Abstract
Poly-L-lactic acid (PLLA) nano fibrous scaffolds prepared by electrospinning technology have been used widely in tissue engineering applications. However, PLLA scaffolds are hydrophobic in nature, moreover the fibrous porous structure produced by electrospinning makes the scaffolds even more hydrophobic which generally limits cell attachment and proliferation. Polymer blending is one of the several efforts used so far to enhance hydrophilicity and recognized as an easy cost-effective approach for the manipulation physiochemical properties of polymeric biomaterials. Pluronic block copolymers containing hydrophilic poly(ethylene oxide) (PEO) blocks and hydrophobic poly(propylene oxide) (PPO) blocks are arranged in triblock structure: PEO–PPO–PEO. It is commonly used recently to blend hydrophobic polymers to enhance hydrophilicity for pharmaceutical and tissue engineering applications. In this study, novel pluronic P123 blend PLLA electrospun nanofibre scaffolds with improved hydrophilicity and biological properties were fabricated. The surface morphology and surface chemistry of the nanofibre scaffolds were characterized by scanning electron microscope (SEM) and FTIR analyses. Surface hydrophilicity and change in mechanical properties were studied. The ability of the scaffolds to support the attachment, and proliferation and differentiation of human adipose tissue derived MSCs, were evaluated generally. The fabricated scaffolds have completely improved, hydrophilicity, similar osteogenic differentiation potential with plasma-treated PLLA nanofibre scaffold, and hence P123 blend PLLA electrospun nanofibre scaffolds are a very good and cost effective choice as a scaffold for bone tissue engineering application.
Introduction
Bone plays many vital roles in our physiology, some of them are: maintaining shape, facilitating movement, produces blood, mineral storage and homeostasis, regulates blood pH, mechanical support and protection of other vital soft tissues/organs [Citation1]. Its importance will be clearly visible in case of diseases where bone does not function properly such as: osteogenesis imperfecta, osteoarthritis, osteomyelitis and osteoporosis. These diseases together with traumatic injury, orthopaedic surgeries and primary tumour resection induce bone defects that demands bone repair or replacement [Citation2]. All these make bone defect one of the most common problems in orthopaedics [Citation3] with huge clinical and economic impacts [Citation2]. Nowadays, the number of patients suffering from such bone defects is rapidly increasing, with a huge need for bone substitutes (grafts) [Citation4].
Currently autologous bone grafting is commonly used as a gold standard for the treatment of critical-sized bone defects [Citation5]. But autograft have drawbacks like infection, scarring, blood loss [Citation3], donor site morbidity and secondary damage to the patient, plus the source is limited [Citation6]. Similarly, use of allograft or xenograft is associated with high risks of infectious diseases and rejection by the host immune system [Citation7]. The need for alternative approaches to develop reliable bone grafts reducing the risks and expenses of using autografts and allografts [Citation8] is increasing. Bone tissue engineering is an emerging technology which brings a solution for this problem [Citation7]. It uses the knowledge of life sciences and engineering to support the function of injured bone tissue via a triad of artificial extracellular matrix (ECM) scaffold, stem cells that can become osteoblasts and osteogenic agents [Citation9,Citation10]. Hence, scaffold, cells and growth factors are the three main components of bone tissue engineering [Citation11]. Development of a tissue engineering scaffold as an artificial bone matrix is crucial for regenerating bone tissue [Citation12]. It provides a three- dimensional environment for cell attachment and proliferation as well as filling bone defects while providing mechanical competence during bone regeneration [Citation13]. Hence, tissue engineering process begins with the fabrication of a biologically compatible scaffold that will support living cells to attach (adhere), proliferate and differentiate, and thus promote tissue regeneration both in vitro and in vivo [Citation12].
It is well known that biodegradable synthetic and/or natural polymers are widely used to fabricate tissue engineering scaffolds [Citation14]. Poly-L-lactic acid (PLLA) is a synthetic polymers most commonly used for scaffold fabrication in bone tissue engineering and has a great potential application yet to be exploited because of its special processability, biocompatibility [Citation14–16] and more importantly, it is possible to control its mechanical property and biodegradability by controlling its molecular weight through its synthesis conditions [Citation17]. PLLA nano fibrous scaffolds prepared by electrospinning technology have been used widely in tissue engineering applications; because of, its highly porous structure with interconnected pores similar to ECM [Citation18]. However, the scaffolds made from PLLA have some shortcomings; the surface properties are far from optimal. Principally, the low wettability and low surface energy remain an important issue [Citation19]. The nature of the scaffold surface mainly; chemical functionalities, charge, roughness and wettability are critical for cell adhesion and proliferation [Citation20,Citation21]. A poor cell adhesion and proliferation onto the surface can lead to a poor incorporation of the implant, infections, inflammations and even complete implant failure. Therefore, surface properties of PLLA need to be altered to enhance cell–material interactions [Citation22]. Water wetting is one of the basic parameters used to characterize the performance of scaffolds for tissue engineering [Citation23,Citation24].
