Abstract
Breast cancer stem cells (BCSCs) are heterogeneous subpopulation of tumour initiating cells within breast tumours. They are spared even after chemotherapy and responsible for tumour relapse. Targeting BCSCs is, therefore, necessary to achieve radical cure in breast cancer. Despite the availability of agents targeting BCSCs, their clinical application is limited due to their off-target effects and bioavailability issues. Nanotechnology based drug carriers (nanocarriers) offer various advantages to deliver anti-BCSCs agents specifically to their target sites by overcoming their bioavailability issues. In this review, we describe various strategies for targeting BCSCs using nanocarriers.
Graphical Abstract
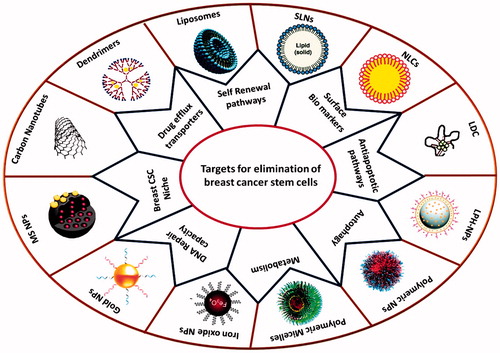
Introduction
Breast cancer is the most frequently diagnosed cancer and is the second leading cause of cancer deaths in women. Metastasis and relapse of tumour are emerging as major challenges in treatment of breast cancer [Citation1,Citation2]. Compelling body of evidences suggests that, small population of tumour initiating cells within tumours called breast cancer stem cells (BCSCs) are responsible for tumour relapse and metastasis [Citation3–6]. The quiescence, expression of higher levels of drug efflux transporters and high DNA repair capacity of BCSCs makes them to resist apoptosis by chemotherapeutic agents [Citation7,Citation8]. The pool of BCSCs which are spared after chemotherapy will convert into tumour cells in future and results in tumour relapse [Citation9]. In addition, BCSCs promote metastasis by induction epithelial-to-mesenchymal transition (EMT), in which, epithelial cells lose their intercellular adhesion, accompanied by gain of invasive and migratory properties, which is a pre-requisite for metastasis [Citation10–13] (). It is, therefore, necessary to eradicate BCSCs to achieve radical cure in breast cancer.
Figure 1. The role of BCSCs in tumour relapse and metastasis. (A) Conventional chemotherapeutic agents for treatment of breast cancer eliminate bulk tumour cells (non-BCSCs) while sparing tumour initiating, BCSCs and results in tumour relapse; (B) The transition from an epithelial state to a mesenchymal state (EMT) in BCSCs (or metastatic cells) occurs by activation of transcription factors such as Snail, Slug and Zeb and promotes dissemination. This EMT phenotype of cells enters blood circulation and survives by evading immune surveillance, then enter target organ. These cells can remain in a dormant state, lasting from months to decades or re-acquire epithelial properties by undergoing mesenchymal to epithelial transition (MET) in target organ to initiate secondary tumour growth; (C) Key signalling pathways implicated in the survival of BCSCs.
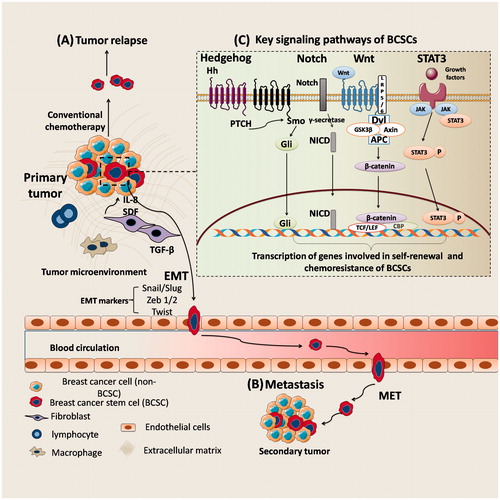
Expression of surface biomarkers (CD44, CD133, EpCAM and ALDH1), self-renewal pathways (Wnt, Notch, TGF-β, Hedgehog, Bmi-1 and STAT3), drug efflux transporters (ABCG2 and ABCB1), anti-apoptotic proteins (Bcl2, survivin etc.), autophagy, metabolism and specialized microenvironment are the important biological features of BCSCs [Citation7,Citation14,Citation15] (). In recent years, a number of anti-BCSC agents have been proposed to eliminate BCSCs by altering these features (). However, these anti-BCSC agents have failed in clinical setting due to their poor water solubility, short circulation time, inconsistent stability and off-target effects [Citation16–18]. There is a need, therefore, for suitable drug delivery systems to improve bioavailability and carry anti-BCSC agents specifically to BCSCs without off-target effects. Due to advantage of site specific delivery and ability to improve bioavailability and stability of drugs, nanocarriers have attracted attention of many researchers as carriers for targeting BCSCs: (1) surface markers, (2) self-renewal signalling pathways, (3) drug efflux transporters, (4) metabolism, (5) autophagy process, (6) anti-apoptotic proteins and (7) microenvironment [Citation18–23] (). In the present review, we discuss the current developments in these nanocarrier based approaches.
Figure 2. Possible therapeutic approaches for eradication of BCSCs using nanocarriers. (1) Targeting BCSC surface markers; (2) Silencing self-renewal pathways; (3) inhibiting drug efflux transporters; (4) inhibiting anti-apoptotic proteins; (5) altering metabolism; (6) targeting autophagy process; (7) disruption of vascular niche of the BCSCs to impair the specialized microenvironment housing.

Table 1. Molecular targets of BCSCs.
Table 2. Various anti-BCSCs agents and their mechanism of action for elimination of BCSCs.
Targeting BCSCS using nanocarriers
Nanocarriers offer various advantages for targeting tumours by overcoming the bioavailability issued of chemotherapeutic agents [Citation24]. They can passively accumulate at the tumour site owing to the leaky vasculature of tumour [Citation25]. In addition, active targeting by surface modification of suitable ligands for tumour biomarkers improves the site specificity of nanocarriers [Citation26–28]. Therefore, nanocarriers are being widely employed in recent years for tumour targeted drug delivery. With the evolution of “cancer stem cell model” in recent years several researchers have also applied nanocarriers for targeting CSCs [Citation29]. In this section, we discuss nanocarrier based strategies for targeting BCSCs.
Targeting surface biomarkers of BCSCs using nanocarriers
BCSCs express specific biological markers on their surface. Fluorescence-activated cell sorting (FACS) uses these biomarkers to sort BCSCs [Citation5]. Cluster of differentiation 44 (CD44), cluster of differentiation 133 (CD133), epithelial cellular adhesion molecule (EpCAM) and aldehyde dehydrogenase1 (ALDH1) are the important surface markers of BCSCs (). Recently, researchers focused on nanocarrier mediated targeting of these biomarkers, to deliver drugs specifically to BCSCs.
CD44 targeted nanocarriers
Biopolymers like hyaluronic acid and chitosan were being employed as ligands for active targeting of CD44 biomarker [Citation30]. In a recent study, Dong Wang et al. have developed hyaluronan (HA) modified mesoporous silica nanoparticles (MSNs) loaded with 8-hydroxyquinoline supported by lipid bilayers containing docetaxel to eliminate BCSCs. In this study, HA modification resulted in enhanced uptake of nanoparticles in BCSCs via CD44 receptor. In the MCF-7 xenograft mice, this combination therapy enhanced antitumour efficacy and reduced off-target effects () [Citation31]. In another study, Goodarzi et al. have developed a macromolecular conjugate of docetaxel with HA to selectively target CD44 + BCSCs The developed bioconjugates are effective in eliminating BCSCs along with non-BCSCs [Citation32]. Ruo-Jing Li et al. have reported that, stealth liposomes of All-trans retinoic acid (ATRA) were able to decrease the expression of CD44 in the BCSCs in vitro, and prevented tumour relapse in breast cancer [Citation9]. Wei Rao et al. have recently developed a doxorubicin-loaded polymeric nanoparticle surface modified with chitosan to specifically target the CD44 surface receptors of BCSCs [Citation33] ().
Figure 3. The preparation procedure of HA modified mesoporous silica nanoparticles (MSN). MSN modified with an amine-containing silane were first loaded with docetaxel or 8-hydroxy quinoline. Liposomes were prepared with different components consisting of DOPC, DOPE, cholesterol and 18:0 PEG-2000 PE (60:5:30:5 mass ratio). HA was conjugated to the liposomes by an EDC-based method. Finally, hyaluronan modified mesoporous silica nanoparticle-supported lipid bilayers (HA-MSS) was constructed by fusing HA-modified liposomes to drug loaded MSN [Citation31] (Reproduced with permission. Copy Right, Elsevier 2013).
