Abstract
Biocompatibility and ecotoxicity concerns associated with chemically produced metallic nanoparticles have led to an increasing interest in the development of environmentally benign alternatives for nanoparticle synthesis using biological platforms. Herein, we report the utilization of an extract of seed-derived callus of Catharanthus roseus for the production of stable silver nanoparticles (Ag NPs). The bioreduction of silver ions was evident from UV–Vis spectroscopy results: the absorption maxima were observed at 425 nm, indicative of elemental silver. Transmission electron micrographs revealed that the Ag NPs were well-dispersed and predominantly spherical with particle sizes in the range of 2–15 nm. The synthesized Ag NPs exhibited colloidal stability in an aqueous dispersion for a period of 120 days, as indicated by UV–Vis absorbance spectra and zeta potential measurements. Fourier transform infrared spectroscopy revealed the possible utilization of hydroxyl groups and amides in the reduction of silver ions and surface stabilization of the Ag NPs, respectively. Notably, the synthesized Ag NPs showed considerable antibacterial action against Escherichia coli even after 8 weeks of storage under ambient conditions. Thus, cell extracts of cultured callus of Catharanthus roseus could be explored as an ecofriendly platform for the synthesis of stable and functional nanoparticles.
Introduction
Inorganic nanomaterials with structures that impart novel and significantly improved physicochemical and biological properties have elicited much interest over the past few decades [Citation1]. Among noble metal nanostructures, silver nanoparticles (Ag NPs) exhibit unique and tuneable surface plasmon properties, high surface to volume ratio and easy surface functionalization [Citation2]. These unique properties promote the wide application of Ag NPs in diverse areas, including antimicrobial development [Citation3], biomolecular detection [Citation4], targeted drug delivery [Citation5] and catalysis [Citation6]. Crucial for these applications is the development of a suitable synthesis protocol in which size, shape, dispersity and stability can be controlled. Several chemical and physical methods for the preparation and stabilization of metal nanoparticles have been reported; of these conventional methods, Ag NPs with uniform and small particle sizes are typically prepared through wet chemical reduction of a silver precursor by a reducing agent such as sodium borohydride, citrate and ascorbate [Citation7]. However, owing to ecotoxicity and biocompatibility concerns that may be associated with such reducing agents, increasingly efforts have focused on the development of clean, environmentally benign “green” and sustainable approaches for the synthesis of Ag NPs [Citation2,Citation8].
To this end, the biological synthesis of Ag NPs with bacteria [Citation9], algae [Citation2,Citation10], plants [Citation11] or fungi [Citation12,Citation13] has attracted considerable interest as it affords eco-friendly, nontoxic and cost-effective routes. Among the different biological systems, the use of extract from parts of plants is preferred for nanoparticle biosynthesis owing to easier downstream processing, as it does not require the maintenance of cell cultures, thereby facilitating the large-scale synthesis of nanoparticles [Citation11]. Despite these advantages, fluctuations of the biological constituents in field-grown plant extracts owing to geographical, environmental and seasonal variations, as well as control of the polydispersity of the resultant nanoparticles, remain as challenges [Citation14,Citation15]. The inhomogeneity in size and shape of plant-extract-based nanoparticles has been attributed to the involvement in the reduction of silver ions of a wide variety of biomolecules with varying reducing capacities [Citation15–17]. To avoid this inhomogeneity, Sintubin et al. [Citation16] suggested the use of biological molecules or cellular structures extracted from eukaryotic or prokaryotic cells, which should give rise to more monodisperse Ag NPs.
For the production of secondary metabolites that are used as agrochemicals, pharmaceuticals and biopesticides, plant cell and organ cultures combine the merits of a whole plant system with those of microbial and animal cell cultures [Citation18,Citation19]. Cultured plant cells, like microorganisms, are inexpensive to grow and can be maintained in simple synthetic media. Notably, unlike field-grown plants, the biological constituents and performance of cultured plant cells are not affected by climate, season or weather [Citation18]. Therefore, cultured plant cell extracts may prove to be reliable sources of biomolecules that facilitate the synthesis and stabilization of nanoparticles.
The Madagascar periwinkle (Catharanthus roseus), a tropical perennial plant, is a source of several important indole alkaloids with medicinal value [Citation20]. Owing to its pharmaceutical importance, it has been regarded as an important model for plant secondary metabolism studies [Citation21]. To the best of our knowledge, we herein for the first time describe a green route that uses seed-derived callus extract of C. roseus in the synthesis of stable, well-dispersed and functional Ag NPs. Various characterization techniques were employed to reveal the intrinsic physicochemical characteristics of the obtained Ag NPs. The colloidal stability of the synthesized Ag NPs in aqueous dispersions was systematically studied by measuring the changes in UV–Vis absorbance spectra and zeta potential. The potential for the application of the synthesized Ag NPs as efficient antimicrobial agents was evaluated using Escherichia coli (E. coli) as the model organism. In addition, the long-term antibacterial activity of the synthesized Ag NPs was assessed using the agar well diffusion assay.