The hydrophilic scaffolds provide an ideal substrate for homogeneous cell distribution, and fast cell growth; since, the appropriate absorption/diffusion of cell culture medium into the scaffolds and the existence of specific interaction sites for cellular attachment, all of which are crucial for the formation of well-integrated tissues [Citation25]. However, PLLA is hydrophobic in nature; moreover, the fibrous porous structure produced by electrospinning makes the scaffolds even more hydrophobic [Citation26]. It has been reported that the surface water contact angle (measure of hydrophilicity) of electrospun PLLA scaffolds is 128.2 ± 2.3 [Citation27], which limits its applications in tissue engineering, since cells may attach and proliferate not well on surfaces with water contact angle more than 90° [Citation28,Citation29]. Hence, it needs post-treatment in order to increase the hydrophilicity. So far several efforts were taken to enhance the hydrophilicity and physiological activities of hydrophobic scaffolds [Citation27,Citation30–35]. But, all these consumes time and cost. Another most important, time and cost saving approach is simultaneous use (blending) of hydrophilic, or amphiphilic polymers in the hydrophobic matrix [Citation32]. Blending is recognized as a cost-effective technique for the manipulation of material and physiochemical properties of polymeric biomaterials [Citation36].
Pluronic block copolymers are amphiphilic synthetic polymers containing hydrophilic poly(ethylene oxide) (PEO) blocks and hydrophobic poly(propylene oxide) (PPO) blocks arranged in triblock structure: PEO–PPO–PEO [Citation37–39]. Pluronic P123 is inexpensive commercially available, FDA approved, non-ionic surfactants that have been widely used for biomedical applications including drug delivery and tissue engineering. There are some reports that showed blending hydrophilic pluronics with hydrophobic polymers tends to segregate to the surface thereby modulating the surface properties of the hydrophobic polymer. Hence, pluronics with a relatively higher degree of hydrophilicity such as pluronic P123 would be more desirable. Moreover, there exist other examples in the literature where PEO–PPO–PEO polymers have been successfully used to modify the wettability of electrospun mats [Citation40,Citation41]. Pluronic has been successfully applied to improve the hydrophilicity of various hydrophobic polymers [Citation25,Citation42–44]. But, yet not used with PLLA for tissue engineering application.
Based on these facts, in this study we fabricated novel electrospun PLLA–P123 nanofibre scaffolds with improved hydrophilicity, biological properties, and having better osteogenic differentiation potential than either pristine PLLA or plasma treated PLLA nanofiber scaffolds for bone tissue engineering application. The surface morphology and surface chemistry of the nanofibre scaffolds were characterized by scanning electron microscope (SEM) and FTIR analyses. Surface wetting and change in mechanical properties were studied. The ability of the scaffolds to support the attachment, and proliferation and differentiation of human adipose tissue derived MSCs, were evaluated generally. As to our knowledge, no PLLA-P123 nanofibre scaffold has been fabricated before for bone tissue engineering applications.
Materials and methods
Fabrication of electrospun nanofibre scaffolds
PLLA and PLLA–P123 composite (PLLA–P123) scaffolds were prepared by electrospinning as follows. To make PLLA scaffolds first, 0.43 g of PLLA was dissolved in 6 ml of chloroform and continuously stirred under magnetic stirrer at 200 rpm till all the PLLA was completely dissolved. For PLLA–P123 scaffolds 0.043 g of P123 was added with PLLA at the same time. After that 1 ml of DMF was added and stirred until bubble free transparent solution was formed and then transferred to two 5 cc syringe with needle. One-step two nozzles electrospinning was used to fabricate the scaffolds. The electrospinning experimental setup was a Nano Model (Tehran, Iran) with two nozzles. The voltage applied at the tip of the needle was 18 kV. The mass flow rates was 0.5 ml/h, and distance between the tip of the needle and the collector was maintained at 15 cm. The speed of the rotary collector was 400 rpm and scanning distance was 10 cm. To compare effect of P123 on the hydrophilicity of the scaffolds one group of PLLA electrospun nano fibrous scaffolds were air plasma treated with Diener electronic plasma cleaner (Germany).