![Figure 3. The preparation procedure of HA modified mesoporous silica nanoparticles (MSN). MSN modified with an amine-containing silane were first loaded with docetaxel or 8-hydroxy quinoline. Liposomes were prepared with different components consisting of DOPC, DOPE, cholesterol and 18:0 PEG-2000 PE (60:5:30:5 mass ratio). HA was conjugated to the liposomes by an EDC-based method. Finally, hyaluronan modified mesoporous silica nanoparticle-supported lipid bilayers (HA-MSS) was constructed by fusing HA-modified liposomes to drug loaded MSN [Citation31] (Reproduced with permission. Copy Right, Elsevier 2013).](/cms/asset/9a0c49a4-cba0-47cf-9458-8804330b1002/ianb_a_1366337_f0003_c.jpg)
Table 3. Targeting BCSC surface markers using nanocarriers.
CD133 targeted nanocarriers
Swaminathan et al. have recently reported that, encapsulation of paclitaxel in CD133 antibody conjugated polymeric nanoparticles specifically targeted CD133+ BCSCs population and improved therapeutic efficacy compared to free paclitaxel. In addition of the CD133 targeted nanocarriers prevented tumour-recurrence in xenograft mice model [Citation34] ().
EpCAM targeted nanocarriers
In order to specifically delivery SiRNA to EpCAM + BCSCs, Mohammadi et al. have devised, single-walled carbon nanotubes (SWCNTs) functionalized with EpCAM aptamer and piperazine–polyethylenimine derivative [Citation35]. In another study, Alibolandi et al. have developed EpCAM aptamer conjugated polyethylene glycol–poly lactic acid-co-glycolic acid (PEG-PLGA) nanopolymersomes for targeted delivery of Doxorubicin to human breast cancer cells. They reported that, DOX loaded aptamer conjugated nanopolymersomes exhibited efficient cell uptake and internalization, and showed a significant cytotoxicity toward EpCAM-positive breast cancer cells [Citation36]. Recently, Subramanian et al. have developed polyethyleneimine (PEI) nanocomplex fabricated with EpCAM aptamer and EpCAM SiRNA to target EpCAM + cells. This nanocomplex exhibited greater efficiency in elimination of EpCAM + cells [Citation37] ().
Nanocarrier mediated silencing of self-renewal pathways in BCSCs
Self-renewal is one of the key mechanisms required for the survival of BCSCs. Wnt/β-catenin, Notch, Hedgehog (Hh), Transformin growth factor-β (TGF-β), signal transducer and activator of transcription factor 3 (STAT3) and B-cell-specific moloney-murine leukaemia virus integration site 1 (Bmi-1) are the key signalling pathways implicated in self-renewal and survival of BCSCs [Citation38] ().
Table 4. Targeting self-renewal pathways of BCSCs using nanocarriers.
Nanocarriers for silencing Wnt/β-catenin pathway
Wnt pathway activation occurs when Wnt ligand binds the transmembrane receptor, which in turn results in activation of the low-density lipoprotein-related receptor (LRP). This leads to the suppression of glycogen synthase kinase-3β (GSK-3β) protein, and improve the stability of β-catenin. It then forms a complex with the transcription factor/lymphocyte enhancer factor and activates the expression of its target genes such as c-terminus of myc protein (c-myc) and cyclin D1, involved in self-renewal of BCSCs [Citation39,Citation40]. β-catenin, is an important molecular target for silencing Wnt/β-catenin pathway. Several nanocarriers have been developed in recent years for silencing Wnt pathway by inhibiting β-catenin. For instance, Jingbin et al. have recently developed self-assembled poly (d,l-lactide-co-glycolide)/hyaluronic Acid block copolymer-based nanocarriers loaded with β-catenin inhibitor, sulforaphane (SFN) to inhibit self-renewal of BCSCs. These SFN-loaded nanocarriers were effective in silencing Wnt pathway in BCSCs than free SFN due to improved drug accumulation. In vivo studies also showed that, these nanocarriers had the strongest antitumour efficacy than free drug [Citation41]. In another study, to silence Wnt signalling Peng Zhao et al. devised a self-assembled nanoparticle system with immune tolerant elastin-like polypeptide (iTEP) and salinomycin, a Wnt/β-catenin signalling pathway inhibitor for elimination of BCSCs. Salinomycin was encapsulated into amphiphilic iTEP − Salinomycin conjugates. The nanoconjugates of salinomycin significantly reduced the tumour growth in 4T1-orthotopic breast cancer model [Citation42,Citation43].
Nanocarriers for silencing notch signalling pathway
Because of its key role in self-renewal of BCSCs, Notch signalling has attracted research attention to prevent tumour relapse and metastasis [Citation44,Citation45]. Binding of ligands results in cleavage of Notch receptor by γ-secretase enzyme to release Notch intracellular domain (NICD). NICD then translocates into nucleus and then activates Notch target genes [Citation46]. γ-Secretase, therefore, is an important target to prevent proteolysis of Notch receptors and to suppress the Notch activity in BCSCs. In a recent study, it was demonstrated that, glucose functionalized MSNPs carrying γ-secretase inhibitor, specifically inhibited notch signalling in BCSCs and non-BCSCs to inhibit their self-renewal capacity and to prevent tumour recurrence [Citation20]. Recently, Yang et al. developed Multifunctional Core/Shell nanoparticles Cross-linked Polyetherimide-folic acid to deliver Notch-1 SiRNA to breast cancer cells [Citation47]. Nanocarriers treated MDA-MB-231 cells exhibited significantly decreased expression of Notch-1.
Nanocarriers for silencing transforming growth factor-β (TGF-β) pathway
TGF-β signalling exerts tumour suppressor effects in normal cells and early carcinomas. However, in advanced tumours, the protective and cytostatic effects of TGF-β will be lost (as a result of mutations) and promotes tumour progression, invasion and metastasis. TGF-β signalling, therefore, has dual role as both tumour suppressor and promoter [Citation48]. Recently, Zuo et al. have reported that inhibition of TGF-β signalling pathway using small molecule inhibitor, LY364947 promoted the penetration and improved the efficacy of nanocarriers with siRNA against oncogene, polo-like kinase 1 (Plk1) [Citation49]. In another study by Liu et al., gold-metallofullerenol based nanomaterial effectively eliminated BCSCs by inhibiting TGF-β signalling [Citation50].
Nanocarriers for silencing hedgehog (Hh) signalling pathway
In Hh pathway, the activation of Smoothened (Smo), a seven-pass transmembrane receptor is necessary for signalling process. Binding of Hh ligand to Smo activates the glioma associated oncogene (Gli) family of transcription factors (Gli1/2/3) to carry out the further downstream signalling required for self-renewal of BCSCs [Citation51]. Smo and Gli family of transcription factors are the important targets for inhibition of Hh pathway. Nanocarriers are being widely employed to deliver Smo inhibitors and silence Hh pathway.
Hu et al. have developed amphipathic and redox-responsive polymer particles for co-delivery of cyclopamine (Smo inhibitor) and Doxorubicin to eradicate BCSCs and non-BCSCs [Citation52]. The polymeric nanocarrier system composed of a hydrophobic PLGA head and a hydrophilic HA segment linked by a bioreducible disulphide bond. This nanocarrier system diminished the number and size of tumour spheres and also achieved active targeting of BCSCs. In addition, they enhanced tumour regression and prolonged survival compared to monotherapy using orthotopic mammary fat pad tumour growth model. In another study, it was reported that, cyclopamine inhibited Hh signalling in BCSCs and enhanced antitumour activity when given in combination with 177Lu-mediated radiotherapy. The authors demonstrated that combined chemoradiation therapy is an effective strategy for elimination of both non-BCSCs and BCSCs in vitro and in vivo [Citation53].
Nanocarriers for silencing IL-6/JAK/STAT3 signalling pathway
IL-6/JAK/STAT3 is the canonical STAT3 activation signalling pathway, reported to be deregulated in cancer. Since the receptor of IL-6 does not contain a kinase catalytic domain, it induces STAT3 phosphorylation by activating members of the JAK family [Citation54–58]. It was demonstrated that inhibition of STAT3 pathway suppressed growth of xenograft tumours. In addition, it has been reported that IL-6/JAK1/STAT3 signalling pathway plays an important role in activation of EMT [Citation59,Citation60]. Misra et al. have demonstrated STAT3 modulation using mixed polymeric micelles of niclosamide (an inhibitor of STAT3 phosphorylation), to inhibit BCSCs and non-BCSCs. They have evaluated the efficacy of developed micelles in MDA-MB231 breast cancer cells. The niclosamide micelles were reported to eliminate CD44 + BCSCs and also improved the cytotoxicity of niclosamide in non-BCSCs [Citation61].