Materials and methods
Plant materials and culture conditions
Mature seeds of C. roseus L. (G). Don obtained from the Cell Cultivation Engineering Laboratory, University of Tsukuba, Japan were used in this study for callus induction. Sterile distilled or Milli-Q water was used in all the experiments. All glassware were rinsed with Milli-Q water and air-dried before use. Twenty to 30 surface-disinfected seeds were inoculated in culture medium consisting of Murashige and Skoog (MS) inorganic salts [Citation22] supplemented (per litre) with 0.5 mg 2,4-dichlorophenoxy acetic acid (2,4-D), 0.1 mg kinetin, 30 g sucrose and 2 g tissue-culture-grade agar (Gelrite). The pH was adjusted to 6.2 before autoclaving at 121 °C for 15 min. Callus induction was carried out in 300 ml Erlenmeyer flasks containing 100 ml of the medium. Cultures were incubated at 25 °C under a 16 h photoperiod with cool white fluorescent tubes at an intensity of 50–100 µmol m−2 s−1 in a plant growth chamber. After 4 weeks of incubation, calluses that formed on seed explants were selected and subsequently sub-cultured at 3–4 week intervals to obtain a mass of calluses. Unless otherwise stated, all experiments for Ag NPs biosynthesis were performed with 4 week old callus with a wet weight of approximately 20 g.
Synthesis of silver nanoparticles
Thoroughly washed greenish-white callus induced from C. roseus seeds was suspended in 100 ml of Mill-Q water and homogenized (2 × 15 min cycles) in an Ace homogenizer (Nihon Seiki Kaisha, Tokyo, Japan). To ensure complete breakage of the cells, the resulting homogenates were sonicated (3 × 5 min cycles) at maximum output and duty cycle in a Branson Sonifier 150 (Branson Ultrasonics, Danbury, CT). The mixture was subsequently centrifuged at 10,000 rpm for 15 min, and the supernatant was filtered with Advantec qualitative filter paper [150 mm] (Toyo Roshi Kaisha, Tokyo, Japan) to remove cell debris, if any. The resulting filtrate was used as aqueous callus extract. The synthesis of Ag NPs was carried out by the addition of 25 ml of aqueous callus extract to an equal volume of 1 mM AgNO3 solution in a 100 ml Erlenmeyer flask. The reaction mixture was incubated at 25 °C for 96 h. The reduction of AgNO3 to Ag NPs was monitored visually at 2 h intervals by observing the changes in colour of the reaction mixture.
Characterization of nanoparticles
The bioreduction of silver ions to nanoparticles was periodically monitored by measuring the absorption maxima of the reaction mixture in the wavelength range of 300–700 nm in a JASCO V-550 UV–Vis spectrophotometer (JASCO, Hachioji, Japan). The synthesized Ag NPs were separated from the reaction mixture by centrifugation at 10,000 rpm for 30 min, and the resulting pellets were re-dispersed in Milli-Q water by sonication for 5 min. The average diameters and polydispersities of the Ag NPs synthesized at various time intervals were determined by dynamic light scattering (DLS) using Zetasizer Nano ZS (Malvern Instruments, Worcestershire, UK). Furthermore, the morphologies and the particle size distribution of the Ag NPs were characterized by transmission electron microscopy (TEM; H-7650, Hitachi, Tokyo, Japan) at 80 kV. In brief, the aqueous dispersion of Ag NPs was dropped onto carbon-coated 200 mesh copper grids and then air-dried for at least 2 h in a fume hood before TEM imaging. The acquired images were analysed for particle size distribution by measuring the diameter of approximately 100 Ag NPs using Image J software (National Institute of Health NIH, Bethesda, MD). X-ray diffraction (XRD) measurements of the lyophilized Ag NPs were recorded in the 2θ range from 20° to 90° in a Bruker AXS D8 Advance diffractometer (Bruker, Berlin, Germany) with Cu Kα radiation (λ = 1.5406 Å) operating at a voltage of 40 kV and a current of 30 mA. Fourier transform infrared spectroscopy (FTIR) of the synthesized Ag NPs was recorded in the range 400–4000 cm−1 at a resolution of 4 cm−1 in KBr pellets with a JASCO FT/IR-300.
