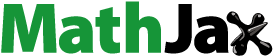
Abstract
In the present investigation, transgenic tobacco callus cultures and plants overexpressing the silicatein gene LoSilA1 from marine sponge Latrunculia oparinae were obtained and their bioreduction behaviour for the synthesis of silver nanoparticles (AgNPs) was studied. Synthesized nanoparticles were characterized using UV–visible spectroscopy, Fourier transformed infrared spectroscopy (FTIR), X-ray diffraction (XRD), scanning electron microscopy (SEM), energy-dispersive X-ray spectroscopy (EDX), atomic flame electron microscopy (AFM) and nanoparticle tracking analysis (NTA). Our measurements showed that the reduction of silver nitrate produced spherical AgNPs with diameters in the range of 12–80 nm. The results of XRD analysis proved the crystal nature of the obtained AgNPs. FTIR analysis indicated that particles are reduced and stabilized in solution by the capping agent, which is likely to be proteins present in the callus extract. Interestingly, the reduction potential of LoSiLA1-transgenic callus line was increased three-fold compared with the empty vector-transformed calli. The synthesized AgNPs were found to exhibit strong antibacterial activity against Escherichia coli and Agrobacterium rhizogenes. The present study reports the first evidence for using genetic engineering for activation of the reduction potential of plant cells for synthesis of biocidal AgNPs.
Introduction
Silver nanoparticles (AgNPs) have attracted the attention of researchers in the last two decades due to their wide applications in various fields. The surface plasmon properties of Ag nanomaterials are the foundation of a number of optics-based analytical techniques [Citation1]. The properties and uses of AgNPs in nanomedicine have also attracted increasing interest, such as employing their antimicrobial properties for wound healing [Citation2,Citation3]. Silver nanoparticles have been found to possess anti-inflammatory, antiviral, anti-angiogenesis and anti-platelet activity, as well as cytotoxicity against cancer cells, which makes them vitally important for prospective biomedical applications [Citation4–6].
Silver nanoparticles of specific sizes and morphologies can be synthesized using chemical and physical methods [Citation7]. However, these methods employ toxic chemicals as reducing agents, or non-biodegradable stabilizing agents and are therefore potentially dangerous to the environment and biological systems. Moreover, most of these methods entail intricate controls or nonstandard. Thus, development of efficient and environmentally friendly processes for synthesis of silver nanoparticles is one of the most challenging task of nanotechnology.
“Green” synthesis or phytosynthesis of metal nanoparticles has become an intensively studied area as an alternative to chemical and physical methods [Citation8,Citation9]. Many different plant species or their extracts were studied in case of their biosynthetic potential for the reduction of silver, gold, titanium and platinum ions [reviewed by Citation10–13]. More recently, plant cell cultures were utilized as a simple, cheap and eco-friendly approach. The first report showing that Carica papaya callus culture can be used for the synthesis of silver nanoparticles in the range of 60–80 nm was published by Mude and coauthors [Citation14]. Silver nanoparticles were subsequently obtained using extracts of Citrullus colocynthis [Citation15], Costus speciosus [Citation16] and Sesuvium portulacastrum [Citation17] cell cultures.
The biosynthetic potential of different plants or cell cultures for reduction of metal ions differed much and is evidently depends on individual biomolecules from extracts responsible for metal ions reductions. It seems clear that many different phytochemicals could potentially function as both the reducing and stabilizing agents. Commonly, polysaccharides, proteins and low-molecular weight secondary metabolites are considered the major reducing and capping agents present in plant extracts [Citation18–21].
Bioengineering of plant cell metabolism using genetic modifications is widely used in biotechnology for the production of valuable secondary compounds [Citation22], recombinant proteins [Citation23], polysaccharides [Citation24], oils [Citation25], antibodies [Citation26] and antigens [Citation27]. However, this opportunity has never been studied in term of the bioreduction of metal ions for the green synthesis of nanoparticles.
The formation of siliceous spicules in Demosponges is mediated by a family of water soluble self-assembling enzymes called silicateins [Citation28]. Recombinant silicatein catalysis bioinspired the synthesis of silica nanostructures of various size and morphology under mild conditions [Citation29]. Recently, the ability of silicatein to facilitate the formation of quartz, cristobalite and hexa-tetrahedral crystalline SiO2 was shown [Citation30]. Moreover, silicatein mediates the synthesis of titania, zirconia, gold and silver nanoparticles in solution at neutral pH and room temperature [Citation31–35].
The objective of this study was to study bioreduction potential of silicatein-expressing plant cells. For this aim, we obtained transgenic plants and callus cultures of Nicotiana tabacum expressing silicatein gene LoSilA1 from marine sponge Latrunculia oparinae. Our results demonstrate that the extract of LoSilA1-transgenic tobacco callus possesses pronounced ability for the reduction of silver ions when compared to control calli. To the best of our knowledge, this is the first report of the heterologous expression of recombinant silicatein in plant cells as well as the first example of utilizing genetically transformed plant cells in extracellular synthesis of metal nanoparticles.