Nanocarriers for induction of apoptosis in BCSCs
Deregulation of apoptosis and anti-apoptotic (survival) signalling pathways is a characteristic of cancer and a critical determinant of efficacy of chemotherapy [Citation62]. The B-cell lymphoma2 (Bcl2), FLICE like inhibitory protein (cFLIP), nuclear factor-κ-B (NFκB), phosphatase and tensin homolog (PTEN), mammalian target of rapamycin(m-TOR), death receptors (DR)-4/5 proteins are the well-characterized regulators for induction apoptosis in BCSCs [Citation21,Citation24,Citation63]. Nanocarrier mediated induction of apoptosis by downregulation of anti-apoptotic proteins or upregulation of apoptotic proteins specifically in BCSCs using nanocarriers is an attractive strategy. Chen et al. have proposed an inorganic nanoparticle system containing Doxorubicin and Bcl2 SiRNA to improve the efficacy of chemotherapy by eliminating drug resistant cancer cells [Citation64]. In another recent study by Zhou et al., it was reported that, dual responsive mesoporous nanoparticles (MSNs) of Doxorubicin and Bcl2 SiRNA improved the efficacy of chemotherapy. The MSNs were prepared by modification of polyethylenimine–polylysine copolymers (PEI-PLL) via the disulphide bonds. To achieve active targeting folate (FA)-linked polyethylene glycol (FA-PEG) was conjugated to PEI-PLL. The FA-conjugated MSNs (MSNs-PPPFA) showed significantly enhanced intracellular uptake in folate receptor overexpressed MDA-MB-231 breast cancer cells than non-targeted counterparts and resulted in enhanced tumour regression because of downregulation of Bcl2 protein [Citation23]. Shi-Yong Li et al. have developed, nanoparticles (NPs) of decitabine (DAC) for chemosensitization of BCSCs to DOX therapy and to inhibit ALDH1 expression [Citation65]. In vitro studies revealed that treatment with nanoparticles loaded with DAC (NPDAC) combined with nanoparticles loaded with doxorubicin (NPDOX) reduced the proportion of BCSCs in the mammospheres of MDA-MB-231 cells, and overcame the drug resistance. In another study, a non-ionic surfactant (niosome) based co-delivery system containing Bcl2-SiRNA and ABCG2-SiRNA in combination with Doxorubicin to down regulate the ABCG2 and simultaneously induces apoptosis in BCSCs and non-BCSCs [Citation21]. Recently, Shen et al. have devised a nanoparticle delivery system of proteasome inhibitor Bortezomib (BTZ) to induce apoptosis by disrupting protein homeostasis in BCSCs. BTZ nanoparticles (NPBTZ) prolonged circulation half-life of BTZ, improved cell uptake and enhanced tumour regression in both BCSCs and non-BCSCs [Citation66]. In another study, it was reported that, the combination of vinorelbine stealthy liposomes and parthenolide stealthy liposomes resulted in simultaneous eradication of both BCSCs and non-BCSCs by affecting their apoptosis [Citation67]. Zhang et al. have demonstrated that, targeting of daunorubicin and quinacrine using liposome resulted in elimination of BCSC and prevented of tumour relapse. The authors reported that, liposomes of daunorubicin and quinacrine resulted in prolonged circulatory effect and are selectively accumulated in mitochondria and induced apoptosis by activating pro-apoptotic protein Bax, decreasing of mitochondrial membrane potential (ΔΨm), releasing and activating caspase-9 and its downstream caspases-3 in BCSCs [Citation68]. Sadhuka et al. have recently devised super paramagnetic iron oxide nanoparticles (SPIONs) to promote magnetic hyperthermia and induce reactive oxygen species (ROS) mediated apoptosis in BCSCs. Authors have demonstrated that SPION-transduced hyperthermia decreases side population cells, ADLH expression, tumour sphere formation, and in vivo xenotransplantation. In this study, the authors found ROS levels were increased upon hyperthermia in proportional to the time of the magnetic field exposure [Citation69].
Inhibition of drug efflux transporters of BCSCs
ABCB1 or P-glycoprotein (P-gp) and ABCG2 or breast cancer resistance protein (BCRP) are important drug efflux transporters implicated in the chemoresistance in breast cancer [Citation70,Citation71]. Inhibitors of ABCG2, like Fumitremorgin C (FTC), Tryprostatin-A, Tariquidar, have been proposed to kill BCSCs in order to prevent chemoresistance. However, their low bioavailability and off-target effects hindered their clinical application [Citation72–76].
Recently, it was reported that, pluronic block co-polymers have ability to overcome drug efflux and sensitize BCSCs to chemotherapy due to their ability to inhibit drug efflux transporters by inhibition of ATP synthesis in mitochondria of tumour cells [Citation77–81]. Nanocarriers comprising pluronic block co-polymers are, therefore, being widely employed for enhancing drug accumulation and chemosensitization of BCSCs. In a recent study, Wang et al. have developed stimuli (pH and thermal) responsive nanoparticles comprising Pluronic F127, PLGA and chitosan for co-delivery of doxorubicin and irinotecan to eliminate BCSCs. Besides minimizing efflux of drug from BCSCs, the nanoparticles also affected the activity of topoisomerases-I & II and resulted in efficient destruction of the BCSCs both in vitro and in vivo [Citation82]. Sun et al. have demonstrated an enhanced efficacy of chemotherapy by simultaneous suppression of multi drug resistance (MDR) proteins and anti-apoptotic genes using niosomes based co-delivery of ABCG2 SiRNA and Bcl2 SiRNA along with Doxorubicin chemotherapy [Citation21]. In another report, novel cationic poly (lactide-co-glycolide) nanoformulation of MDR SiRNA reversed the MDR in vitro and enhanced the efficacy of Doxorubicin chemotherapy [Citation83]. Recent research, therefore, suggest that, inhibition of drug efflux transporters using nanocarriers present an attractive strategy for sensitizing BCSCs to chemotherapeutic agents.
Targeting BCSCs metabolism
Inhibition of the mitochondrial metabolic function is one of the approaches for elimination of BCSCs [Citation84,Citation85]. Hexokinase 2 (HK2), which catalyzes the first step of glucose metabolism is the important target for altering the metabolism in BCSCs [Citation86]. It was recently reported that, metformin (anti-diabetic drug) inhibit HK2 and thereby enhanced the effects of chemotherapy [Citation87]. Considering the potential of metformin to kill BCSCs, Goodarzi et al. synthesized a hyaluronic (HA) acid conjugated metformin and docetaxel to kill both BCSCs and cancer cells. The synthesized polymer–drug conjugates were characterized using NMR, FTIR, DSC and GPC. The synthesized HA–drug conjugates have potential for CD44 mediated drug targeting of both BCSCs and non-BCSCs [Citation22]. In another recent study, it was reported that, metformin loaded solid lipid nanoparticles (SLNs) have efficient cellular and mitochondrial uptake in MCF-7 breast cancer cells. In this study, metformin was successfully incorporated into SLNs by an emulsification and low-temperature solidification method. The pharmacokinetics results showed that SLNs could improve the bioavailability of metformin [Citation88].
Figure 4. Potential nanocarrier systems for efficient targeting of BCSCs. (A) Nanocarriers serve as vehicles for therapeutic agents and increase drug accumulation in tumour tissues via EPR effect. (B) Surface modification of nanocarriers using suitable ligand can reduce off-target effects. (C) Stimuli-responsive nanoparticle systems, which are activated upon exposure to tumour microenvironment may further enhance the accumulation of drugs and also increase cellular internalisation. (D) Nanocarrier systems for co-delivery of dual drugs can improve therapeutic efficacy by simultaneous targeting of both BCSCs and non-BCSCs.
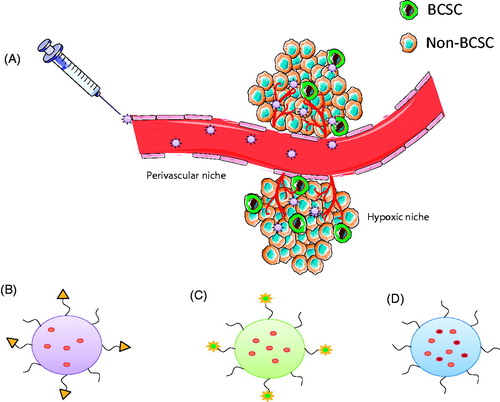
Autophagy
Autophagy plays an important role in breast cancer initiation or transformation of mammary epithelial cells, chemoresistance and metastasis [Citation89]. It was reported that treatment with autophagy inhibitors (e.g. Chloroquine) or silencing of autophagy-associated genes affects stem cell renewal, differentiation and stress-resistant abilities and enhanced the sensitivity to chemotherapeutic agents by elimination of BCSCs [Citation90,Citation91]. In a recent study, Rong Sun et al. have reported that nanocarrier mediated autophagy inhibition significantly improved the efficacy of chemotherapeutic agents against BCSCs. In this study, they prepared nanocarrier system using PEG-PLA co polymers to deliver the autophagy inhibitor chloroquine (CQ) in combination with Doxorubicin and Docetaxel. The authors reported that, the developed nanocarriers significantly prolonged the circulation half-life of CQ and enhanced the accumulation of drugs in tumour tissues. Further, the combination therapy resulted in enhanced tumour regression by eliminating non-BCSCs as well as BCSCs in MDA-MB-231 orthotropic tumour mice model [Citation92].