Colloidal stability of nanoparticles
The long-term stability of the synthesized Ag NPs in aqueous dispersions was determined by UV–Vis spectroscopy and electrophoretic light scattering (ELS-6000, Otsuka, Japan). A 10-fold dilution of the stock Ag NPs aqueous dispersion was stored at ambient temperature in a 50 ml falcon tube covered with aluminium foil. Aliquots of the sample were withdrawn at 1 week intervals for a period of 120 days to measure changes in the UV–Vis spectra and zeta potential.
Antibacterial assay
The antibacterial activity of the synthesized Ag NPs was studied using an agar well diffusion method [Citation23]. The strain of E. coli ATCC25922 obtained from the Cell Cultivation Engineering Laboratory, University of Tsukuba, Japan was used as a model test strain Gram-negative bacterium. The strain was cultivated by streaking a small amount of the frozen glycerol stock on Mueller–Hinton agar (MHA) plates and incubating at 37 °C for 24 h. For the preparation of bacterial inoculum, a single colony was inoculated in 25 ml MH broth and incubated overnight (12 h) at 37 °C while shaken (200 rpm). This culture was diluted 50-fold in MH broth, and 0.1 ml was spread onto MHA plates. Thereafter, different volumes of the synthesized Ag NPs corresponding to various amounts were added to the wells with a diameter of 6 mm; before use in this experiment, the Ag NPs were stored for either 1 or 8 weeks. Control plates were made using wells containing only callus extract of C. roseus. All plates were incubated at 37 °C for 24 h in a bacteriological incubator. The occurrence of a clear zone around the well was considered positive for antibacterial activity. The zone of inhibition (ZOI) was measured by subtracting the well diameter from the total inhibition zone diameter. The mean and standard deviation recorded were based on three independent experiments.
Inhibition of colony formation
The inhibition of colony formation by the synthesized Ag NPs was determined with the liquid-to-plate-assay method described previously by Lee et al. [Citation24] with minor modifications. In a liquid-to-plate assay, the nanoparticle and bacteria interactions can be observed in water without any media effect. In brief, an overnight culture of E. coli (ATCC25922) prepared as described above was centrifuged for 5 min at 4500 rpm at 4 °C, and the pellet was washed twice using PBS buffer (NaCl 8 g/L, KCl 0.2 g/L, Na2HPO4 1.44 g/L, KH2PO4 0.24 g/L) and autoclaved Milli-Q water. The washed pellet was re-suspended in autoclaved Milli-Q water, and the optical density (OD) was adjusted to approximately 0.1 at 600 nm. Aliquots of the microbial suspension were mixed with serial concentrations of Ag NPs to a final volume of 2 ml in a Falcon multi-well plate (Corning, NY) and incubated for 3 h at 37 °C while shaken at 200 rpm using a deep-well maximizer bioshaker [M-BR-022UP] (Taitec, Koshigaya, Japan). In addition, wells containing a microbial suspension mixed with autoclaved Milli-Q water served as a water control. After nanoparticle treatment, samples from the plate were taken every hour, spread onto MHA plates, and incubated for 24 h at 37 °C; three MHA plates for each combination of exposure time and Ag NPs concentration were tested. Viable cell counts were assessed visually as the number of colonies per plate. To calculate the percent colony formation, the average number of colonies from the nanoparticle-treated microbial suspension was compared with the number on the water control.
Data analyses
All data analyses were performed using Origin 8.0 (OriginLab, Northampton, MA) and Microsoft Excel 2010 statistical software packages. Mean separations were compared using Tukey’s test with a significance level of 5%. Representative spectra and images of at least three independent experiments are presented.
Results
Green synthesis of silver nanoparticles
The solution containing seed-derived callus extract of C. roseus and 1 mM AgNO3 solution was initially colourless (). A visible colour change to pale yellow was noticed within 2 h of incubation at 25 °C (). Thereafter, the gradual change in the colour of the reaction mixture was monitored over a period of 72 h; the maximum change (reddish brown) was observed after 72 h. These changes in the colour of the reaction mixture suggest the possible formation of Ag NPs. The visual observations were corroborated by the UV–Vis absorption measurements of the reaction mixture at different time intervals (): the absorption of the reaction mixture peaked at 425 nm, which is characteristic of elemental silver. The peak heights increased in direct proportion to the incubation period ().
Transmission electron microscopy
A representative TEM micrograph showing the morphologies of the synthesized Ag NPs after 24 h of incubation is presented in : the nanoparticles that formed were predominantly spherical and were well-dispersed (). The diameters of most of the Ag NPs were in the range of 2–15 nm with only a small fraction of the nanoparticles with diameters >15 nm ().