Materials and methods
Silicatein LoSilA1 gene cloning and vector construction
The full-length sequence of the LoSilA1 gene of marine sponge L. oparinae was isolated and characterized by us previously [Citation36]. To amplify the fragment of LoSilA1, corresponding to mature silicatein protein, we used cDNA of L. oparinae and gene-specific primers: forward Sil-D 5′-GAT GAA TTC ATG TCC TAT CCT GAG TCC GTG-3′ (the EcoRI restriction site is underlined) and reverse Sil-R 5′-GTT GAG GGC CCA AGA GTA GGGT AGG AGG-3′ (the ApaI restriction site is underlined), which correspond to SYPES and SYPTL amino acid stretches located at N- and C-terminus of mature silicatein protein, respectively (Figure S1). PCR was performed using previously described primers and conditions [Citation37], whereby a 653-bp long LoSilA1 fragment was obtained.
Plasmids, pSAT6-MCS and pSAT6-EGFP-N1 [Citation38], both containing the tandem cauliflower mosaic virus (CaMV) 35 S promoter, tobacco etch virus (TEV) leader and the CaMV 35 S terminator, were used for construction of plant expression vectors. LoSilA1 was sub-cloned as an EcoRI-ApaI fragment into the same sites of the linearized plasmid. The obtained constructions pSAT6-LoSilA1 and pSAT6-LoSilA1-EGFP were checked for the absence of mutations by DNA sequencing, as described earlier [Citation39] at the Instrumental Centre of Biotechnology and Gene Engineering of FSCEATB FEB RAS using an ABI 3130 Genetic Analyzer (Applied Biosystems, Foster City, CA, USA). Both newly constructed expression cassettes and the original EGFP expression cassette from pSAT6-EGFP-N1 were further excised as PI-PspI-fragments and sub-cloned into the binary vector pPZP-RCS2-nptII [Citation38,Citation40] containing left and right border regions of Ti plasmid and a gene for kanamycin resistance (nptII) under the control of octopine synthase (ocs) promoter and terminator sequences. The final constructions (), pPZP-RCS2-nptII/LoSilA1, pPZP-RCS2-nptII/LoSilA1-EGFP and pPZP-RCS2-nptII/EGFP, together with the original empty vector, pPZP-RCS2-nptII, were transferred into Agrobacterium tumefaciens strain EHA105/pTiBo542 [Citation41] by electroporation (BioRad Gene Pulser, 0.1 cm cuvettes, 25mF, >2.5 kV) in accordance with the manufacturer’s protocol. Individual colonies were selected on LB medium containing 150 mg/l rifampicin, 300 mg/l spectinomycin and 200 mg/l streptomycin at 28 °C.
Figure 1. Map of T-DNA regions of binary vectors used in this study. (A) pPZP-RCS2-nptII, (B) pPZP-RCS2-nptII/LoSilA1, (C) pPZP-RCS2-nptII/LoSilA1-EGFP, (D) pPZP-RCS2-nptII/EGFP. OCS-P and OCS-T – octopine synthase promoter and terminator sequences; 35 S-P and 35 S-T – cauliflower mosaic virus 35 S promoter and terminator sequences; NPT II – the neomycin phosphotransferase gene; LoSilA1 – the silicatein A1 gene from the Latrunculia oparinae; EGFP – the enhanced green fluorescent protein gene.
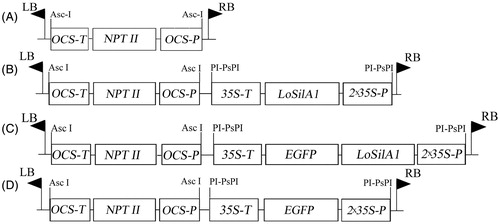
Transformation of tobacco
Agrobacterium tumefaciens carrying pPZP-RCS2-nptII, pPZP-RCS2-nptII/LoSilA1, pPZP-RCS2-nptII/LoSilA1-EGFP and pPZP-RCS2-nptII/EGFP plasmids were used to inoculate leaf discs of sterile, clonally cultivated plantlets of N. tabacum L. (cv Xanthi) according to the previously described conditions [Citation42]. Leaf discs were co-cultivated with A. tumefaciens for 48 h and transferred to the WB/A agarized medium supplemented with 0.5 mg/L 6-benzylaminopurine and 2.0 mg/L α-naphthaleneacetic acid [Citation43] containing 500 mg/L cefotaxime and 100 mg/L kanamycin. Transgenic callus cultures expressing the selectable marker (nptII) gene alone or together with the LoSilA1 gene, LoSilA1-EGFP fusion gene or EGFP gene, namely Nt-cV, Nt-cS, Nt-cSG and Nt-cG, were obtained after four months of selection with kanamycin. Plant regeneration was achieved by placing the 4–5 week-old primary calli on hormone-free W medium containing 100 mg/L kanamycin under a 16/8 h light/dark cycle. Thus, transgenic plantlets expressing the selectable marker (nptII) gene alone or together with the LoSilA1 gene, LoSilA1-EGFP fusion gene or EGFP gene, namely Nt-pV, Nt-pS, Nt-pSG and Nt-pG, were obtained. The control non-transformed callus culture and plants were established from the same plantlets and cultivated under the same conditions as the transformed ones.