Characterization of the scaffolds
The morphology of the scaffold was examined under SEM. Pieces of scaffolds were placed on a surface and coated with gold using a sputter coater for 60 s at 18 mA prior to imaging with a JEOL JSM-6390LV SEM (JEOL Ltd., Japan), then the specimens were affected by electron irradiation and finally the SEM images were taken.
Fourier transformer infrared spectrophotometric analysis (FTIR)
The surface chemistry of the nanofibre scaffolds was analyzed via FTIR spectrophotometer (PerkinElmer Spectrum Version 10.4.1, USA).
Water contact angle
The hydrophobicity of the scaffolds was evaluated by sessile drop water contact angle measurement (OCA 15 plus) Surface Analysis system. A drop of water was placed on the surface of nanofibre scaffolds and the contact angel was measured after 10 s at room temperature.
Tensile testing
The mechanical strength of the nanofibre scaffolds was evaluated by a tensile testing machine (INSTRON-5566, Elancourt, France). A 5 mm by 30 mm pieces of the scaffolds was held on the surfaces by clips and placed in to the tensile tester. The tensile measurement was performed at 50 mm/min crosshead speed at room temperature.
Isolation and expansion of adipose derived mesenchymal stem cells
Isolation of mesenchymal stem cell from adipose tissue was performed according the previous protocol [Citation45,Citation46]. The adipose tissue was obtained from cosmetic liposuction of 10 different donors (age 24–45, Modarres Hospital, Tehran, Iran) with their full consent according the guidelines of Iran’s Ministry of Health Medical Ethics Committee. The adipose tissue was then treated with collagenase and trypsin (GIBCO) with shaking at 37 °C in an incubator for 30 min and then centrifuged, and the cell pellet was resuspended in Dulbecco’s Modified Eagle’s Medium (DMEM) containing 10% (v/v) fetal bovine serum (FBS), transferred to the flasks and put in to an incubator until reaching 80% confluence, the cell were subcultured till passage number 5.
Cell seeding and osteogenic differentiation
To seed cell, the scaffolds were cut in to 7.07 cm2 circular pieces, put in to 24-well tissue culture plate (TCP), sterilized with 70% ethanol, washed with PBS twice and finally, incubated with basal medium (DMEM supplemented with 10% FBS) including antibiotics. An initial density of 5 × 103 cells was seeded on each scaffolds for assessment of cell attachment and proliferation. For osteogenic differentiation evaluations, basal medium was supplemented with 100 nM dexamethasone, 0.2 mM ascorbic acid 2-phosphate and 10 mM β-glycerophosphate (Sigma-Aldrich, St. Louis, MO) and placed on the cell-loaded scaffolds after seeding an initial density of 104 cells followed by reaching 80% confluence.
Cell proliferation
The affinity of the cell for the new nanofibre scaffold which is defined with adhesion and proliferation of cultured stem cells on the scaffold was investigated through MTT assay. For this purpose, the incubated cell-loaded scaffolds were refreshed with DMEM including 10% MTT (Sigma-Aldrich, St. Louis, MO) solution after 24 h for cell adhesion and on days 1, 4 and 7 for cell proliferation assay and incubated again for 3–4 h in order to oxidize this substrate in the living cells by mitochondrial dehydrogenases. Then the supernatant was replaced with DMSO (Merck, Darmstadt, Germany) as the solvent of the dark blue formazan crystals formed in the live cells and finally the absorbance of purple solution was measured via spectrophotometer (Bioteck, Winooski, VT) at 570 nm.
Alkaline phosphatase activity (ALP)
ALP activity is an early marker of osteogenic differentiation of human MSCs. ALP activity was determined by a colorimetric method using Phosphatase Substrate Kit which contains P-Nitro Phenyl Phosphate (PNPP) as a substrate. Briefly, 250 ml RIPA buffer and protease inhibitor were used in order to extract the total protein of cells and prevent the extracted proteins from degradation. The lysate was shaken at 4 °C and centrifuged at 15,000 rpm for 15 min to sediment cell debris. Finally the supernatant was collected and the activity of ALP was measured by using an ALP assay kit (Parsazmoon, Tehran, Iran). The activity of enzyme (IU/L) was normalized against total protein (mg/dl).