Targeting BCSCs microenvironment using nanocarriers
BCSCs require a specialized microenvironment or niche which is regulated by various factors for their survival. The factor that regulate BCSCs microenvironment include fibroblast stimuli, immune cells, autocrine signals and extracellular matrix (ECM) components, as well as physical/chemical factors such as oxygen pressure, nutrients levels and low pH [Citation93,Citation94]. Growth factors and cytokines released by tumour cells and cancer-associated fibroblasts and immune cells have strong effects on the survival and metastasis of BCSCs by maintaining microenvironment [Citation95,Citation96]. Nanocarriers have been developed recently to disrupt these components of BCSC microenvironment. For instance, it was reported that, nanocarrier mediated inhibition of lysyl oxidase (an enzyme required for collagen cross-linking and rigidity of extracellular matrix) results in disruption of tumour microenvironment [Citation97,Citation98]. In another study, PLA microspheres loaded with IL-12, TNF-α and granulocyte-macrophage colony-stimulating factor (GM-CSF) was used as anti-tumoural therapy in a murine breast cancer model. The microspheres were administered by intratumoural injection, where they initiated a major infiltration of polymorphonuclear cells and CD8 + cytotoxic T-cells that resulted in increased the number of tumour free mice at the end of the study. This therapy also resulted in specific memory T-cells that could prevent tumour relapse in a murine breast cancer model [Citation99].
Future directions
Compelling body of evidences suggest that, there is a clear promise in application of nanocarriers for detection, targeting, and eradication of BCSCs. However, in order to improve efficacy in specific targeting of BCSCs, nanocarriers should be rationally designed and must possess long circulation time, enhanced tumour accumulation and sufficient internalization in BCSCs. In this section, we discuss several potential design strategies to improve efficacy of future nanocarriers in targeting BCSCs ().
Enhancing tumour accumulation using long circulating nanocarriers
Modification of nanocarriers with poly ethylene glycol (PEG) or other hydrophilic polymer modifications is a widely recognized approach for prolonging circulation time, by stabilizing nanoparticles, minimising non-specific binding and reducing clearance by immune cells [Citation100]. Prolonging circulation time of the nanocarriers may some extent mitigate the effect of their poor extravasation into tissues due to increased exposure of the therapeutics in the body [Citation101].
Enhancing intracellular penetration usingstimuli-responsive nanocarriers
Although PEGylation improves the accumulation of nanocarriers at tumour site, it was reported that, PEG reduces the cellular uptake of nanoparticles, affects intracellular trafficking and also hinders anti-cancer efficacy [Citation102]. Stimuli-responsive nanocarriers with cleavable PEG moiety have been proposed in recent years to overcome this limitation. Stimuli-responsive nanocarriers target cancer cells based on characteristic microenvironmental changes occur in neoplastic diseases. These stimuli include lowered interstitial pH, and changes in the levels of certain components such as matrix metalloproteinases (MMPs) [Citation103]. For instance, environment-responsive liposomes anchored by phospholipids with cell-penetrating peptides and a pH-sensitive PEG shield have improved cellular internalization. The liposomes lost their PEG coating at low pH and were internalized in the cells via the exposed cell-penetrating peptide moieties [Citation104]. The application of stimuli-sensitive carriers to facilitate cellular internalization in BCSCs have rarely been reported, it could be a potential area worth further exploration.
Designing surface-tailored nanocarriers to reduceoff-target effects
Increasing body of evidence suggests that EPR effect does not provide specific targeting and sufficient intracellular delivery to BCSCs, which are located in the necrotic sites or otherwise deeply embedded tumour sites [Citation28]. The concept of active targeting approach by conjugating an appropriate ligand for specific tumour marker on the surface of nanocarriers is an attractive strategy for selective delivery and improved accumulation of drugs and also to reduce off-target effects [Citation105,Citation106]. However, the markers currently used to target nanocarriers to BCSCs were also reported to be expressed on normal stem cells, this further makes BCSC-specific nanocarriers less discriminative and also can result in undesired toxicities [Citation107]. Identifying highly specific BCSC markers is a major challenge as very few markers are available that exhibit sufficient selectivity in vivo. Active targeting should, therefore, be more comprehensively defined and evaluated to avoid the aforementioned pitfalls associated with receptor-specific targeting and to achieve the ultimate goal of enhanced efficacy. In addition, targeted nanocarriers must be able to reach the surfaces of BCSCs to interact with these cells through their targeting moieties, and this is possible only when there are no interactions of nanocarriers with biological objects in the body (cell surfaces, biomacromolecules, etc.). Therefore, the surface of nanocarriers must be modified with an “inert” polymer that would have minimal interactions with the cells, and then targeting moieties should be attached at the inert layer to allow for specific binding of the targeting moieties with their receptor. PEG is widely used inert polymer to minimize non-specific interaction of nanocarriers. However, there are clear indications that even this polymer attached to nanomaterials can activate complement, and otherwise engage the body immunity [Citation108].
Nanocarrier based nucleic acid delivery to BCSCs
SiRNA and miRNA are the two main nucleic acid therapeutic drugs, which act by suppressing the genes required for survival of cancerous cells. RNAi may also have poor specificity or off-target effects, posing potential safety concerns and sometimes decreasing the effectiveness of treatment [Citation109]. Nanocarriers are being employed to overcome the limitations associated with nucleic acid drugs [Citation110]. After being taken up by cells, nanocarriers carrying nucleic acids should be able to induce endosomal escape and release their load in cytosol.
Designing nanocarriers for co-delivery of drugs or nucleic acids to BCSCs
Nanocarriers can be tailored for encapsulating multiple pay loads for simultaneous targeting of different cell populations. Combination of different anticancer drugs or a drug and nucleic acid using nanocarriers is being studied in recent years for treating cancers [Citation19,Citation111,Citation112]. Similarly the nanocarrier-based delivery systems can also be explored for both identification and targeting BCSCs by co-encapsulation of a diagnostic and therapeutic agents. Although loading multiple drugs inside a single nanocarrier provides advantages in terms of less toxicity and better efficacy, this may result in complex pharmacokinetic and pharmacodynamics for each drug. When designing combination therapy these factors should be evaluated to justify the efficacy or synergistic effect achieved by the use of drug combination [Citation113]. Combinatorial nanocarriers can be designed to target multiple pathways simultaneously with a combination of inhibitors and conventional therapeutics.
Conclusion
BCSCs are the major cause of tumour relapse, metastasis and chemoresistance in breast cancer. As discussed in this review, many molecular targets like surface markers, proteins involved in self-renewal and apoptotic pathways, autophagy, metabolism and microenvironment have been identified for eradication of BCSCs. The current research is focused on developing anticancer agents against these targets to eliminate BCSCs and to achieve radical cure in breast cancer therapy. Although several agents are proposed to eliminate BCSCs, their clinical utility is limited due to their poor water solubility, instability and off-target effects. We have discussed the potential of nanocarrier based drug delivery systems to overcome the limitations associated with current anti BCSC agents. The overview of recent applications of nanocarriers for targeting BCSCs suggests that nanocarriers offer various advantages for targeting of BCSCs. However, application of nanocarriers for BCSC-targeting is in its infancy, and many issues need to be well studied in clinical setting.