Detection of nptII and LoSilA1 transcripts in transgenic tobacco calli and plants
Total RNA isolation and first-strand cDNA synthesis were carried out as described previously [Citation39,Citation44]. The primers 5′-GAG GCT ATT CGG CTA TGA CTG-3′ and 5′-ATC GGG AGC GGC GAT ACC GTA-3′ were used for the PCR amplification of a 700 bp nptII transcript (GenBank accession no. V00618). The primers 5′-TCA CCA TTT ACG AAC GAT AG-3′ and 5′-GCC ATA ACC AGT CAC CAC-3′ were used for the PCR amplification of a 531 bp LoSilA1 transcript (GenBank accession no. EU888269). The PCR reactions were carried out with these primers and the cDNA obtained from the N. tabacum calli or regenerated plantlets; fragments with the predicted length were selected for analysis. These fragments were isolated from gels with a Glass Milk Kit (Sileks M) and sub-cloned into a pTZ57R/T plasmid, using the InsT/Aclone PCR Product Cloning Kit (Fermentas, Vilnius, Lithuania). The clones were amplified with M13 universal primers and sequenced.
Laser confocal imaging of LoSilA-GFP fusion proteins in living cells
Cells of N. tabacum calli (25-day-old) and clonally cultivated plantlets (35-day-old) were used for the analysis. Excised organs and tissues were mounted in MS medium on cover glass slides (50 × 50 mm). The examination of fluorescence in living cells was performed with LSM 510 META and LSM 710 LIVE confocal laser scanning microscopes (Carl Zeiss) at the Instrumental Centre of Biotechnology and Gene Engineering of FSCEATB FEB RAS.
A 488-nm excitation wavelength was used to view EGFP and LoSilA1-GFP expression. Laser power was not over 5%. Lambda scan (515–700 nm) was used to record EGFP, LoSilA1-GFP and chlorophyll fluorescence and to exclude any possible autofluorescence. The image of LoSilA1-GFP fusion protein acquisition and analysis was created using LSM Image Browser and ZEN 2010 software (Carl Zeiss).
Preparation of extract and synthesis of silver nanoparticles
Freshly collected 30-day-old Nt-cV and Nt-cS calli or 40-day-old sterile Nt-pV and Nt-pS plantlets were used to obtain the extract. Plant material was washed with sterile distilled water to remove residues of medium components. Broth solutions were prepared by grinding of 1 g of cells in 5 ml of sterile distilled water using mortar and pestle. The resulting broth was centrifuged using Beckman Coulter Allegra X-22 centrifuge at 20,000g and supernatant was finally filtered through GE’s 0.45 µm Whatman filter and used for synthesis of silver nanoparticles. Typically, 1 ml of extract was mixed with 9 ml of 1 mM aqueous silver nitrate (AgNO3, Sigma-Aldrich) solution for the reduction of silver ions. As a control pure water was added to silver nitrate instead of extract. The reaction was carried out at 25 °C for 24 h and 150 rpm. After incubation, silver nanoparticles were purified three times by centrifugation at 20,000g for 20 min followed by redispersion of the pellet in sterile distilled water. Finally, the nanoparticle pellet was resuspended in 1 ml of water and used for analysis.
Characterization of silver nanoparticles
Ultraviolet–visible spectroscopy (UV–vis) was performed on a Bio-Rad SmartSpec Plus instrument operating at 1 nm resolution with 10 mm pathlength. Fourier transformed infrared spectroscopy (FTIR) was carried out on a Nicolet 6700 spectrometer (Thermo Electron, USA) in the range 450–4000 cm−1 in KBr pellets in the Laboratory of Micro- and Nanoscale Researches at Far East Geological Institute (FEGI FEB RAS). The crystalline structure of the synthesized AgNPs was investigated by X-ray diffraction (XRD). The diffraction pattern was recorded in the scanning mode on a Rigaku Miniflex II operated at 30 kV with a current of 15 mA and Cu/Kα radiation in the range of 3°–80° in 2θ angles. Results were compared with the standard powder diffraction card no. 04-0783 of JCPDS (Joint Committee on Powder Diffraction Standards). The average particle size of the synthesized AgNPs was calculated by using the Scherrer equation as follows:
where λ is the X-ray wavelength (1.5418 Ǻ), β is the full width at half maximum (FWHM) and θ is the diffraction angle.
The morphology of the synthesized silver nanoparticles was characterized by scanning electron microscopy (SEM) using Hitachi S-5500 (Japan) at the accelerating voltage of 2.0 kV. The local energy-dispersive spectroscopy (EDS) data were obtained on a Thermo Scientific (USA) spectrometer. Purified nanoparticles were also characterized using an atomic-force microscopy (AFM) using a Pacific Nanotechnology, Inc. Nano-DST (USA). A small volume of sample was spread on a well-cleaned glass cover slip surface mounted on the AFM stub and was dried in vacuum at room temperature. Images were obtained in tapping mode using a silicon probe cantilever of 225 μm length, resonance frequency 145–230 kHz, spring constant 20–95 N/m. Hydrodynamic diameter and zeta potential of the obtained nanoparticles were measured by nanoparticle tracking analysis (NTA) using a Nanosight NS500 system (NanoSight, UK) following the manufacturer’s instructions. Samples were diluted with water to obtain approximately 20 particles per image before being analysed with the NTA system. The measurements were made at room temperature and 60 s capture of video clips of particle movement under Brownian motion. The captured videos (10 videos per sample) were then processed and analysed by NTA analytical software version 2.2. For zeta potential estimation, the script for video recording and analysis designed by the manufacturer was employed.