Calcium content assay
To determine the extent of mineralization: first, the cell-loaded scaffolds were homogenized in 0.6 N HCl (Merck, Germany) and then were shaken at 4 °C for 1 h in order to extract the calcium deposited. Finally, the calcium content was calculated through a calcium content assay kit (Parsazmoon, Tehran, Iran) and cresolphtalein complexone interaction. Furthermore, the standard curve of OD versus concentration was estimated by using a serial dilution of standard solution in the kit.
Statistical analysis
For each measurement data were obtained at least in triplicate (n = 3), averaged and expressed as mean ± standard deviation (SD). Statistical analysis was performed using one way analysis of variance (ANOVA). A value of p ≤.05 was considered statistically significant.
Results and discussion
Characterization of the scaffolds
The morphology of all of the nanofibres (PPLA, PLLA–plasma and PLLA–P123) was smooth, bead free, uniform and continuous with interconnected pores between the nanofibres as shown in . However, there were a significant (p < .05) differences in the fibre diameter between PLLA–P123 and PLLA nanofibres. PLLA–P123 nanofibres with an average diameter 860.2 nm were much thicker than the PLLA nanofibres with 570 nm. The possible reason for this is that the incorporation of additives can change the solution properties [Citation47], in this case the conductivity of the electrospinning solutions decreased and the viscosity of the solution increases by the addition of P123. However, there was no difference in the surface morphology and fibre diameter of plasma treated and untreated nanofibres; since, plasma treatment has no significant effect on the surface morphology of electrospun nanofibres where the diameter of electrospun nanofibres is larger than 80 nm [Citation27].
Figure 1. Surface morphology of the nanofibre scaffolds at 3000× magnification: (A) PLLA, (B) PLLA–plasma and (C) PLLA–P123.
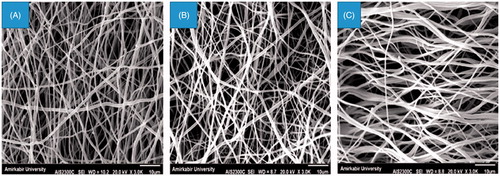
FTIR analysis is one of the best methods used to determine surface chemistry of a system containing two or more chemicals. In our work, it was done to confirm the presence of P123 incorporated in the electrospun nanofibres. FTIR spectrum analysis in showed strong peaks at 1759 cm−1, 1182 cm−1 and 1083 cm−1 for C = O, C–O of ester and acidic groups, respectively in PLLA nanofibres which show a little change in position in P123 blend scaffold. AS shown in the figure, the characteristic peaks of P123 in blend fibres were totally overlapped by PLLA peaks. Definitely, FTIR spectra of the PLLA and PLLA–P123 display similar patterns, indicating that no significant change in the molecular structure taken place upon blending as stated elsewhere [Citation25]. We have seen minimal differences between the FTIR spectra of pristine and PLLA–plasma scaffolds. Since plasma treatments only change the top layer (10 nm or less) of a material without interfering with the bulk properties: the treated surface layer is located on top of the bulk material, which is usually several orders of magnitude larger where FTIR will not be able to detect structural changes upon plasma surface treatments since the sampling depth of FTIR is usually too large [Citation48].
Mechanical properties of fabricated scaffolds
The mechanical properties of the scaffolds are very important parameter to characters nanofibre scaffolds used in tissue engineering because the scaffold need to withstand stress incurred during in vitro culture and in vivo implantation [Citation49]. The electrospun PLLA nanofibres scaffolds had a tensile strength of 1.92 MPa and a breaking tensile strain of 88%, which has been changed to 1.46 MPa and 105% after blending with P123. However, plasma-treated scaffolds did not have apparent effect on tensile of the nanofibre scaffolds. Hence, P123 blending of PLLA reduced the tensile strength but increased the extension of breaking of the electrospun fibres scaffolds.
Surface hydrophilicity
The water contact angel of PLLA nanofibre scaffolds was radically changed form 128.2 ± 2.3 to 0 degree by the incorporation of P123; whereas, plasma treatment changed it to 46.5 ± 1.8 degrees showing the effect of P123 blending to bring complete hydrophilicity compared with plasma treatment. The surface hydrophilicity of nanofibres plays key role in determining the overall performance for tissue engineering applications; because, it affects the cell attachment and proliferation to great extent. The water contact angle of the surface which depends up on the topographic configurations and chemical composition of the scaffolds is the parameter commonly used to test the hydrophilicity of the nanofibre scaffolds [Citation25,Citation32,Citation50]. Electrospun PLLA nanofibre scaffolds are hydrophobic; however, blending of P123 was successfully used to increase the hydrophilicity of electrospun scaffolds maintaining the mechanical flexibility which further affects cell adhesion and proliferation. Plasma effects are not permanent, and an aging takes place during the storage of polymer mats, because the polar groups will rearrange towards the bulk material during storage, the contact angle returns back to higher values [Citation20].