X-ray diffraction
The XRD technique was employed to determine and confirm the phase and crystallographic structure of the Ag NPs synthesized with seed-derived callus extract of C. roseus: four intense diffraction peaks were apparent at 2θ values of 38.2°, 46.1°, 64.4° and 76.7°, corresponding to the 111, 200, 220 and 311 planes, respectively, of the face-centred cubic crystalline structure of metallic silver (). These diffraction peaks are consistent with the database files of the Joint Committee on Powder Diffraction Standards (JCPDS) file no. 04-0783. The peak corresponding to the 111 plane was more intense compared to the other planes, indicating that it was the predominant orientation ().
Fourier transform infrared spectroscopy
FTIR measurements were performed on the aqueous callus extract and synthesized Ag NPs to identify the functional groups in the aqueous callus extract that might be involved in the reduction of silver ions and capping of the synthesized Ag NPs (). The FTIR spectrum of the callus extract showed major absorbance bands at 3350, 2928, 1635, 1385 and 1057 cm−1. The broad band at 3350 cm−1 corresponds to the stretching vibrations of a hydroxyl (O–H) group. We assign the band at 2928 cm−1 to aliphatic C–H stretching vibrations. The peak at 1635 cm−1 is characteristic of the carbonyl (C=O) stretching vibration in the amide linkages of proteins. We attribute the bands at 1385 and 1057 cm−1 to O–H bending vibrations of polyols and C–O stretching vibration of alcohol groups, respectively. The characteristic absorbance bands of the biosynthesized Ag NPs were similar to those of the callus extract (). A shift in the absorbance band from 3350 to 3310 cm−1 with an increase in band intensity was observed, indicating the possible utilization of the hydroxyl groups for the reduction of silver ions to nanoparticles. Furthermore, we observed a notable shift in the absorbance peak from 1635 to 1648 cm−1. We attribute the absorbance band at 1541 cm−1 to the combination of C–N stretching vibrations and in-plane N–H deformation modes of the peptide group. Thus, it is reasonable to infer that the synthesized Ag NPs were capped by amides that are characteristic of plant proteins.
Colloidal stability of silver nanoparticles in aqueous dispersion
The stability of the synthesized Ag NPs in aqueous dispersion was evaluated based on changes in the absorption maxima of the surface plasmon resonance (SPR) band and zeta potential. No considerable changes in the measured SPR band were detected as the bands remained almost symmetrical within the period tested (Supplementary Figure S1). Furthermore, no significant shift in the surface plasmon absorption peak was observed, indicating that the biosynthesized Ag NPs did not aggregate during the period of storage under ambient conditions. Similar to the SPR band, the measured zeta potential values did not vary considerably within the time period studied. The zeta potential of the synthesized Ag NPs stored for 1, 2, 3 and 120 days were −38.88, −37.12, −37.71 and −38.55 mV, respectively. Accordingly, we consider the biosynthesized Ag NPs to be stable against aggregation in aqueous dispersions.
Antibacterial assay
In the long-term assay of antibacterial activity of the biosynthesized Ag NPs, clear zones around the wells were observed in plates loaded with various amounts of Ag NPs. On the control plates with aqueous callus extract only, clear zones were not observed. The size of the ZOI followed a dose–response relationship with the amount of the Ag NPs: ZOIs of approximately 9 and 5 mm were observed in plates loaded with 60 and 12 µg of Ag NPs, respectively (). The Ag NPs stored for 8 weeks were less effective than those stored only 1 week. After 8 weeks of storage, the antibacterial activity (in terms of ZOI) on plates loaded with 60 µg of Ag NPs decreased by 1.6-fold when compared to those stored for 1 week ().
Inhibition of colony formation by silver nanoparticles
The antibacterial activity of the biosynthesized Ag NPs was also tested based on colony formation in liquid-to-plate assays. The reduction in colony formation was dependent on the concentration of the Ag NPs as well as the duration of exposure to autoclaved Milli-Q water (). As the concentration of the Ag NPs increased, colony formation decreased. Exposure to Ag NPs reduced colony formation within 1 h at all concentrations tested. Exposure to 0.15 µg/ml Ag NPs reduced colony formation by 34.1%, 52.6% and 58.8% after 1, 2 and 3 h, respectively, when compared to the water control. Furthermore, at the maximum tested concentration of 0.6 µg/ml, a complete 100% inhibition of colony formation was observed (). Extending the exposure duration of E. coli cells to Ag NP concentrations >0.15 µg/ml from 2 to 3 h did not significantly reduce colony formation (p < .05) ().
Figure 6. Inhibition of colony formation of E. coli exposed to various concentrations of the synthesized Ag NPs after 1 week of storage under ambient conditions (mean ± SD, n = 3). Relative colony formation is expressed as a percentage of the average number of colonies from nanoparticle-treated microbial suspension compared with the number on the water control.