Antibacterial activity of silver nanoparticles
Disc diffusion assay was performed to check the antibacterial properties of AgNPs against human and plant pathogenic Gram-negative Escherichia coli XL1 and A. tumefaciens EHA105 bacteria, respectively. Bacterial strains were grown aseptically in Luria-Bertani (LB) broth for at 37 °C (E. coli) or 28 °C (A. tumefaciens). The cultures were allowed to grow until an optical density of 0.4 containing 1 × 106 CFU/ml was obtained at 600 nm (OD600) and spread on LB agar plates. Sterile Whatman filter paper discs with a 6 mm dimension were loaded with 10, 20, 40 and 60 μg/disk of synthesized AgNPs and allowed to dry at room temperature. The discs were placed on the inoculated agar plates and incubated in an inverted position at 37 °C for 24 h. The plates were examined for the presence of zones of inhibition, indicated by a clear area around the discs. The diameters of inhibition zones were measured and the mean values for each concentration were recorded. Pearson correlation analysis was used to reveal relationships between AgNPs concentrations and the diameter of the observed inhibition zones with Statistica 10.0 (StatSoft Inc., Tulsa, OK, USA).
The bacterial growth kinetics was monitored in the presence of AgNPs at different concentrations (1, 5, 10 and 20 μg/mL) with cells of each bacterial strain grown in LB broth medium. They were incubated at corresponding temperatures and the OD600 was measured at an interval of 1–25 h, with triplicate samples being taken for analysis.
Results and discussion
Obtaining N. tabacum transgenic plants and callus cultures expressing silicatein gene
Among the four silicatein genes of L. oparinae, LoSilA1 was expressed at least 10 times higher than others [Citation36], and was chosen for our analysis. To create a plant-based silicatein-expressing system, we cloned a partial sequence of LoSilA1 gene which corresponds to its mature enzyme. The transgenic calli Nt-cV (callus line transformed with an empty vector), Nt-cS (callus line transformed with the LoSilA1 gene), Nt-cSG (callus line transformed with the LoSilA1 gene fused to the N-terminus of the EGFP gene) and Nt-cG (callus line transformed with the EGFP gene) were obtained via Agrobacterium-mediated transformation of N. tabacum leaf explants. Transgenic plants were regenerated from the corresponding callus cultures to obtain Nt-pV, Nt-pS, Nt-pSG and Nt-pG lines. cDNA samples were prepared from transgenic cultures and plants and RT-PCR amplification with primers corresponding to actin gene was performed to verify that the cDNA was native and free of genomic DNA (). Gene-specific RT-PCR analysis showed that all transformed tobacco calli and plant lines expressed the nptII gene (), among which, Nt-cS, Nt-cSG, Nt-pS and Nt-pSG expressed both nptII and LoSilA1 gene sequences (). EGFP transcript was detected in cDNA samples of Nt-cG, Nt-cSG, Nt-pG and Nt-pSG (). Whereas the heterologous production of functional silicatein in bacteria was previously described [Citation45–47], this is the first report of a eukaryotic-based expression system.
Figure 2. Electrophoretic separation of the PCR products. Nc, negative controls; Pc, positive controls; Nt-c and Nt-p, non-transformed callus and plant lines; Nt-cV and Nt-pV, callus and plant lines transformed with empty vector; Nt-cS and Nt-pS, callus and plant lines transformed with LoSilA1 gene; Nt-cSG and Nt-pSG, callus and plant lines transformed with LoSilA1 gene fused to a N-terminus of EGFP gene; Nt-cG and Nt-pG, callus and plant lines transformed with the EGFP gene.
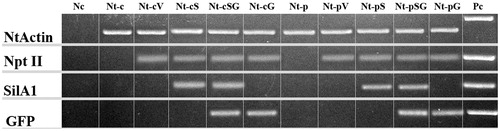
Subcellular locations of LoSilA1-GFP fusion protein in transgenic tobacco plant and callus culture
EGFP was used as a visual marker to follow the distribution of recombinant LoSilA1-EGFP in stable transgenic callus culture and plants (Nt-cSG and Nt-pSG, respectively). Nt-cG and Nt-pG lines which expressed the EGFP gene alone were used as a control. Mature LoSilA1 was engineered with an N-terminal EGFP and was expressed under the control of a cauliflower mosaic virus 35 S promoter. The presence of fluorescence in each subcellular compartment (nucleus, vesicle and cytoplasm) of particular cell types were compared with control cells and between themselves. The subcellular distribution of EGFP alone is shown as a control in . These data support related studies in which the EGFP protein is typically cytosolic and nuclear when expressed in plant cells and protoplasts [Citation48,Citation49], with a standard distribution in the cytoplasm and nuclei. The LoSilA1-EGFP fusion revealed similar subcellular distribution patterns in calli and plant cells (). The pure EGFP and recombinant silicatein were mainly distributed in the cytoplasm and nuclei. The z-stack processing of whole cells of LoSilA1 in calli revealed small, often spherical organelles which were approximately 0.5–1.5 μm in diameter with bright EGFP fluorescence distributed along the ER (endoplasmic reticulum) (data not shown). In plants, silicatein accumulated to high levels in stomatal guard cells. The patterns shown were observed consistently, even at the lower limits of detection in weakly fluorescent cells. This supports the contention that the observed localization patterns are not an artifact of extreme overexpression but rather reflect realistic targeting potentials for endogenous enzymes. The absence of different autofluorescence or chloroplast fluorescence at the original position of the 515–530 nm was demonstrated using the λ-scan mode (Figure S2). EGFP fluorescence was more readily detected in younger leaves and calli cell clusters than in older tissue parts, consistent with the reports that younger tissue has higher levels of protein expression [Citation50].