Polymer blending with an amphiphilic polymer such as the copolymers of poly(ethylene oxide)-b-poly(propylene oxide)-b-poly(ethylene oxide) (PEO − PPO − PEO), known as Poloxamers, or commercially as Pluronics is the best alternative for producing hydrophilic surfaces [Citation43,Citation51]. These polymers have hydrophobic PPO as the midblock and hydrophilic PEO as the end-blocks. The hydrophobic part will help to anchor the P123 molecule to the hydrophobic surface of PLLA and brush like PEO segments extend in contact with water [Citation19]. During electrospinning and until solvent evaporation, these molecules segregated to the surface and completely altered the wetting behaviour of the scaffolds [Citation32]. Once the surface is in contact with water, hydrophilic PEO segments will extend and enrich the surface [Citation44]. It is assumed that the increase in hydrophilicity in the nanofibre meshes could be as a result of the possible hydrophobic interaction between the –CH3 groups of PLLA and the –CH3 groups of P123 which restrict the integration of EO monomers into the PLLA polymer chains thereby projecting them towards the surface [Citation42]. These inter-chain interactions inhibit the incorporation of PEO blocks of P123 into the PLLA chains thus forcing them toward the surface. In other words, P123 triblock can be anchored to the hydrophobic surface of PLLA by PPO blocks and PEO blocks assemble into a brushlike formation in contact with water. [Citation25,Citation42,Citation44) So, The P123 is stable and will not dissolve because of its anchoring and locking in the PLLA chains through hydrophobic interactions. It will be stable as long as PLLA chains are stable.
Cell attachment and proliferation
The cell affinity which is defined as adhesion and proliferation of cultured stem cells was investigated through MTT assay. In this assay, metabolically active cells break down the tetrazolium salt in to a soluble formazan dye, which can absorb at a wave length of 490 nm in a UV–visible spectrophotometer [Citation27].
To assess the potential of PLLA–P123 as tissue-engineered scaffolds, and to investigate its effects on stem cell attachment and proliferation, cells were cultured on nanofibres and their proliferation, infiltration and osteogenic differentiation were investigated in vitro. The MTT result as shown in demonstrated that PLLA–P123 nanofibres are more conducive to cell duplication compared with pure PLLA mats. The cells were grown and were well spread on the nanofibres. An increasing pattern of cell population have been seen during the period of study, for all three scaffolds, that is Pristine PLLA, PLLA–plasma and PLLA–P123. Starting from day one till day 7 the proliferation of MSC in PLLA–P123 was comparable with that of PLLA–plasma scaffold but significantly greater than pristine PLLA scaffolds (p < .005). On day 1 and day 4 cells cultured on PLLA–P123 scaffolds showed almost similar proliferation rate to PLLA–plasma scaffolds but significantly higher than pristine PLLA scaffolds (p < .05). On day 7 the rate of proliferation between PLLA–P123 and PLLA–plasma scaffolds was different but not significant, however, both scaffold observed great significant proliferation rate of MSC than PLLA scaffolds (p < .05). The results revealed that cell proliferation and expansion were more noticeable on blended PLLA–P123 and PLLA–plasma nanofibres compared with the PLLA nanofibres. Hence, hydrophobicity and surface morphology of nanofibres are known to influence cellular adhesion and proliferation [Citation52]. Therefore, in this study, cell adhesion and proliferation on PLLA electrospun scaffolds were enhanced by blending with P123, which is consistent with the significant increase in hydrophilicity [Citation36].