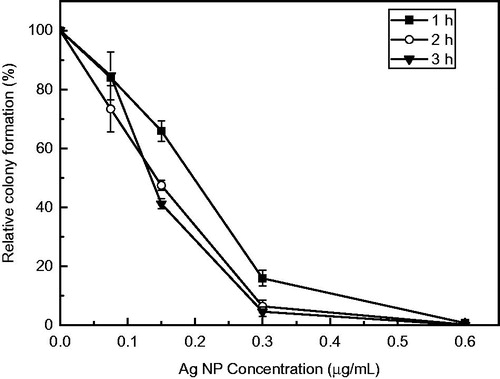
Discussion
The present study reports the production of stable Ag NPs through the interaction of aqueous silver ions from the AgNO3 and biomolecules present in an extract of seed-derived callus of C. roseus. The reduction of these silver ions to Ag NPs was evident from the change in colour of the reaction mixture from colourless to pale yellow within 2 h and to reddish brown within 24 h of incubation at 25 °C. Our visual observations were confirmed by UV–Vis absorption measurements of the reaction mixture: the absorption maximum was at 425 nm, characteristic of the SPR band of Ag NPs. The SPR patterns and characteristics of metal nanoparticles have been shown to be strongly dependent on particle size, stabilizing molecules, surface-adsorbed particles and the dielectric constant of the medium [Citation25]. The observed increase in peak heights as a function of reaction time may be caused by the increase in the efficiency of the synthesis of Ag NPs as a result of the strong reducing capacity of the seed-derived callus extract. Moreover, the absorption maximum did not shift after 24 h of reaction time, indicating the production of Ag NPs with similar particle sizes and shapes. Thus, according to Mie theory [Citation26], the synthesized Ag NPs were likely to be spherical in shape owing to the appearance of a single SPR. Martinez-Castanon et al. [Citation27] demonstrated that the width of the SPR band is related to the size distribution of the nanoparticles. In the present study, we observed that the broadness of the absorption maximum peak decreased with increasing reaction time, suggesting the formation of Ag NPs with a narrow size distribution. This suggestion was further confirmed by TEM.
Several studies have suggested that the physicochemical characteristics of nanoparticles, such as size, shape, solubility and surface charge, play significant roles in determining their biological activities [Citation28]. Hence, we used DLS and TEM to analyse the size and morphology of the synthesized Ag NPs. DLS analysis revealed that the average diameters of Ag NPs synthesized between 24 and 72 h did not differ (data not shown). Thus, since faster reaction times is desirable for efficient nanoparticle production, the morphologies and particle size distribution of Ag NPs synthesized after 24 h of incubation were further characterized using TEM. The TEM results indicate that most of the particles were roughly spherical with a fairly narrow distribution of diameter from 2 to 15 nm. Most of the Ag NPs were present as individual particles, with only a few aggregates. Thus, the TEM observations of the size distribution and shape of the synthesized Ag NPs are consistent with the UV–Vis spectroscopy results. Furthermore, the TEM micrograph shows that the Ag NPs were coated with a thin organic layer, which possibly acted as a capping agent; consequently, the nanoparticles were well dispersed in the aqueous reaction mixture (). Our XRD analysis confirms the crystalline structure of the synthesized Ag NPs. The observed broadening and strong signals of the XRD peaks were attributed to the nanocrystalline nature of the Ag NPs [Citation29]. Pal et al. [Citation1] proposed that the small size of nanoparticles and the presence of a 111 plane of the face centred cubic crystalline structure combine to promote their antimicrobial property. The XRD pattern of our synthesized Ag NPs indicate that the 111 plane was the predominant orientation.
The specific mechanism by which biomolecules present in biological extracts are involved in the synthesis of nanoparticles is yet to be fully elucidated [Citation10]. However, phytochemicals and plant-derived polysaccharides, such as cellulose, starch, dextran and alginic acid have been reported to play dual roles as reducing and capping agents in the plant-inspired synthesis of nanoparticles [Citation30]. In the current study, the FTIR analysis of the synthesized Ag NPs suggests the involvement of hydroxyl and carbonyl groups of amide linkages in proteins in the synthesis and stabilization of nanoparticles. This is evidenced by the prominent shift observed for the peaks corresponding to the O–H (3350–3310 cm−1) and C=O (1635–1648 cm−1) stretching vibrations in the callus extract after Ag NP synthesis. Park et al. [Citation31] reported that the oxidation of the hydroxyl group of polysaccharides to a carbonyl group is most likely involved in the reduction of metal ions to produce metallic nanoparticles. Similarly, in the synthesis of Ag nanoplates with Chlorella vulgaris extracts, Xie et al. [Citation32] demonstrated that the hydroxyl groups in Tyr residues and the carboxyl groups in Asp and/or Glu residues were responsible for the reduction of the Ag ion and the anisotropic growth of Ag nanocrystals into nanoplates, respectively. Proteins have been reported to provide a good protecting environment for metal hydrosols during their growth processes [Citation33]. Musarrat et al. [Citation34] reported that the carbonyl groups from amino acid residues and proteins can more strongly bind to metals, indicating that the proteins could possibly cap the Ag NPs to prevent agglomeration. Therefore, it is probable that in the present green synthesis, proteins in the callus extracts capped and stabilized the Ag NPs. Stabilization of the nanoparticles by the surface-bound proteins could explain the observed stability of the synthesized Ag NPs against aggregation in aqueous dispersion for the considerable time period of 120 days (see Supplementary Figure S1).