Figure 3. Live imaging of subcellular distribution of LoSilA1 in transgenic N. tabacum callus cultures (A) and plants (B). Phenotypes of the LoSilA1-EGFP transgenic callus and plant (A1 and B1, respectively). Confocal images of tobacco cells that express empty vector (A2 and B2, respectively) either EGFP alone (A3 and B3) or LoSilA1-EGFP fusion (A4 and B4) proteins. Images were generated using the fluorescent channel only.
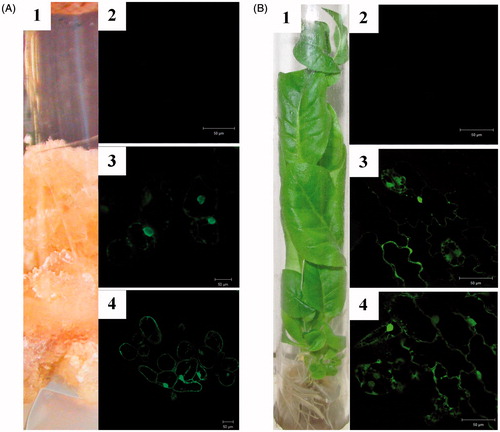
Synthesis of silver nanoparticles
The extracts of transgenic LoSilA1-transgenic calli and plants (Nt-cS and Nt-pS, respectively) were used to evaluate the ability of silicatein gene expression and change nanoparticle synthesis ability in plant systems. In these experiments, calli and plants which were transformed with empty vector (Nt-cV and Nt-pV, respectively) and thus expressed the nptII gene alone were used as a control. Upon incubation of reaction mixtures containing Nt-cV and Nt-cS callus extracts, the colour change was noted by visual observation (). The appearance of a dark brown colour in the reaction mixture commonly indicates the formation of Ag nanoparticles [Citation51,Citation52] and is not visible in the control. Notably, the colour intensity was higher with Nt-cS extract than with Nt-cV extract. At the same time, Nt-pV and Nt-pS plant extracts did not provoke any distinct changes in the colour of reaction mixtures; instead, a brown precipitate on flasks bottoms and walls was formed (). The latter effect was rather unexpected, as N. tabacum extracts were previously utilized for the reduction of silver ions [Citation53,Citation54]. However, in these reports procedure for extract preparation significantly differed from ours. In one case, a long-term boiling of tobacco leaves was employed [Citation54] and in another Tris–HCl buffer was used to prepare precursor for synthesis of AgNPs [Citation53]. Thus, extraction parameters could considerably affect the effectiveness of nanoparticle synthesis via green approach.
Characterization of silver nanoparticles
Since metal nanoparticles are known to show a surface plasmon response (SPR), UV–vis absorbance spectroscopy was employed to give first evidence of AgNP formation. UV–vis spectrophotometric analysis can also be used to evaluate the production yield of nanoparticles. UV absorption spectra of the AgNPs formed in the reaction media has high absorbance peaks at 440 nm for both Nt-cV and Nt-cS mediated nanoparticles (). The plasmon bands are broad with an absorption tail in the longer wavelengths. This broadening of the plasmon band could be due to the wide size and shape distribution of the particles [Citation55]. The level of absorption reached 0.7 and 2.5 after 24 h of incubation for Nt-cV and Nt-cS extracts, respectively, thus indicating that nanoparticle synthesis potential of Nt-cS calli was 3.6 times higher than that of control cells. Although it was likely that AgNPs were formed when Nt-pV and Nt-pS extracts were employed, as seen from the brown precipitate in solution, no SPR peaks were detected, possibly due to their aggregation.
Figure 6. UV–Visible spectra of silver nanoparticles prepared using extracts from callus and plant lines of N. tabacum. Nt-cV and Nt-pV – callus and plant transformed with empty vector; Nt-cS and Nt-pS – callus and plant transformed with the LoSilA1 gene. Control flasks contained silver nitrate solution without adding callus extract.
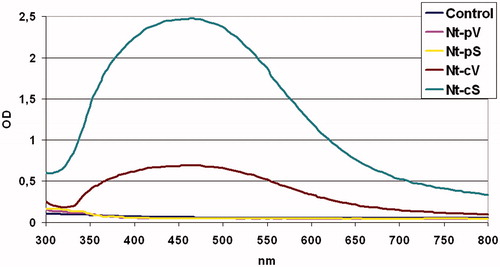
FTIR measurements were carried out to identify the possible biomolecules responsible for the reduction of silver ions in Nt-cS samples. Representative absorption spectra of the nanoparticles obtained is presented in (and indicates the presence of various chemical groups). It clearly reveals the major peaks associated with proteins. Among them, the absorption peaks at about 3376 and 3063 cm−1 correspond to N–H stretching vibrations of the amide A and B bands, respectively [Citation56]. The band observed at 2362 cm−1 was assigned to the primary amine (–NH2) group [Citation57]. An amide I band (between 1600 and 1690 cm−1) is mainly associated with the C = O stretching vibration [Citation58]. The absorbance peaks at 1388–1445 cm−1 and 1247 − 1301 cm−1 correspond to the amide II and III (C–N stretching, N–H bending) group, respectively [Citation59]. The peak at 1534 cm−1 may be assigned to the symmetric stretching vibrations of –COO– (carboxylate ion) groups of amino acid residues with free carboxylate groups in the protein [Citation22]. In addition, absorption at 1100–1160 cm−1 can be attributed to the ether (C–O) and acetal ring (C–O–C) bands [Citation60]. The results confirmed that proteins present in Nt-cS extract could be responsible for the reduction. Similar FTIR spectra were obtained for insulin incorporated in SiO2 matrices [Citation61] and Aspergillus tubingensis proteins adsorbed to the AgNPs [Citation62]. Moreover, silicatein proteins immobilized with nanofibrils showed characteristic amide I and A bands [Citation63].