Osteogenic differentiation of human adipose tissue derived mesenchymal stem cells
The ability of the nanofibre scaffolds (PLLA, PLLA–plasma and PLLA–P123) to support and facilitate osteogenic differentiation of MSC was evaluated by measuring the quantity of bone related markers such as alkaline phosphatase activity and calcium deposition (mineralization). ALP activity is an early marker of osteogenic differentiation of human MSCs. It increases but then decreases when the culture becomes well-mineralized. ALP activity of MSCs cultured in osteogenic conditions usually peaks after 14–21 days, depending on cell sources and culture conditions [Citation53]. ALP activity of adipose tissue-derived MSCs seeded on different nanofibre scaffolds and media is shown in . The figure depicted that an increase in ALP activity of cells cultured on the two nanofibre scaffolds (PLLA–plasma, PLLA–P123) in all the days (7, 14 and 21 days), the highest ALP activity was observed at day 14 in both scaffolds. On day 7 and 21 the ALP activity of cells cultured on PLLA–P123 nanofibres was greater than that of PLLA–plasma but was not significant (p < .05). In all the culture days the ALP activity of cells cultured on the two scaffolds was significantly greater than that of pristine PLLA scaffolds (p < .05). Moreover, the evaluation of calcium content demonstrated an increase in mineralization in all the three nanofibre scaffolds (PLLA, PLLA–plasma and PLLA–P123) in all the days tested as shown in . The highest calcium content was observed at day 21 by both PLLA–Plasma and PLLA–P123 nanofibre scaffold. The extent of mineralization was comparable between the two scaffolds but significantly greater than that of observed on pristine PLLA scaffolds (p < .05). Similar trend of mineralization was observed in all the three consecutive weeks tested. We have seen here that one of the major drawbacks that limits the biomedical application of PLLA is its poor hydrophilicity. Hence, polymer blending is an effective, simple, versatile and less costly method to develop new materials with modified properties without the need to make new polymers [Citation54].
Conclusion
P123 blend PLLA nanofibre scaffolds with very good cell adhesion, cell proliferation and generally with better osteogenic differentiation were successfully fabricated by electrospinning technology. The fabricated scaffolds have completely improved, hydrophilicity, comparable osteogenic differentiation potential with plasma-treated PLLA nanofibre scaffold, and hence P123 blend electrospun nanofibre scaffolds are a very good choice as a scaffold for bone tissue engineering application; since, using P123 is more and more cost effective, versatile and safe; in addition to improving the physical properties, especially the surface hydrophilicity and mechanical properties than plasma-treated PLLA nanofibre. However, further in vivo study is required to decide the ability of fabricated scaffolds for bone tissue regeneration in critical-size bone defects.
Acknowledgements
This work was supported by the Tehran University of Medical Sciences, International Campus (TUMS-IC), Tehran, Iran.
Disclosure statement
No potential conflict of interest was reported by the authors.
References
- Lee NK, Sowa H, Hinoi E, et al. Endocrine regulation of energy metabolism by the skeleton. Cell. 2007;130:456–469.
- Porter JR, Ruckh TT, Popat KC. Bone tissue engineering: a review in bone biomimetics and drug delivery strategies. Biotechnol Prog. 2009;25:1539–1560.
- Polo-corrales L, Latorre-esteves M, Ramirez-vick JE. Scaffold design for bone regeneration. J Nanosci Nanotechnol. 2014;14:15–56.
- Li J, Hong J, Zheng Q, et al. Repair of rat cranial bone defects with nHAC/PLLA and BMP-2-related peptide or rhBMP-2. J Orthop Res. 2011;29:1745–1752.
- Lane JM, Tomin E, Bostrom MPG. Biosynthetic bone grafting. Clin Orthop Relat Res. 1999;367:107–117.
- Oryan A, Alidadi S, Moshiri A, et al. Bone regenerative medicine: classic options, novel strategies, and future directions. J Orthop Surg Res. 2014;9:1–27.
- Qi H, Ye Z, Ren H, et al. Bioactivity assessment of PLLA/PCL/HAP electrospun nanofibrous scaffolds for bone tissue engineering. Life Sci. 2016;148:139–144.
- Burg KJ, Porter S, Kellam JF. Biomaterial developments for bone tissue engineering. Biomaterials. 2000;21:2347–2359.
- Khojasteh A, Behnia H, Dashti SG, et al. Current trends in mesenchymal stem cell application in bone augmentation: a review of the literature. J Oral Maxillofac Surg. 2012;70:972–982.
- Tabatabaei FS, Motamedian SR, Gholipour F, et al. Craniomaxillofacial bone engineering by scaffolds loaded with stem cells: a systematic review. J Den Sch. 2017;30:113–130.
- Motamedian SR, Hosseinpour S, Ahsaie MG, et al. Smart scaffolds in bone tissue engineering: a systematic review of literature. World J Stem Cells. 2015;7:657–668.
- Thavornyutikarn B, Chantarapanich N, Chen Q. Bone tissue engineering scaffolding: computer-aided scaffolding techniques. Prog Biomater. 2014;3:61–102.