Silver-based compounds are known to exhibit a broad spectrum of antimicrobial activity against Gram-positive and Gram-negative bacteria, viruses and fungi [Citation35,Citation36]. There have been numerous reports on the antimicrobial potential of Ag NPs synthesized using biological platforms; however, the antimicrobial efficacy of plant-extract-based Ag NPs stored for several weeks under ambient conditions has not been investigated. The potential for the application of Ag NPs as an antimicrobial agent has been reported to be strongly dependent on their stability against aggregation [Citation37,Citation38]. The ZOI results presented in the current study are notable as the synthesized Ag NPs showed good antibacterial properties even after storage under ambient conditions for 8 weeks. The sustained antibacterial activity after storage for a reasonably long period of time may be due to the protein capping of the synthesized Ag NPs, which prevented them from aggregating. However, it is probable that the observed decrease in the antibacterial activity of the synthesized Ag NPs stored for 8 weeks compared to those stored for only 1 week may be due to the differential dissolution of Ag NPs during storage. Thus, further study is needed to reveal the rate and degree of dissolution of biosynthesized Ag NPs in both aqueous and biological media. Sivera et al. [Citation37] had earlier reported a decrease in the antimicrobial activity of gelatin-stabilized Ag NPs by a factor of approximately 2–4 after storage for 3 months. In the current study, the antibacterial potential of the synthesized Ag NPs was further validated by a liquid-to-plate-assay. A complete inhibition of colony formation was observed within 1 h of treatment of the bacterial cell suspension with 0.6 µg/ml Ag NPs (see ). Comparatively, at microbial density (OD600) of 0.002–0.003, Kim et al. [Citation39] reported a 100% inhibition of E. coli at a concentration of 0.25 mg/L for citrate-capped Ag NPs using the liquid-to-plate assay method. Thus, over a range of concentrations comparable to those of chemically synthesized Ag NPs, the biosynthesized Ag NPs showed bacterial toxicity. The results of the antibacterial assay in the present study hold significance as the biosynthesized Ag NPs maybe utilized as an environmentally benign alternative to the chemically synthesized counterparts especially in the disinfection of drinking water where lower inhibitory concentrations of antimicrobial agents is important.
The overall antibacterial potential is likely due to the intrinsic physicochemical characteristics of the synthesized Ag NPs, which mediate the controlled release of silver cations. Although the mode of action of Ag NPs as an antibacterial agent has not been fully elucidated, several researchers have implicated cell membrane damage and the disruption of the bacterial respiratory chain leading to the generation of reactive oxygen species [Citation2]. Mapara et al. [Citation40] reported the Ag-NP-induced turbulence of membranous permeability as an important contributing factor for bacterial growth inhibition. Furthermore, the inhibition of the enzymatic function of some proteins by interaction with the thiol groups of l-cysteine is also thought to be responsible for the antibacterial effect of Ag NPs [Citation41]. An earlier study by Li et al. [Citation42] reported the inactivation of respiratory chain dehydrogenases in E. coli cells exposed to Ag NPs, thus inhibiting respiration and growth of cells.
Conclusions
In summary, an environmentally benign, green synthesis method has been developed using seed-derived callus extracts of C. roseus for the preparation of Ag NPs. Our characterization studies reveal the formation of nanocrystalline silver with excellent stability in aqueous dispersions for a considerably long period of 120 days. As a result, the Ag NPs showed good potential as an antibacterial agent even when stored for 8 weeks under ambient conditions. As these bioinspired Ag NPs are stabilized by plant proteins, they could be integrated in various applications. Further studies are required on the roles of pH and biological solutions on the stability of the Ag NPs. Although we utilized E. coli as a model organism to assay the long-term antibacterial activity of the biosynthesized Ag NPs, investigations on the antimicrobial efficacy of these Ag NPs against Gram-positive bacteria and multi-resistant microbial strains are needed to further confirm their usefulness as stable broad spectrum antimicrobial agents.