Figure 7. FTIR spectra of silver nanoparticles synthesized using Nt-cV (A) and Nt-cS (B) callus extracts.
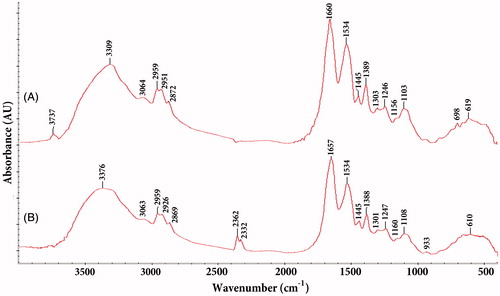
The crystalline nature of the AgNPs was examined with XRD analysis (). The peaks at 2θ = 32.26, 38.14, 46.27, 64.49 corresponding to (101), (111), (200) and (220) Bragg’s reflection planes were observed and compared with the standard powder diffraction card of JCPDS, silver file No. 04–0783. The XRD results indicate that the synthesized particles are face-centred cubic structures of silver similar to biologically synthesized AgNPs reported previously [Citation51,Citation64]. The mean size of AgNPs was calculated using the Scherrer formula and found to be about 12 nm.
Scanning electron microscopy analysis was further employed to investigate the size and morphology of the synthesized AgNPs (). The AgNPs were spherical in shape and were mostly dispersed. The size of the particles varied from 10 to 40 nm. The presence of metallic silver in the synthesized AgNPs was confirmed by the EDS analysis (). The EDS spectrum showed high intense silver peak in the range of 2.5–3.5 keV, which is the characteristic absorption of metallic silver; similar results were also reported earlier [Citation65].
Figure 9. SEM images and EDS spectra of AgNPs synthesized using Nt-cV (A) and Nt-cS (B) callus extracts. Scale bar 200 nm.
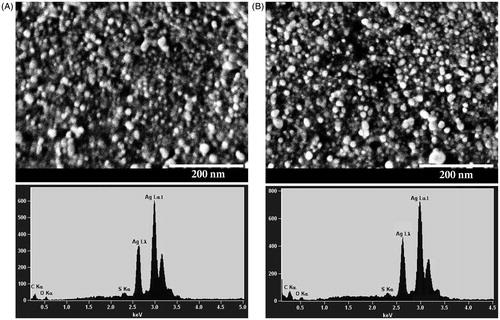
Atomic flame electron microscopy analysis confirmed our results obtained with SEM and showed dispersed nanoparticles with spherical morphology of about 30 nm in diameter ().
Figure 10. AFM topographic and phase images of AgNPs synthesized using Nt-cV (A) and Nt-cS (B) callus extracts. Scale bar 100 and 200 nm, respectively.
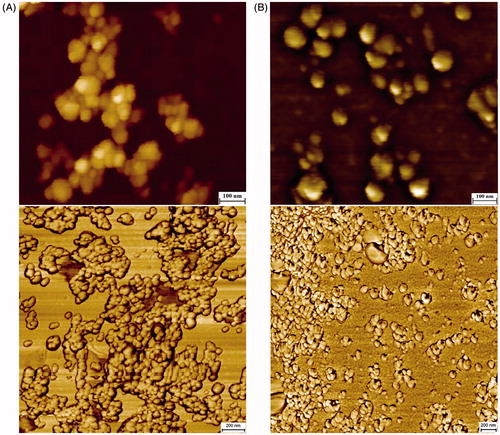
The NTA measurement revealed the size distribution of the AgNPs with a mean diameter of 63.6 and 53.5 nm for the Nt-cV and Nt-cS samples, respectively (). The zeta potential of synthesized nanoparticles was −30.2 and −29.4 mV for the Nt-cV and Nt-cS samples, respectively, which indicates that the synthesized nanoparticles have a high degree of stability [Citation66]. The total concentration of AgNPs measured with NTA in the Nt-cV sample was 3.1-fold lower than in the Nt-cS sample (179.7 and 551.4 E10/ml, respectively). Comparing the AgNPs concentration in Nt-cV and Nt-cS samples from the two calculation technologies (OD and NTA) reveals that the fold change values are highly correlated.
Interestingly, the size values of AgNPs obtained by XRD, SEM, AFM and NTA are different. This difference can be partially explained by the fact that these particles are actually not ideally spherical. The non-spherical shape of the particles can lead to the misrepresentation of sizes in both XRD and AFM analysis [Citation67,Citation68]. It is also worth noting that imperfect face-centred cubic structures, the high proportion of atoms at the surface or impurities disturb the translational symmetry characteristic of the crystals making Scherrer formula unreliable at estimating particle size calculated from full width at half maximum (FWHM) of the XRD peaks [Citation69]. Additionally, the crystallite size estimated using the Scherrer formula is smaller than the actual particle size obtained by microscopic analysis in case of their polycrystalline structure [Citation70,Citation71]. As for NTA, the hydrodynamic diameter values are always larger than the core size of the particles measured using other techniques and depend on the surface properties [Citation72].