Abbreviations | ||
ABC | = | ATP-Binding Cassette |
ATM | = | Ataxia Telangiectasia Mutated serine/threonine kinase |
Bcl2 | = | B-cell lymphoma 2 |
BCRP | = | Breast cancer resistance protein |
BCSCs | = | Breast cancer stem cells |
BIK | = | Bcl2 interacting killer |
Bmi-1 | = | B-cell-specific moloney murine leukaemia virus integration site 1 |
BMP2 | = | Bonje morphogenetic protein 2 |
CAIX | = | Carbonic anhydrase-IX |
CAT | = | Catalase |
CSL | = | CBF-1/RBPJ-κ in Homo sapiens/Mus musculus respectively, Suppressor of Hairless in Drosophila melanogaster, Lag-1 in Caenorhabditis elegans |
CDK | = | Cyclin dependent kinases |
ChKs | = | Checkpoint kinases |
c-myc | = | C-terminus of myc protein |
DHh | = | Desert Hedgehog |
DLL4 | = | Delta-like 4 ligand |
DOX | = | Doxorubicin |
DRs | = | Death receptors |
EMT | = | Epithelial to mesenchymal transition |
EpICD | = | EpCAM intracellular domain |
FAS | = | Fatty acid synthase |
FTC | = | Fumitremorgin C |
Gli | = | Glioma-associated oncogene |
GLUT | = | glucose transporter |
GM-CSF | = | granulocyte-macrophage colony-stimulating factor |
GPO | = | glutathione peroxidase |
GSK-3β | = | Glycogen synthase kinase 3 β |
HDR | = | Homology-directed recombination |
Hh | = | Hedgehog |
HK | = | Hexose kinase |
IHh | = | Indian Hedgehog |
IL | = | Interleukin |
JAK | = | Janus Kinase |
LRP | = | Low-density lipoprotein-related receptor |
MAML | = | Master mind like |
mTOR | = | Mammalian target of rapamycin |
Nanog | = | Gene named after the Tír na nÓg legend |
NHEJ | = | Non-homologous end joining |
NICD | = | Notch intracellular domain |
Non-BCSCs | = | Non-Breast cancer stem cells or bulk tumour cells |
Oct-4 | = | Octamer binding transcription factor |
PDZ | = | Dishevelled PDZ domain |
PI3-k | = | Phosphoinositide 3-kinase |
PTEN | = | Phosphatase and tensin homolog |
SCs | = | Normal Stem cells |
SHh | = | Sonic Hedgehog |
SMADs | = | Homologs of Sma and MAD proteins |
Smo | = | Smoothened |
SOD | = | Superoxide dismutase |
SOX | = | Sry-related HMG box |
STAT | = | Signal transducers and activators of transcription |
STAT3 | = | Signal transducer and activator of transcription factor 3 |
TGF-β | = | Transforming growth factor-β |
TR | = | Thio-redoxin |
Disclosure statement
No potential conflict of interest was reported by the authors.
References
- Ferlay J, Héry C, Autier P, Sankaranarayanan R. Global Burden of Breast Cancer. Breast cancer epidemiology. New York, NY: Springer; 2010. p. 1–19.
- Sandhu GS, Erqou S, Patterson H, et al. Prevalence of triple-negative breast cancer in India: systematic review and meta-analysis. J Glob Oncol. 2016;2:412–421.
- Dontu G, Al‐Hajj M, Abdallah WM, et al. Stem cells in normal breast development and breast cancer. Cell Prolif. 2003;36:59–72.
- Wicha M, Dontu G, Al-Hajj M, et al. Stem cells in normal breast development and breast cancer. Breast Cancer Res. 2003;5:50.
- Chen K, Huang Y-H, Chen J-L. Understanding and targeting cancer stem cells: therapeutic implications and challenges. Acta Pharmacol Sin. 2013;34:732–740.
- Al-Hajj M, Wicha MS, Benito-Hernandez A, et al. Prospective identification of tumorigenic breast cancer cells. Proc Natl Acad Sci USA. 2003;100:3983–3988.
- Britton K, Eyre R, Harvey I, et al. Breast cancer, side population cells and ABCG2 expression. Cancer Lett. 2012;323:97–105.
- Reya T, Morrison SJ, Clarke MF, et al. Stem cells, cancer, and cancer stem cells. Nature. 2001;414:105–111.
- Li R-J, Ying X, Zhang Y, et al. All-trans retinoic acid stealth liposomes prevent the relapse of breast cancer arising from the cancer stem cells. J Control Release. 2011;149:281–291.
- Sabe H. Cancer early dissemination: cancerous epithelial-mesenchymal transdifferentiation and transforming growth factor β signalling. J Biochem. 2011;149:633–639.
- Hu C, Niestroj M, Yuan D, Chang S, Chen J. Treating cancer stem cells and cancer metastasis using glucose-coated gold nanoparticles. Int J Nanomed. 2015;10:2065.
- Karnoub AE, Dash AB, Vo AP, et al. Mesenchymal stem cells within tumour stroma promote breast cancer metastasis. Nature. 2007;449:557–563.
- Liu Z, Bandyopadhyay A, Nichols RW, et al. Blockade of autocrine TGF-β signaling inhibits stem cell phenotype, survival, and metastasis of murine breast cancer cells. J Stem Cell Res Ther. 2012;2:1.
- Chapellier M, Maguer-Satta V. BMP2, a key to uncover luminal breast cancer origin linked to pollutant effects on epithelial stem cells niche. Mol Cell Oncol. 2016;3:e1026527.
- Ablett MP, Singh JK, Clarke RB. Stem cells in breast tumours: are they ready for the clinic? Eur J Cancer. 2012;48:2104–2116.
- Borah A, Raveendran S, Rochani A, et al. Targeting self-renewal pathways in cancer stem cells: clinical implications for cancer therapy. Oncogenesis. 2015;4:e177.
- Vinogradov S, Wei X. Cancer stem cells and drug resistance: the potential of nanomedicine. Nanomedicine (Lond). 2012;7:597–615.
- Gao J, Feng S-S, Guo Y. Nanomedicine for treatment of cancer stem cells. Nanomedicine (Lond). 2014;9:181–184.
- Muntimadugu E, Kumar R, Saladi S, et al. CD44 targeted chemotherapy for co-eradication of breast cancer stem cells and cancer cells using polymeric nanoparticles of salinomycin and paclitaxel. Colloids Surf B Biointerfaces. 2016;143:532–546.
- Mamaeva V, Niemi R, Beck M, et al. Inhibiting notch activity in breast cancer stem cells by glucose functionalized nanoparticles carrying γ-secretase inhibitors. Mol Ther. 2016;24:926–936.
- Sun M, Yang C, Zheng J, et al. Enhanced efficacy of chemotherapy for breast cancer stem cells by simultaneous suppression of multidrug resistance and antiapoptotic cellular defense. Acta Biomater. 2015;28:171–182.
- Goodarzi N, Amini M, Ghahremani MH, et al. Hyaluronic acid-drug conjugate of docetaxel and metformin to target cancer cells and cancer stem cells: synthesis and characterization. J Control Release. 2013;1:e59.
- Zhou X, Chen L, Nie W, et al. Dual-responsive mesoporous silica nanoparticles mediated codelivery of doxorubicin and Bcl-2 SiRNA for targeted treatment of breast cancer. J Phys Chem C. 2016;120:22375–22387.
- Tummala S, Kumar MS, Pindiprolu SK. Improved anti-tumor activity of oxaliplatin by encapsulating in anti-DR5 targeted gold nanoparticles. Drug Deliv. 2016;23:3505–3519.
- Talluri SV, Kuppusamy G, Karri VVSR, et al. Application of quality-by-design approach to optimize diallyl disulfide-loaded solid lipid nanoparticles. Artif Cells Nanomed Biotechnol. 2017;45:474–488.
- Siddhartha VT, Pindiprolu SKSS, Chintamaneni PK, et al. RAGE receptor targeted bioconjuguate lipid nanoparticles of diallyl disulfide for improved apoptotic activity in triple negative breast cancer: in vitro studies. Artif Cells Nanomed Biotechnol. 2017;1–11. doi: 10.1080/21691401.2017.1313267. [Epub ahead of print]
- Talluri S, Pindiprolu S, Janarthanam R, Kuppusamy G. Development and efficacy evaluation of smart nanocarriers for targeting breast cancers. Eur J Cancer. 2016;57:S122.
- Bazak R, Houri M, El Achy S, et al. Cancer active targeting by nanoparticles: a comprehensive review of literature. J Cancer Res Clin Oncol. 2015;141:769–784.
- Shi F, Yang F, He X, et al. Inhibitory effect of epirubicin-loaded lipid microbubbles with conjugated anti-ABCG2 antibody combined with therapeutic ultrasound on multiple myeloma cancer stem cells. J Drug Target. 2016;24:34–46.
- Mattheolabakis G, Milane L, Singh A, et al. Hyaluronic acid targeting of CD44 for cancer therapy: from receptor biology to nanomedicine. J Drug Target. 2015;23:605–618.
- Wang D, Huang J, Wang X, et al. The eradication of breast cancer cells and stem cells by 8-hydroxyquinoline-loaded hyaluronan modified mesoporous silica nanoparticle-supported lipid bilayers containing docetaxel. Biomaterials. 2013;34:7662–7673.
- Goodarzi N, Ghahremani MH, Amini M, et al. CD44-targeted docetaxel conjugate for cancer cells and cancer stem-like cells: a novel hyaluronic acid-based drug delivery system. Chem Biol Drug Des. 2014;83:741–752.
- Rao W, Wang H, Han J, et al. Chitosan-decorated doxorubicin-encapsulated nanoparticle targets and eliminates tumor reinitiating cancer stem-like cells. ACS Nano. 2015;9:5725–5740.