- Fröhlich M, Grayson WL, Wan LQ, et al. Tissue engineered bone grafts: biological requirements, tissue culture and clinical relevance. Cscr. 2008;3:254–264.
- Lou T, Wang X, Song G. Fabrication of nano-fibrous poly(L-lactic acid) scaffold reinforced by surface modified chitosan micro-fiber. Int J Biol Macromol. 2013;61:353–358.
- Khajavi R, Abbasipour M, Bahador A. Electrospun biodegradable nanofibers scaffolds for bone tissue engineering. J Appl Polym Sci. 2016;133:42883 (1–19).
- Xie L, Yu H, Yang W, et al. Preparation, in vitro degradability, cytotoxicity, and in vivo biocompatibility of porous biocomposite scaffolds. J Biomat Sci Poly Edi. 2016;27:5063.
- Lu L, Peter SJ, Lyman MD, et al. In vitro degradation of porous poly (L-lactic acid) foams. Biomaterials. 2000;21:1595–1605.
- Kontogiannopoulos KN, Assimopoulou AN, Tsivintzelis I, et al. Electrospun fiber mats containing shikonin and derivatives with potential biomedical applications. Int J Pharm. 2011;409:216–228.
- Wang S, Cui W, Bei J. Bulk and surface modifications of polylactide. Anal Bioanal Chem. 2005;381:547–556.
- Akkas T, Citak C, Sirkecioglu A, et al. Which is more effective for protein adsorption: surface roughness, surface wettability or swelling? Case study of polyurethane films prepared from castor oil and poly (ethylene glycol). Polym Int. 2012;62:1202–1209.
- Chang H, Huang C, Lin K, et al. Effect of surface potential on NIH3T3 cell adhesion and proliferation. J Phys Chem C. 2014;118:14464–14470.
- Jacobs T, Declercq H, De Geyter N, et al. Plasma surface modification of polylactic acid to promote interaction with fibroblasts. J Mater Sci Mater Med. 2013;24:469–478.
- Wu J, Wang N, Zhao Y, et al. Electrospinning of multilevel structured functional micro-/nanofibers and their applications. J Mater Chem A1.1. 2013;25:7290–7305.
- Xue Z, Cao Y, Liu N, et al. Special wettable materials for oil/water separation. J Mater Chem A2. 2014;8:2445–2460.
- Mirhosseini MM, Zargarian SS. Fabrication and characterization of hydrophilic poly (e-caprolactone)/pluronic P123 electrospun fibers. J Appl Polym Sci. 2016;133:43345–43355.
- Cui W, Li X, Zhou S, et al. Degradation patterns and surface wettability of electrospun fibrous mats. Polym Degrad Stabil. 2008;93:731–738.
- Liu W, Zhan J, Su Y, et al. Effects of plasma treatment to nanofibers on initial cell adhesion and cell morphology. Colloids Surf B Biointerf. 2014;113:101–106.
- Ma BM, Gupta M, Li Z, et al. Decorated electrospun fibers exhibiting superhydrophobicity. Adv Mater. 2007;19:255–259.
- Cui W, Cheng L, Li H, et al. Preparation of hydrophilic poly (L-lactide) electrospun fibrous scaffolds modified with chitosan for enhanced cell biocompatibility. Polymer. 2012;53:2298–2305.
- Khatri Z, Wei K, Kim B, et al. Effect of deacetylation on wicking behavior of co-electrospun cellulose acetate/polyvinyl alcohol nanofibers blend. Carbohydr Polym. 2012;87:2183–2188.
- De Luca AC, Terenghi G, Downes S. Chemical surface modification of poly-e-caprolactone improves Schwann cell proliferation for peripheral nerve repair. Tissue Eng Regen Med. 2012;8:153–163.
- Kurusu RS, Demarquette NR. Wetting of hydrophilic electrospun mats produced by blending SEBS with PEO–PPO–PEO copolymers of different molecular weight. Langmuir. 2016;32:1846–1853.
- Zhang Y, Ouyang H, Lim CT, et al. Electrospinning of gelatin fibers and gelatin/PCL composite fibrous scaffolds. J Biomed Mater Res Part B Appl Biomater. 2004;72:156–165.
- Savoji H, Lerouge S, Ajji A, et al. Plasma-etching for controlled modification of structural and mechanical properties of electrospun PET scaffolds. Plasma Process Polym. 2015;12:314–327.