Many proteins and peptides recognize specific inorganic compounds and are able to control nanostructure formation both in vitro and in vivo [Citation73]. For instance, the controlled synthesis of magnetite crystals was achieved using the recombinant Mms6 protein derived from Magnetospirillum magneticum [Citation74] and in human mesenchymal stem cells transfected with the mms6 gene [Citation75]. The silver-binding peptides AG3, AG4 and AG5 serve as templates for nucleation and the growth of AgNPs in solution [Citation76] and could be used to manipulate bacterial interactions with heavy metals [Citation77]. A periplasmic chaperone of silver ions SilE binds Ag+ and promotes silver tolerance [Citation78]. The Sil gene cluster was involved in the extracellular synthesis of copper and silver nanoparticles [Citation79]. Similarly, silicateins are known to promote biosilica formation in nature [Citation80,Citation81], whereas recombinant silicatein proteins facilitate the synthesis of both silica and metal nanocrystals [Citation82]. Proteins are frequently associated with metal nanoparticles obtained via green synthesis using plant extracts [Citation83]. The molecular mechanism underlying the protein-induced reduction of metal ions depends on amino acid composition and the catalytic activity of proteins participating in nanoparticle formation [Citation84,Citation85]. Particular amino acids are supposed to play pivotal roles in this process including tyrosine [Citation86], tryptophan [Citation87], histidine [Citation88], proline and hydroxyl-containing amino acid residues [Citation76]. Interestingly, extracellular cationic proteins from Fusarium oxysporum, which are rather similar to silicatein, were shown to be responsible for the synthesis of zirconia nanoparticles at room temperature [Citation89]. Moreover, other genera of fungi were not capable of producing nanocrystalline zirconia. Thus, our data and those in the literature allow us to suggest that the expression of particular proteins could significantly affect the potential of organisms in nanoparticle synthesis.
Determination of the antibacterial activity
The antibacterial efficiency of synthesized AgNPs against human and plant pathogenic Gram-negative E. coli and A. tumefaciens bacteria was analysed by disc diffusion assay. Our results showed that both bacteria are highly susceptible to the AgNPs within the tested concentration range (). It was observed that the zone of inhibition increased with an increase in the concentration of AgNPs. Pearson correlation analysis revealed a high positive correlation dependence between AgNPs concentration and zone of inhibition in both E. coli and A. tumefaciens (r = 0.880, p = 0.001 and r = 0.740, p = 0.015, respectively).
Table 1. Average zone of inhibition (mm) of AgNPs against E. coli and A. tumefaciens.
The bacterial growth kinetics () were also examined with 106 CFU of E. coli and A. tumefaciens treated with different concentrations of AgNPs (1, 5, 10 and 20 μg/mL). The bacterial growth curves were studied by measuring the OD600 values at intervals of 1–26 h. It is notable from the graphs that the growth rate of E. coli and A. tumefaciens decreases according to the increasing AgNPs concentration and with time of exposure. The half maximal inhibitory concentration (IC50) values for the E. coli and A. tumefaciens, determined by kinetic assays, were 7 and 4 μg/mL, respectively. In general, the antibacterial effect of AgNPs obtained using Nt-cS extract was found to be similar to that described in the earlier reports [Citation90,Citation91].
Figure 12. Determination of growth kinetics of E. coli and A. tumefaciens in presence of AgNPs. Data are shown as mean ± SE from three biological replicates.
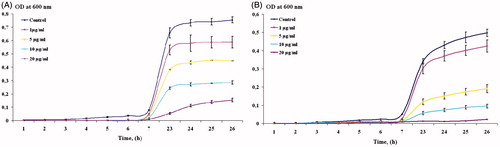
Although numerous reports describe the antimicrobial activities of AgNPs, the precise mechanism of their bactericidal effect remains not fully understood. It is considered that at the first step AgNPs attach to the surface of the bacteria causing the formation of irregularly shaped pits, disruption in membrane permeability, synthesis and energy generation [Citation92]. As soon as these effects correlate with surface area available for interaction, smaller AgNPs will give more bactericidal effect than larger particles [Citation93]. The shape of nanoparticles also significantly contribute to their mode of action [Citation94]. As AgNPs interact with bacteria surface via high atom density (111) facets [Citation94], decahedral or truncated triangular nanoparticles possessed higher reactivity in comparison to spherical or rod-shaped particles that contain less (111) facet percentages [Citation95].
Further, AgNPs penetrate the bacteria and interact with phosphorus-containing elements like DNA, sulphur-containing proteins and disulphide bonds of enzymes, thus causing further damage to cells [Citation96]. Finally, AgNPs are capable of releasing silver ions which interact with cytoplasmic components and nucleic acids [Citation94,Citation97,Citation98]. In accordance with this, aerobic bacteria are more vulnerable to the toxic effect of AgNPs than anaerobic bacteria [Citation93]. Upon oxidation under acidic conditions, Ag+ can freely release from AgNPs while insufficient air represses this process in anaerobic conditions [Citation99,Citation100].