- Swaminathan SK, Roger E, Toti U, et al. CD133-targeted paclitaxel delivery inhibits local tumor recurrence in a mouse model of breast cancer. J Control Release. 2013;171:280–287.
- Mohammadi M, Salmasi Z, Hashemi M, et al. Single-walled carbon nanotubes functionalized with aptamer and piperazine–polyethylenimine derivative for targeted siRNA delivery into breast cancer cells. Int J Pharm. 2015;485:50–60.
- Alibolandi M, Ramezani M, Sadeghi F, et al. Epithelial cell adhesion molecule aptamer conjugated PEG-PLGA nanopolymersomes for targeted delivery of doxorubicin to human breast adenocarcinoma cell line in vitro. Int J Pharm. 2015;479:241–251.
- Subramanian N, Kanwar JR, kumar Athalya P, et al. EpCAM aptamer mediated cancer cell specific delivery of EpCAM siRNA using polymeric nanocomplex. J Biomed Sci. 2015;22:4.
- Clarke MF, Dick JE, Dirks PB, et al. Cancer stem cells—perspectives on current status and future directions: AACR Workshop on cancer stem cells. Cancer Res. 2006;66:9339–9344.
- Fleming HE, Janzen V, Celso CL, et al. Wnt signaling in the niche enforces hematopoietic stem cell quiescence and is necessary to preserve self-renewal in vivo. Cell Stem Cell. 2008;2:274–283.
- Choi H, Chun Y-S, Kim T-Y, et al. HIF-2alpha enhances beta-catenin/TCF-driven transcription by interacting with beta-catenin. Cancer Res. 2010;70:10101–10111.
- Huang J, Tao C, Yu Y, et al. Simultaneous targeting of differentiated breast cancer cells and breast cancer stem cells by combination of docetaxel-and sulforaphane-loaded self-assembled poly (D, L-lactide-co-glycolide)/hyaluronic acid block copolymer-based nanoparticles. J Biomed Nanotechnol. 2016;12:1463–1477.
- Zhao P, Dong S, Bhattacharyya J, et al. iTEP nanoparticle-delivered salinomycin displays an enhanced toxicity to cancer stem cells in orthotopic breast tumors. Mol Pharm. 2014;11:2703–2712.
- Zhao P, Xia G, Dong S, et al. An iTEP-salinomycin nanoparticle that specifically and effectively inhibits metastases of 4T1 orthotopic breast tumors. Biomaterials. 2016;93:1–9.
- Artavanis-Tsakonas S, Rand MD, Lake RJ. Notch signaling: cell fate control and signal integration in development. Science. 1999;284:770–776.
- Androutsellis-Theotokis A, Leker RR, Soldner F, et al. Notch signalling regulates stem cell numbers in vitro and in vivo. Nature. 2006;442:823–826.
- Schweisguth F. Regulation of notch signaling activity. Curr Biol. 2004;14:R129–R138.
- Yang H, Li Y, Li T, et al. Multifunctional core/shell nanoparticles cross-linked polyetherimide-folic acid as efficient Notch-1 siRNA carrier for targeted killing of breast cancer. Sci Rep. 2014;4:7072.
- Moses H, Barcellos-Hoff MH. TGF-beta biology in mammary development and breast cancer. Cold Spring Harb Perspect Biol. 2011;3:a003277.
- Zuo Z-Q, Chen K-G, Yu X-Y, et al. Promoting tumor penetration of nanoparticles for cancer stem cell therapy by TGF-β signaling pathway inhibition. Biomaterials. 2016;82:48–59.
- Liu Y, Chen C, Qian P, et al. Gd-metallofullerenol nanomaterial as non-toxic breast cancer stem cell-specific inhibitor. Nat Commun. 2015;6:5988.
- Svärd J, Henricson KH, Persson-Lek M, et al. Genetic elimination of Suppressor of fused reveals an essential repressor function in the mammalian Hedgehog signaling pathway. Dev Cell. 2006;10:187–197.
- Hu K, Zhou H, Liu Y, et al. Hyaluronic acid functional amphipathic and redox-responsive polymer particles for the co-delivery of doxorubicin and cyclopamine to eradicate breast cancer cells and cancer stem cells. Nanoscale. 2015;7:8607–8618.
- You J, Zhao J, Wen X, et al. Chemoradiation therapy using cyclopamine-loaded liquid-lipid nanoparticles and lutetium-177-labeled core-crosslinked polymeric micelles. J Control Release. 2015;202:40–48.
- Ihle JN. The Stat family in cytokine signaling. Curr Opin Cell Biol. 2001;13:211–217.
- Ihle JN, Kerr IM. Jaks and Stats in signaling by the cytokine receptor superfamily. Trends Genet. 1995;11:69–74.
- Ihle JN, Witthuhn BA, Quelle FW, et al. Signaling by the cytokine receptor superfamily: JAKs and STATs. Trends Biochem Sci. 1994;19:222–227.
- Stahl N, Farruggella TJ, Boulton TG, et al. Choice of STATs and other substrates specified by modular tyrosine-based motifs in cytokine receptors. Science. 1995;267:1349.
- Heim MH, Kerr IM, Stark GR, et al. Contribution of STAT SH2 groups to specific interferon signaling by the Jak-STAT pathway. Science. 1995;267:1347
- Chung SS, Vadgama JV. Curcumin and epigallocatechin gallate inhibit the cancer stem cell phenotype via down-regulation of STAT3-NFκB signaling. Anticancer Res. 2015;35:39–46.
- Kim S-Y, Kang JW, Song X, et al. Role of the IL-6-JAK1-STAT3-Oct-4 pathway in the conversion of non-stem cancer cells into cancer stem-like cells. Cell Signal. 2013;25:961–969.
- Misra SK, Jensen TW, Pan D. Enriched inhibition of cancer and stem-like cancer cells via STAT-3 modulating niclocelles. Nanoscale. 2015;7:7127–7132.
- Brown JM, Attardi LD. The role of apoptosis in cancer development and treatment response. Nat Rev Cancer. 2005;5:231–237.
- Martinou J-C, Youle RJ. Mitochondria in apoptosis: Bcl-2 family members and mitochondrial dynamics. Dev Cell. 2011;21:92–101.
- Chen AM, Zhang M, Wei D, et al. Co‐delivery of doxorubicin and Bcl‐2 siRNA by mesoporous silica nanoparticles enhances the efficacy of chemotherapy in multidrug‐resistant cancer cells. Small. 2009;5:2673–2677.
- Li S-Y, Sun R, Wang H-X, et al. Combination therapy with epigenetic-targeted and chemotherapeutic drugs delivered by nanoparticles to enhance the chemotherapy response and overcome resistance by breast cancer stem cells. J Control Release. 2015;205:7–14.
- Shen S, Du X-J, Liu J, et al. Delivery of bortezomib with nanoparticles for basal-like triple-negative breast cancer therapy. J Control Release. 2015;208:14–24.
- Liu Y, Lu W-L, Guo J, et al. A potential target associated with both cancer and cancer stem cells: a combination therapy for eradication of breast cancer using vinorelbine stealthy liposomes plus parthenolide stealthy liposomes. J Control Release. 2008;129:18–25.
- Zhang L, Yao H-J, Yu Y, et al. Mitochondrial targeting liposomes incorporating daunorubicin and quinacrine for treatment of relapsed breast cancer arising from cancer stem cells. Biomaterials. 2012;33:565–582.
- Sadhukha T, Niu L, Wiedmann TS, et al. Effective elimination of cancer stem cells by magnetic hyperthermia. Mol Pharm. 2013;10:1432–1441.
- Shervington A, Lu C. Expression of multidrug resistance genes in normal and cancer stem cells. Cancer Invest. 2008;26:535–542.
- Yin L, Castagnino P, Assoian RK. ABCG2 expression and side population abundance regulated by a transforming growth factor beta-directed epithelial-mesenchymal transition. Cancer Res. 2008;68:800–807.
- Rabindran SK, He H, Singh M, et al. Reversal of a novel multidrug resistance mechanism in human colon carcinoma cells by fumitremorgin C. Cancer Res. 1998;58:5850–5858.
- Rabindran SK, Ross DD, Doyle LA, et al. Fumitremorgin C reverses multidrug resistance in cells transfected with the breast cancer resistance protein. Cancer Res. 2000;60:47–50.
- Woehlecke H, Osada H, Herrmann A, et al. Reversal of breast cancer resistance protein-mediated drug resistance by tryprostatin A. Int J Cancer. 2003;107:721–728.
- Zhao S, Smith KS, Deveau AM, et al. Biological activity of the tryprostatins and their diastereomers on human carcinoma cell lines. J Med Chem. 2002;45:1559–1562.
- Robey RW, Steadman K, Polgar O, et al. Pheophorbide a is a specific probe for ABCG2 function and inhibition. Cancer Res. 2004;64:1242–1246.
