera peel extract for green synthesis of M. oleifera capped palladium nanoparticles with antibacterial and hemolytic property. J Photochem Photobiol B. 2016;162:550–557.
- Khan ZUH, Khan A, Chen YM, et al. Biomedical applications of green synthesized Nobel metal nanoparticles. J Photochem Photobiol B. 2017;173:150–164.
- Tahir K, Nazir S, Ahmad A, et al. Facile and green synthesis of phytochemicals capped platinum nanoparticles and in vitro their superior antibacterial activity. J Photochem Photobiol B. 2017;166:246–251.
- Vanaraj S, Jabastin J, Sathiskumar S, et al. Production and characterization of bio-AuNPs to induce synergistic effect against multidrug resistant bacterial biofilm. J Clust Sci. 2017;28:227–244.
- Vimbela GV, Ngo SM, Fraze C, et al. Antibacterial properties and toxicity from metallic nanomaterials. Int J Nanomedicine. 2017;12:3941.
- Cowan MM. Plant products as antimicrobial agents. Clin Microbiol Rev. 1999;12:564–582.
- Akthar MS, Degaga B, Azam T. Antimicrobial activity of essential oils extracted from medicinal plants against the pathogenic microorganisms: a review. Issues Biol Sci Pharm Res. 2014;2:1–7.
- Köksal E, Tohma H, Kılıç Ö, et al. Assessment of antimicrobial and antioxidant activities of Nepeta trachonitica: analysis of its phenolic compounds using HPLC-MS/MS. Sci Pharm. 2017;85:24.
- Bonifácio BV, da Silva PB, Ramos MA, et al. Nanotechnology-based drug delivery systems and herbal medicines: a review. Int J Nanomed. 2014;9:1.
- Abdelhady NM, Badr KA. Comparative study of phenolic content, antioxidant potentials and cytotoxic activity of the crude and green synthesized silver nanoparticles′ extracts of two Phlomis species growing in Egypt. J Pharmacognosy Phytochem. 2016;5:377.
- Olteanu RL, Nicolescu CM, Bumbac M. Influence of phytochemical reductive capacity on ultraviolet-visible spectroscopic behavior of silver nanoparticles. Anal Lett. Forthcoming 2017.
- Klančnik A, Piskernik S, Jeršek B, et al. Evaluation of diffusion and dilution methods to determine the antibacterial activity of plant extracts. J Microbiol Methods. 2010;81:121–126.
- Othman M, San Loh H, Wiart C, et al. Optimal methods for evaluating antimicrobial activities from plant extracts. J Microbiol Methods. 2011;84:161–166.
- Rajeshkumar S, Malarkodi C, Vanaja M, et al. Anticancer and enhanced antimicrobial activity of biosynthesized silver nanoparticles against clinical pathogens. J Mol Struct. 2016;1116:165–173.
- Singh P, Kim YJ, Wang C, et al. The development of a green approach for the biosynthesis of silver and gold nanoparticles by using Panax ginseng root extract, and their biological applications. Artif Cells Nanomed Biotechnol. 2015;44:1150–1157.
- Pal S, Tak YK, Song JM. Does the antibacterial activity of silver nanoparticles depend on the shape of the nanoparticle? A study of the gram-negative bacterium Escherichia coli. Appl Environ Microbiol. 2007;73:1712–1720.
- Adabi M, Naghibzadeh M, Adabi M, et al. Biocompatibility and nanostructured materials: applications in nanomedicine. Artif Cells Nanomed Biotechnol. 2016;45:833–842.
- Helmlinger J, Sengstock C, Grosz-Heitfeld C, et al. Silver nanoparticles with different size and shape: equal cytotoxicity, but different antibacterial effects. RSC Advances. 2016;6:18490–18501.
- El Badawy AM, Silva RG, Morris B, et al. Surface charge-dependent toxicity of silver nanoparticles. Environ Sci Technol. 2010;45:283–287.
- Abbaszadegan A, Ghahramani Y, Gholami A, et al. The effect of charge at the surface of silver nanoparticles on antimicrobial activity against gram-positive and gram-negative bacteria: a preliminary study. J Nanomater. 2015;16:53.
- Chang T-Y, Chen C-C, Cheng K-M, et al. Trimethyl chitosan-capped silver nanoparticles with positive surface charge: their catalytic activity and antibacterial spectrum including multidrug-resistant strains of Acinetobacter baumannii. Colloids Surf B Biointerfaces. 2017;155:61–70.
- Panáček A, Smékalová M, Kilianová M, et al. Strong and nonspecific synergistic antibacterial efficiency of antibiotics combined with silver nanoparticles at very low concentrations showing no cytotoxic effect. Molecules. 2015;21:26.
- Deng H, McShan D, Zhang Y, et al. Mechanistic study of the synergistic antibacterial activity of combined silver nanoparticles and common antibiotics. Environ Sci Technol. 2016;50:8840–8848.
- Rivero-Cruz I, Acevedo L, Guerrero JA, et al. Antimycobacterial agents from selected Mexican medicinal plants. J Pharm Pharmacol. 2005;57:1117–1126.