Supplementary_material.pdf
Download PDF (66.2 KB)Acknowledgements
The authors thank the Japan Student Service Organisation for the fellowship awarded to DO.
Disclosure statement
The authors report that they have no conflicts of interest. The authors alone are responsible for the content and writing of the article.
Additional information
Funding
References
- Pal S, Tak YK, Song JM. Does the antibacterial activity of silver nanoparticles depend on the shape of the nanoparticle? A study of the gram-negative bacterium Escherichia coli. Appl Environ Microbiol. 2007;73:1712–1720.
- Aziz N, Faraz M, Pandey R, et al. Facile algae-derived route to biogenic silver nanoparticles: synthesis, antibacterial, and photocatalytic properties. Langmuir. 2015;31:11605–11612.
- Monteiro DB, Gorup LF, Takamiya AS, et al. The growing importance of materials that prevent microbial adhesion: antimicrobial effect of medical devices containing silver. Int J Antimicrob Agents. 2009;34:103–110.
- Wei H, Chen C, Han B, et al. Enzyme colorimetric assay using unmodified silver nanoparticles. Anal Chem. 2008;80:7051–7055.
- Duraipandy N, Lakra R, Vinjimur SK, et al. Caging of plumbagin on silver nanoparticles imparts selectivity and sensitivity to plumbagin for targeted cancer cell apoptosis. Metallomics. 2014;6:2025–2033.
- Christopher P, Xin H, Linic S. Visible-light-enhanced catalytic oxidation reactions on plasmonic silver nanostructures. Nat Chem. 2011;3:467–472.
- Panacek A, Kvitek L, Prucek R, et al. Silver colloid nanoparticles: synthesis, characterization, and their antibacterial activity. J Phys Chem B. 2006;110:16248–16253.
- Ramanathan R, O’Mullane AP, Parikh RY, et al. Bacterial kinetics-controlled shape-directed biosynthesis of silver nanoparticles using Morganella psychrotolerans. Langmuir. 2011;27:714–719.
- Singh R, Shedbalker UU, Wadhwani SA, et al. Bacteriagenic silver nanoparticles: synthesis, mechanism, and applications. Appl Microbiol Biotechnol. 2015;99:4579–4593.
- Singh G, Babele PK, Shahi SK, et al. Green synthesis of silver nanoparticles using cell extracts of Anabaena doliolum and screening of its antibacterial and antitumor activity. J Microbiol Biotechnol. 2014;24:1354–1367.
- Chung I, Park I, Seung-Hyun K, et al. Plant-mediated synthesis of silver nanoparticles: their characteristic properties and therapeutic applications. Nanoscale Res Lett. 2016;11:40.
- Fayaz AM, Balaji K, Girilal M, et al. Biogenic synthesis of silver nanoparticles and their synergistic effect with antibiotics: a study against gram-positive and gram-negative bacteria. Nanomedicine Nanotechnol Biol Med. 2010;6:103–109.
- Rodrigues AG, Ping LY, Marcato PD, et al. Biogenic antimicrobial silver nanoparticles produced by fungi. Appl Microbiol Biotechnol. 2013;97:775–782.
- Singh R, Wagh P, Wadhwani S, et al. Synthesis, optimization, and characterization of silver nanoparticles from Acinetobacter calcoaceticus and their enhanced antibacterial activity when combined with antibiotics. Int J Nanomedicine. 2013;8:4277–4290.
- Salunke GR, Ghosh S, Santosh Kumar RJ, et al. Rapid efficient synthesis and characterization of silver, gold, and bimetallic nanoparticles from the medicinal plant Plumbago zeylanica and their application in biofilm control. Int J Nanomedicine. 2014;9:2635–2653.
- Sintubin L, Verstraete W, Boon N. Biologically produced nanosilver: current state and future perspectives. Biotechnol Bioeng. 2012;109:2422–2436.
- Luo C, Shanmugam V, Yeh C. Nanoparticle biosynthesis using unicellular and subcellular supports. NPG Asia Mater. 2015;7:e209.
- Hellwig S, Drossard J, Twyman RM, et al. Plant cell cultures for the production of recombinant proteins. Nat Biotechnol. 2004;22:1415–1422.
- Murthy HN, Lee E, Paek K. Production of secondary metabolites from cell and organ cultures: strategies and approaches for biomass improvement and metabolite accumulation. Plant Cell Tiss Organ Cult. 2014;118:1–16.
- El-Sayed M, Verpoorte R. Catharanthus terpenoid indole alkaloids: biosynthesis and regulation. Phytochem Rev. 2007;6:277–305.