- Batrakova EV, Li S, Brynskikh AM, et al. Effects of pluronic and doxorubicin on drug uptake, cellular metabolism, apoptosis and tumor inhibition in animal models of MDR cancers. J Control Release. 2010;143:290–301.
- Batrakova EV, Li S, Li Y, et al. Effect of pluronic P85 on ATPase activity of drug efflux transporters. Pharm Res. 2004;21:2226–2233.
- Danson S, Ferry D, Alakhov V, et al. Phase I dose escalation and pharmacokinetic study of pluronic polymer-bound doxorubicin (SP1049C) in patients with advanced cancer. Br J Cancer. 2004;90:2085–2091.
- Minko T, Batrakova EV, Li S, et al. Pluronic block copolymers alter apoptotic signal transduction of doxorubicin in drug-resistant cancer cells. J Control Release. 2005;105:269–278.
- Venne A, Li S, Mandeville R, et al. Hypersensitizing effect of pluronic L61 on cytotoxic activity, transport, and subcellular distribution of doxorubicin in multiple drug-resistant cells. Cancer Res. 1996;56:3626–3629.
- Wang H, Agarwal P, Zhao S, et al. Hyaluronic acid-decorated dual responsive nanoparticles of Pluronic F127, PLGA, and chitosan for targeted co-delivery of doxorubicin and irinotecan to eliminate cancer stem-like cells. Biomaterials. 2015;72:74–89.
- Misra R, Das M, Sahoo BS, et al. Reversal of multidrug resistance in vitro by co-delivery of MDR1 targeting siRNA and doxorubicin using a novel cationic poly(lactide-co-glycolide) nanoformulation. Int J Pharm. 2014;475:372–384.
- Dong C, Yuan T, Wu Y, et al. Loss of FBP1 by Snail-mediated repression provides metabolic advantages in basal-like breast cancer. Cancer Cell. 2013;23:316–331.
- Ginestier C, Monville F, Wicinski J, et al. Mevalonate metabolism regulates Basal breast cancer stem cells and is a potential therapeutic target. Stem Cells. 2012;30:1327–1337.
- Patra KC, Wang Q, Bhaskar PT, et al. Hexokinase 2 is required for tumor initiation and maintenance and its systemic deletion is therapeutic in mouse models of cancer. Cancer Cell. 2013;24:213–228.
- Salani B, Del Rio A, Marini C, et al. Metformin, cancer and glucose metabolism. Endocr Relat Cancer. 2014;21:R461–R471.
- Xu Q, Zhu T, Yi C, et al. Characterization and evaluation of metformin-loaded solid lipid nanoparticles for cellular and mitochondrial uptake. Drug Dev Ind Pharm. 2016;42:701–706.
- Jain K, Paranandi KS, Sridharan S, et al. Autophagy in breast cancer and its implications for therapy. Am J Cancer Res. 2013;3:251–265.
- Mai TT, Moon J, Song Y, et al. Ginsenoside F2 induces apoptosis accompanied by protective autophagy in breast cancer stem cells. Cancer Lett. 2012;321:144–153.
- Singh BN, Kumar D, Shankar S, et al. Rottlerin induces autophagy which leads to apoptotic cell death through inhibition of PI3K/Akt/mTOR pathway in human pancreatic cancer stem cells. Biochem Pharmacol. 2012;84:1154–1163.
- Sun R, Shen S, Zhang Y-J, et al. Nanoparticle-facilitated autophagy inhibition promotes the efficacy of chemotherapeutics against breast cancer stem cells. Biomaterials. 2016;103:44–55.
- Bozorgi A, Khazaei M, Khazaei MR. New findings on breast cancer stem cells: a review. J Breast Cancer. 2015;18:303–312.
- He L, Gu J, Lim LY, et al. Nanomedicine-mediated therapies to target breast cancer stem cells. Front Pharmacol. 2016;7:313.
- Culig Z. Cytokine disbalance in common human cancers. Biochim Biophys Acta. 2011;1813:308–314.
- Korkaya H, Kim G-i, Davis A, et al. Activation of an IL6 inflammatory loop mediates trastuzumab resistance in HER2+ breast cancer by expanding the cancer stem cell population. Mol Cell. 2012;47:570–584.
- Kanapathipillai M, Brock A, Ingber DE. Nanoparticle targeting of anti-cancer drugs that alter intracellular signaling or influence the tumor microenvironment. Adv Drug Deliv Rev. 2014;79:107–118.
- Kanapathipillai M, Mammoto A, Mammoto T, et al. Inhibition of mammary tumor growth using lysyl oxidase-targeting nanoparticles to modify extracellular matrix. Nano Lett. 2012;12:3213–3217.
- Sabel MS, Skitzki J, Stoolman L, et al. Intratumoral IL-12 and TNF-α-loaded microspheres lead to regression of breast cancer and systemic antitumor immunity. Ann Surg Oncol. 2004;11:147–156.
- Knop K, Hoogenboom R, Fischer D, et al. Poly (ethylene glycol) in drug delivery: pros and cons as well as potential alternatives. Angew Chem Int Ed. 2010;49:6288–6308.
- Maeda H, Kabanov A, Kataoka K, Okano T. Polymer drugs in the clinical stage: advantages and prospects. New York: Springer; 2006.
- Mishra S, Webster P, Davis ME. PEGylation significantly affects cellular uptake and intracellular trafficking of non-viral gene delivery particles. Eur J Cell Biol. 2004;83:97–111.
- Mura S, Nicolas J, Couvreur P. Stimuli-responsive nanocarriers for drug delivery. Nat Mater. 2013;12:991
- Kale AA, Torchilin VP. Environment-responsive multifunctional liposomes. In: Weissig V, editor. Liposomes: methods and protocols, volume 1: pharmaceutical nanocarriers. New York: Springer; 2010; p. 213–242.
- Byrne JD, Betancourt T, Brannon-Peppas L. Active targeting schemes for nanoparticle systems in cancer therapeutics. Adv Drug Deliv Rev. 2008;60:1615–1626.
- Kaur S, Mehra NK, Jain K, et al. Development and evaluation of targeting ligand-anchored CNTs as prospective targeted drug delivery system. Artif Cells Nanomedicine Biotechnol. 2017;45:242–250.
- Xia P. Surface markers of cancer stem cells in solid tumors. Curr Stem Cell Res Ther. 2014;9:102–111.
- Hamad I, Hunter A, Szebeni J, et al. Poly (ethylene glycol) s generate complement activation products in human serum through increased alternative pathway turnover and a MASP-2-dependent process. Mol Immunol. 2008;46:225–232.
- Jackson AL, Linsley PS. Recognizing and avoiding siRNA off-target effects for target identification and therapeutic application. Nat Rev Drug Discov. 2010;9:57.
- Deng X, Wu X, Weng H, Song F. The siRNA-mediated silencing of Bmi-1 promotes apoptosis and inhibits invasion of MCF-7 breast cancer cells. Xi Bao Yu Fen Zi Mian Yi Xue Za Zhi [Chin J Cell Mol Immunol.]. 2016;32:1036.
- Zakerzadeh E, Alizadeh E, Samadi Kafil H, et al. Novel antibacterial polymeric nanocomposite for smart co-delivery of anticancer drugs. Artif Cells Nanomed Biotechnol. 2016;1–12. doi: 10.1080/21691401.2016.1260576. [Epub ahead of print]
- Yu M, Han S, Kou Z, et al. Lipid nanoparticle-based co-delivery of epirubicin and BCL-2 siRNA for enhanced intracellular drug release and reversing multidrug resistance. Artif Cells Nanomed Biotechnol. 2017;1–10. doi: 10.1080/21691401.2017.1307215. [Epub ahead of print]
- Godsey ME, Suryaprakash S, Leong KW. Materials innovation for co-delivery of diverse therapeutic cargos. RSC Adv. 2013;3:24794–24811.
- Wright MH, Calcagno AM, Salcido CD, et al. Brca1 breast tumors contain distinct CD44+/CD24-and CD133+ cells with cancer stem cell characteristics. Breast Cancer Res. 2008;10:R10.
- Klonisch T, Wiechec E, Hombach-Klonisch S, et al. Cancer stem cell markers in common cancers–therapeutic implications. Trends Mol Med. 2008;14:450–460.
- Bostad M, Olsen CE, Peng Q, et al. Light-controlled endosomal escape of the novel CD133-targeting immunotoxin AC133-saporin by photochemical internalization − a minimally invasive cancer stem cell-targeting strategy. J Control Release. 2015;206:37–48.
- Osta WA, Chen Y, Mikhitarian K, et al. EpCAM is overexpressed in breast cancer and is a potential target for breast cancer gene therapy. Cancer Res. 2004;64:5818–5824.