- Rodríguez-León E, Iñiguez-Palomares R, Navarro RE, et al. Synthesis of silver nanoparticles using reducing agents obtained from natural sources (Rumex hymenosepalus extracts). Nanoscale Res Lett. 2013;8:1.
- Wu H, He L, Gao M, et al. One-step in situ assembly of size-controlled silver nanoparticles on polyphenol-grafted collagen fiber with enhanced antibacterial properties. New J Chem. 2011;35:2902–2909.
- Rodríguez RÁ, Lahoz IR, Faza ON, et al. Theoretical and experimental exploration of the photochemistry of resveratrol: beyond the simple double bond isomerization. Org Biomol Chem. 2012;10:9175–9182.
- Huang X, Wu H, Liao X, et al. One-step, size-controlled synthesis of gold nanoparticles at room temperature using plant tannin. Green Chem. 2010;12:395–399.
- Shukla SP, Roy M, Mukherjee P, et al. Size selective green synthesis of silver and gold nanoparticles: enhanced antibacterial efficacy of resveratrol capped silver sol. J Nanosci Nanotechnol. 2016;16:2453–2463.
- Durán N, Silveira CP, Durán M, et al. Silver nanoparticle protein corona and toxicity: a mini-review. J Nanobiotechnol. 2015;13:55.
- Hansen U, Thünemann AF. Characterization of silver nanoparticles in cell culture medium containing fetal bovine serum. Langmuir. 2015;31:6842–6852.
- Satyavani K, Gurudeeban S, Ramanathan T, et al. Biomedical potential of silver nanoparticles synthesized from calli cells of Citrullus colocynthis (L.) Schrad. J Nanobiotechnol. 2011;9:43.
- Majumdar R, Bag BG, Maity N. Acacia nilotica (Babool) leaf extract mediated size-controlled rapid synthesis of gold nanoparticles and study of its catalytic activity. Int Nano Lett. 2013;3:53.
- Agnihotri S, Mukherji S, Mukherji S. Size-controlled silver nanoparticles synthesized over the range 5–100 nm using the same protocol and their antibacterial efficacy. RSC Advances. 2014;4:3974–3983.
- Wu H, Huang X, Gao M, et al. Polyphenol-grafted collagen fiber as reductant and stabilizer for one-step synthesis of size-controlled gold nanoparticles and their catalytic application to 4-nitrophenol reduction. Green Chem. 2011;13:651–658.
- Shirley AD, Dayanand A, Sreedhar B, et al. Antimicrobial activity of silver nanoparticles synthesized from novel Streptomyces species. Dig J Nanomater Biostruct. 2010;5:447–451.
- Ahmed S, Ahmad M, Swami BL, et al. A review on plants extract mediated synthesis of silver nanoparticles for antimicrobial applications: a green expertise. J Adv Res. 2016;7:17–28.
- Nayak D, Ashe S, Rauta PR, et al. Bark extract mediated green synthesis of silver nanoparticles: evaluation of antimicrobial activity and antiproliferative response against osteosarcoma. Mater Sci Eng C Mater Biol Appl. 2016;58:44–52.
- Cui Y, Oh YJ, Lim J, et al. AFM study of the differential inhibitory effects of the green tea polyphenol (-)-epigallocatechin-3-gallate (EGCG) against Gram-positive and Gram-negative bacteria. Food Microbiol. 2012;29:80–87.
- Rempe CS, Burris KP, Lenaghan SC, et al. The potential of systems biology to discover antibacterial mechanisms of plant phenolics. Front Microbiol. 2017;8:422.
- Hwang D, Lim YH. Resveratrol antibacterial activity against Escherichia coli is mediated by Z-ring formation inhibition via suppression of FtsZ expression. Sci Rep. 2015;5:10029.
- Greulich C, Braun D, Peetsch A, et al. The toxic effect of silver ions and silver nanoparticles towards bacteria and human cells occurs in the same concentration range. RSC Adv. 2012;2:6981–6987.
- Orlowski P, Krzyzowska M, Zdanowski R, et al. Assessment of in vitro cellular responses of monocytes and keratinocytes to tannic acid modified silver nanoparticles. Toxicol In Vitro. 2013;27:1798–1808.
- Lankoff A, Sandberg WJ, Wegierek-Ciuk A, et al. The effect of agglomeration state of silver and titanium dioxide nanoparticles on cellular response of HepG2, A549 and THP-1 cells. Toxicol Lett. 2012;208:197–213.
- Butler KS, Peeler DJ, Casey BJ, et al. Silver nanoparticles: correlating nanoparticle size and cellular uptake with genotoxicity. Mutagenesis. 2015;30:577–591.
- Krystek P, Kettler K, van der Wagt B, et al. Exploring influences on the cellular uptake of medium-sized silver nanoparticles into THP-1 cells. Microchem J. 2015;120:45–50.
- Kettler K, Giannakou C, de Jong WH, et al. Uptake of silver nanoparticles by monocytic THP-1 cells depends on particle size and presence of serum proteins. J Nanopart Res. 2016;18:286.