- De Valence S, Tille J, Chaabane C, et al. Plasma treatment for improving cell biocompatibility of a biodegradable polymer scaffold for vascular graft applications. Eur J Pharm Biopharm. 2013;85:78–86.
- Daranarong D, Chan RTH, Wanandy NS, et al. Electrospun polyhydroxybutyrate and poly (L-lactide-co-ε-caprolactone) composites as nanofibrous scaffolds. BioMed Research Int. 2014;2014:741408. doi: 10.1155/2014/741408.
- Zhang W, Shi Y, Chen Y, et al. Multifunctional pluronic P123/F127 mixed polymeric micelles loaded with paclitaxel for the treatment of multidrug resistant tumors. Biomaterials. 2011;32:2894–2906.
- Wei Z, Hao J, Yuan S, et al. Paclitaxel-loaded pluronic P123/F127 mixed polymeric micelles: Formulation, optimization and in vitro characterization. Int J Pharm. 2009;376:176–185.
- Liu Z, Liu D, Wang L, et al. Docetaxel-loaded pluronic P123 polymeric micelles: in vitro and in vivo evaluation. Int J Mol Sci. 2011;12:1684–1696.
- Hunt JA, Chen R, Van Veen T, et al. Hydrogels for tissue engineering and regenerative medicine. J Mater Chem B Mater Biol Med. 2014;2:5319–5338.
- Wang Y, Wang T, Su Y, et al. Remarkable reduction of irreversible fouling and improvement of the permeation properties of poly (ether sulfone) ultrafiltration membranes by blending with pluronic F127. Langmuir. 2005;21:11856–11862.
- Vasita R, Mani G, Agrawal CM, et al. Surface hydrophilization of electrospun PLGA micro-/nanofibers by blending. Polymer®F-108. Polymer. 2010;51:3706–3714.
- Liu N, Pan J, Miao YE, et al. Electrospinning of poly (e-caprolactone-co-lactide)/pluronic blended scaffolds for skin tissue engineering. J Mater Sci. 2014;49:7253–7262.
- Kurusu RS, Demarquette NR. Blending and morphology control to turn hydrophobic SEBS electrospun mats superhydrophilic. Langmuir. 2015;31:5495–5503.
- Amjadian S, Seyedjafari E, Zeynali B, et al. The synergistic effect of nano-hydroxyapatite and dexamethasone in the fibrous delivery system of gelatin and poly(l-lactide) on the osteogenesis of mesenchymal stem cells. Int J Pharm. 2016;507:1–11.
- Ramezanifard R, Seyedjafari E, Ardeshirylajimi A, et al. Biomimetic scaffolds containing nanofibers coated with willemite nanoparticles for improvement of stem cell osteogenesis. Mater Sci Eng C. 2016;62:398–406.
- Rungswang W, Kotaki M, Shimojima T, et al. Role of surfactant on inducing specific microdomains of block copolymer: an example case from polystyrene-b-poly(ethylene-co-1-butene)-b-polystyrene (SEBS) electrospun thermoplastic-elastomer fiber containing polyethylene glycol lauryl ether (PGLE). Polymer. 2014;55:2068–2076.
- Morent R, De Geyter N, Desmet T, et al. Plasma surface modification of biodegradable polymers: a review plasma process. Polymer. 2011;8:171–190.
- Shao J, Chen C, Wang Y, et al. Applied surface science structure and surface nanomechanics of poly (l-lactide) from thermally induced phase separation process. Appl Surf Sci. 2012;258:6665–6671.
- Su Y, Su Q, Liu W, et al. Controlled release of bone morphogenetic protein-2 and dexamethasone loaded in core–shell PLLACL – collagen fibers for use in bone tissue engineering. Acta Biomater. 2012;8:763–771.
- Kim CH, Khil MS, Kim HY, et al. An improved hydrophilicity via electrospinning for enhanced cell attachment and proliferation. J Biomed Mater Res Part B Appl Biomater. 2006;78B:283–290.
- Kai Z, Ying D, Guo-qiang C. Effects of surface morphology on the biocompatibility of polyhydroxyalkanoates. Biochem Eng J. 2003;16:115–123.
- Declercq H, Van Den Vreken N, De Maeyer E, et al. Isolation, proliferation and differentiation of osteoblastic cells to study cell/biomaterial interactions: comparison of different isolation techniques and source. Biomaterials. 2004;25:757–768.
- Xiao L, Wang B, Yang G, et al. Poly (lactic acid)-based: synthesis, modification and applications. Biomaterials. 2006;247–282.