- Gilboa-Geffen A, Hamar P, Le MT, et al. Gene knockdown by EpCAM aptamer–siRNA chimeras suppresses epithelial breast cancers and their tumor-initiating cells. Mol Cancer Ther. 2015;14:2279–2291.
- Gurney A, Axelrod F, Bond CJ, et al. Wnt pathway inhibition via the targeting of Frizzled receptors results in decreased growth and tumorigenicity of human tumors. Proc Natl Acad Sci. 2012;109:11717–11722.
- Jang G-B, Hong I-S, Kim R-J, et al. WNT/β-Catenin small-molecule inhibitor CWP232228 preferentially inhibits the growth of breast cancer stem-like cells. Cancer Res. 2015;75:1691–1702.
- Takebe N, Harris PJ, Warren RQ, et al. Targeting cancer stem cells by inhibiting Wnt, Notch, and Hedgehog pathways. Nat Rev Clin Oncol. 2011;8:97–106.
- Chen B, Dodge ME, Tang W, et al. Small molecule–mediated disruption of Wnt-dependent signaling in tissue regeneration and cancer. Nat Chem Biol. 2009;5:100–107.
- Grudzien P, Lo S, Albain KS, et al. Inhibition of Notch signaling reduces the stem-like population of breast cancer cells and prevents mammosphere formation. Anticancer Res. 2010;30:3853–3867.
- Epenetos A, Kousparou C, Stylianou S. Inhibition of Notch signaling for the treatment of human carcinomas. AACR Annual Meeting, April 18–22, 2009; Denver, CO.
- Hoey T, Yen W-C, Axelrod F, et al. DLL4 blockade inhibits tumor growth and reduces tumor-initiating cell frequency. Cell Stem Cell. 2009;5:168–177.
- Noguera-Troise I, Daly C, Papadopoulos NJ, et al. Blockade of Dll4 inhibits tumour growth by promoting non-productive angiogenesis. Nature. 2006;444:1032–1037.
- Ridgway J, Zhang G, Wu Y, et al. Inhibition of Dll4 signalling inhibits tumour growth by deregulating angiogenesis. Nature. 2006;444:1083–1087.
- Scehnet JS, Jiang W, Kumar SR, et al. Inhibition of Dll4-mediated signaling induces proliferation of immature vessels and results in poor tissue perfusion. Blood. 2007;109:4753–4760.
- Liu M, Zhang W, Tang W, et al. Isocyclopamine, a novel synthetic derivative of cyclopamine, reverts doxorubicin resistance in MCF-7/ADR cells by increasing intracellular doxorubicin accumulation and downregulating breast cancer stem-like cells. Tumor Biol. 2016;37:1919–1931.
- Benvenuto M, Masuelli L, De Smaele E, et al. In vitro and in vivo inhibition of breast cancer cell growth by targeting the Hedgehog/GLI pathway with SMO (GDC-0449) or GLI (GANT-61) inhibitors. Oncotarget. 2016;7:9250.
- Thakur R, Trivedi R, Rastogi N, et al. Inhibition of STAT3, FAK and Src mediated signaling reduces cancer stem cell load, tumorigenic potential and metastasis in breast cancer. Sci Rep. 2015;5:10194.
- Korkaya H, Paulson A, Charafe-Jauffret E, et al. Regulation of mammary stem/progenitor cells by PTEN/Akt/beta-catenin signaling. PLoS Biol. 2009;7:e1000121.
- Lai Y, Yu X, Lin X, et al. Inhibition of mTOR sensitizes breast cancer stem cells to radiation-induced repression of self-renewal through the regulation of MnSOD and Akt. Int J Mol Med. 2016;37:369–377.
- Lima RT, Martins LM, Guimaraes JE, et al. Specific downregulation of bcl-2 and xIAP by RNAi enhances the effects of chemotherapeutic agents in MCF-7 human breast cancer cells. Cancer Gene Ther. 2004;11:309–316.
- Pandey PR, Okuda H, Watabe M, et al. Resveratrol suppresses growth of cancer stem-like cells by inhibiting fatty acid synthase. Breast Cancer Res Treat. 2011;130:387–398.
- Londoño-Joshi AI, Oliver PG, Li Y, et al. Basal-like breast cancer stem cells are sensitive to anti-DR5 mediated cytotoxicity. Breast Cancer Res Treat. 2012;133:437–445.
- Gong C, Bauvy C, Tonelli G, et al. Beclin 1 and autophagy are required for the tumorigenicity of breast cancer stem-like/progenitor cells. Oncogene. 2013;32:2261–2272.
- Marini C, Salani B, Massollo M, et al. Direct inhibition of hexokinase activity by metformin at least partially impairs glucose metabolism and tumor growth in experimental breast cancer. Cell Cycle. 2013;12:3490–3499.
- Hirsch HA, Iliopoulos D, Tsichlis PN, et al. Metformin selectively targets cancer stem cells, and acts together with chemotherapy to block tumor growth and prolong remission. Cancer Res. 2009;69:7507–7511.
- Zhao F, Ming J, Zhou Y, et al. Inhibition of Glut1 by WZB117 sensitizes radioresistant breast cancer cells to irradiation. Cancer Chemother Pharmacol. 2016;77:963–972.
- Zhang H, Lu H, Xiang L, et al. HIF-1 regulates CD47 expression in breast cancer cells to promote evasion of phagocytosis and maintenance of cancer stem cells. Proc Natl Acad Sci USA. 2015;112:E6215–E6223.
- Samanta D, Gilkes DM, Chaturvedi P, et al. Hypoxia-inducible factors are required for chemotherapy resistance of breast cancer stem cells. Proc Natl Acad Sci USA. 2014;111:E5429–E5438.
- Lock F, McDonald P, Lou Y, et al. Targeting carbonic anhydrase IX depletes breast cancer stem cells within the hypoxic niche. Oncogene. 2013;32:5210–5219.
- Ginestier C, Liu S, Diebel ME, et al. CXCR1 blockade selectively targets human breast cancer stem cells in vitro and in xenografts. J Clin Invest. 2010;120:485–497.
- Yip N, Fombon I, Liu P, et al. Disulfiram modulated ROS–MAPK and NFκB pathways and targeted breast cancer cells with cancer stem cell-like properties. Br J Cancer. 2011;104:1564–1574.
- Hou Z-J, Luo X, Zhang W, et al. Flubendazole, FDA-approved anthelmintic, targets breast cancer stem-like cells. Oncotarget. 2015;6:6326.
- Vazquez-Martin A, Oliveras-Ferraros C, Del Barco S, et al. The anti-diabetic drug metformin suppresses self-renewal and proliferation of trastuzumab-resistant tumor-initiating breast cancer stem cells. Breast Cancer Res Treat. 2011;126:355–364.
- Pan J-X, Ding K, Wang C-Y. Niclosamide, an old antihelminthic agent, demonstrates antitumor activity by blocking multiple signaling pathways of cancer stem cells. Chin J Cancer. 2012;31:178.
- Zhang Y, Zhang H, Wang X, et al. The eradication of breast cancer and cancer stem cells using octreotide modified paclitaxel active targeting micelles and salinomycin passive targeting micelles. Biomaterials. 2012;33:679–691.
- Ke X-Y, Ng VWL, Gao S-J, et al. Co-delivery of thioridazine and doxorubicin using polymeric micelles for targeting both cancer cells and cancer stem cells. Biomaterials. 2014;35:1096–1108.
- Prud'homme GJ, Glinka Y, Toulina A, et al. Breast cancer stem-like cells are inhibited by a non-toxic aryl hydrocarbon receptor agonist. PLoS One. 2010;5:e13831.
- Chen D, Pamu S, Cui Q, et al. Novel epigallocatechin gallate (EGCG) analogs activate AMP-activated protein kinase pathway and target cancer stem cells. Bioorg Med Chem. 2012;20:3031–3037.
- Zhu Y, Zhang X, Liu Y, et al. Antitumor effect of the mTOR inhibitor everolimus in combination with trastuzumab on human breast cancer stem cells in vitro and in vivo. Tumor Biol. 2012;33:1349–1362.
- Li Y, Zhang T, Korkaya H, et al. Sulforaphane, a dietary component of broccoli/broccoli sprouts, inhibits breast cancer stem cells. Clin Cancer Res. 2010;16:2580–2590.
- Gener P, Gouveia LP, Sabat GR, et al. Fluorescent CSC models evidence that targeted nanomedicines improve treatment sensitivity of breast and colon cancer stem cells. Nanomedicine Nanotechnol Biol Med. 2015;11:1883–1892.
- Ren X, Liu L, Zhou Y, et al. Nanoparticle siRNA against BMI-1 with a polyethylenimine–laminarin conjugate for gene therapy in human breast cancer. Bioconj Chem. 2015;27:66–73.