- Tonk D, Mujib A, Maqsood M, et al. Aspergillus flavus fungus elicitation improves vincristine and vinblastine yield by augmenting callus biomass growth in Catharanthus roseus. Plant Cell Tiss Organ Cult. 2016;126:291–303.
- Murashige T, Skoog F. A revised medium for rapid growth and bioassays with tobacco tissue cultures. Physiol Plant. 1962;15:473–497.
- Kora AJ, Manjusha R, Arunachalam J. Superior bacterial activity of SDS capped silver nanoparticles: synthesis and characterization. Mater Sci Eng C. 2009;29:2104–2109.
- Lee S, Lee J, Kim K, et al. Eco-toxicity of commercial silver nanopowders to bacterial and yeast strains. Biotechnol Bioproc E. 2009;14:490–495.
- Velmurugan P, Lee S, Iydroose M, et al. Pine cone-mediated green synthesis of silver nanoparticles and their antibacterial activity against agricultural pathogens. Appl Microbiol Biotechnol. 2013;97:361–368.
- Hussain S, Al-Thabaiti SA, Khan Z. Surfactant-assisted bio-conjugated synthesis of silver nanoparticles (AgNPs). Bioprocess Biosyst Eng. 2014;37:1727–1735.
- Martinez-Castanon GA, Nino-Martinez N, Martinez-Gutierrez F, et al. Synthesis and antibacterial activity of silver nanoparticles with different sizes. J Nanopart Res. 2008;10:1343–1348.
- Otari SV, Patil RM, Nadaf NH, et al. Green synthesis of silver nanoparticles by microorganisms using organic pollutant: its antimicrobial and catalytic application. Environ Sci Pollut Res. 2014;21:1503–1513.
- Oluwafemi OS, Lucwaba Y, Gura A, et al. A facile completely ‘green’ size tunable synthesis of maltose-reduced silver nanoparticles without the use of any accelerator. Colloids Surf B: Biointerfaces. 2013;102:718–723.
- Park Y. A new paradigm shift for the green synthesis of antibacterial silver nanoparticles utilizing plant extracts. Toxicol Res. 2014;30:169–178.
- Park Y, Hong YN, Weyers A, et al. Polysaccharides and phytochemicals: a natural reservoir for the green synthesis of gold and silver nanoparticles. IET Nanobiotechnol. 2011;5:69–78.
- Xie J, Lee JY, Wang DIC, et al. Silver nanoplates: from biological to biomimetic synthesis. ACS Nano. 2007;1:429–439.
- Mitra RN, Das PK. In situ preparation of gold nanoparticles of varying shape in molecular hydrogel of peptide amphiphiles. J Phys Chem C. 2008;112:8159–8166.
- Musarrat J, Dwivedi S, Singh BR, et al. Production of antimicrobial silver nanoparticles in water extracts of the fungus Amylomyces rouxii strain KSU-09. Bioresour Technol. 2010;101:8772–8776.
- Jo Y, Kim BH, Jung G. Antifungal activity of silver ions and nanoparticles on phytopathogenic fungi. Plant Dis. 2009;93:1037–1043.
- Elbeshehy EFK, Elazzazy AM, Aggelis G. Silver nanoparticles synthesis mediated by new isolates of Bacillus spp., nanoparticle characterization and their activity against bean yellow mosaic virus and human pathogens. Front Microbiol. 2015;6:453.
- Sivera M, Kvitek L, Soukupova J, et al. Silver nanoparticles modified by gelatin with extraordinary pH stability and long-term antibacterial activity. PLoS One. 2014;9:e103675.
- Ali K, Ahmed B, Dwivedi S, et al. Microwave accelerated green synthesis of stable silver nanoparticles with Eucalyptus globulus leaf extract and their antibacterial and antibiofilm activity on clinical isolates. PLoS One. 2015;10:e0131178.
- Kim SW, Baek Y, An Y. Assay-dependent effect of silver nanoparticles to Escherichia coli and Bacillus subtilis. Appl Microbiol Biotechnol. 2011;92:1045–1052.
- Mapara N, Sharma M, Shriram V, et al. Antimicrobial potentials of Helicteres isora silver nanoparticles against extensively drug-resistant (XDR) clinical isolates of Pseudomonas aeruginosa. Appl Microbiol Biotechnol. 2015;99:10655–10667.
- Bao H, Yu X, Xu C, et al. New toxicity mechanism of silver nanoparticles: promoting apoptosis and inhibiting proliferation. PLoS One. 2015;10:e0122535.
- Li W, Xie X, Shi Q, et al. Antibacterial activity and mechanism of silver nanoparticles on Escherichia coli. Appl Microbiol Biotechnol. 2010;85:1115–1122.