Conclusions
This study reports the first example of the heterologous expression of the silicatein gene in transgenic plants and cell cultures. Recombinant silicatein accumulated at high levels in cytoplasm and nuclei, but it did not affect cell morphology or growth. Thus, plants could be considered an alternative protein expression system for obtaining and investigating the properties of silicatein.
Stable spherical AgNPs of small size were prepared using a simple green synthesis approach employing LoSilA1-expressing calli extract. Interestingly, the reduction potential of LoSilA1-transgenic callus culture extract was 3.6-times higher than that of control calli. Furthermore, the AgNPs exhibited antibacterial activity against human and plant pathogenic bacteria such as E. coli and A. tumefaciens, as revealed by the disk-diffusion method and kinetic assay.
This is the first report showing that genetic transformation can be used as a new biomimetic approach for the engineering of biocidal silver nanoparticles. In our case, silicatein, the protein which catalyses the synthesis of silica and metal nanoparticles in vitro, promotes the biosynthetic potential of a tobacco callus culture system for silver reduction.
Supplementary_figures.zip
Download Zip (2.6 MB)Acknowledgements
The analyses described in this work were performed using equipment from the Instrumental Centre for Biotechnology and Gene Engineering at the Federal Scientific Centre of the East Asia Terrestrial Biodiversity of the Far East Branch of Russian Academy of Sciences (Vladivostok) and Centre for Elemental and Isotopic Analysis at the Far East Geological Institute of the Far East Branch of Russian Academy of Science (Vladivostok). Financial support was provided by the Russian Science Foundation (RSF No. 17-74-10035) for the generation of transgenic cultures, cloning and confocal microscopy and by Far Eastern Branch of the Russian Academy of Sciences (FEB RAS No. 15-I-6-102) for the preparation, characterization and determination of the antibacterial activity of silver nanoparticles.
Disclosure statement
No potential conflict of interest was reported by the authors.
Additional information
Funding
References
- Kelly KL, Coronado E, Zhao LL, et al. The optical properties of metal nanoparticles: the influence of size, shape, and dielectric environment. J Phys Chem B. 2003;107:668–677.
- Wang C, Singh P, Kim YJ, et al. Characterization and antimicrobial application of biosynthesized gold and silver nanoparticles by using Microbacterium resistens. Artif Cells Nanomed Biotechnol. 2016;44:1714–1721.
- Singh P, Kim YJ, Wang C, et al. Weissella oryzae DC6-facilitated green synthesis of silver nanoparticles and their antimicrobial potential. Artif Cells Nanomed Biotechnol. 2016;44:1569–1575.
- Oka M, Tomioka T, Tomita K, et al. Inactivation of enveloped viruses by a silver-thiosulfate complex. Met Based Drugs. 1994;1:511
- Chandrakasan G, Seetharaman P, Gnanasekar S, et al. Xenorhabdus stockiae KT835471-mediated feasible biosynthesis of metal nanoparticles for their antibacterial and cytotoxic activities. Artif Cells Nanomed Biotechnol. 2017. [Epub ahead of print]. doi: 10.1080/21691401.2017.1282495.
- Tokumaru T, Shimizu Y, Fox CL. Antiviral activities of silver sulfadiazine in ocular infection. Res Commun Chem Pathol Pharmacol. 1984;8:151–158.
- Li Y, Duan X, Qian Y, et al. Nanocrystalline silver particles: synthesis, agglomeration, and sputtering induced by electron beam. J Colloid Interface Sci. 1999;209:347–349.
- Singh H, Du J, Singh P, et al. Ecofriendly synthesis of silver and gold nanoparticles by Euphrasia officinalis leaf extract and its biomedical applications. Artif Cells Nanomed Biotechnol. 2017. [Epub ahead of print]. doi: 10.1080/21691401.2017.1362417.
- Norouz Dizaji A, Yilmaz M, Piskin E. Silver or gold deposition onto magnetite nanoparticles by using plant extracts as reducing and stabilizing agents. Artif Cells Nanomed Biotechnol. 2016;44:1109–1115.
- Mohanpuria P, Rana NK, Yadav SK. Biosynthesis of nanoparticles: technological concepts and future applications. J Nanopart Res. 2008;10:507–517.
- Kumar V, Yadav SK. Plant-mediated synthesis of silver and gold nanoparticles and their applications. J Chem Technol Biotechnol. 2009;84:151–157.
- Sinha S, Pan I, Chandra P, et al. Nanoparticles fabrication using ambient biological resources. J Appl Biosci. 2009;19:1113–1130.
- Narayanan KB, Sakthivel N. Green synthesis of biogenic metal nanoparticles by terrestrial and aquatic phototrophic and heterotrophic eukaryotes and biocompatible agents. Adv Colloid Interface Sci. 2011;169:59–79.
- Mude N, Ingle A, Gade A, et al. Synthesis of silver nanoparticles using callus extract of Carica papaya – a first report. J Plant Biochem Biotechnol. 2009;18:83–86.
- Satyavani K, Gurudeeban S, Ramanathan T, et al. Biomedical potential of silver nanoparticles synthesized from calli cells of Citrullus colocynthis (L.) Schrad. J Nanobiotechnol. 2011;9:43–50.
- Malabadi RB, Meti1 NT, Mulgund GS, et al. Synthesis of silver nanoparticles from in vitro derived plants and callus cultures of Costus speciosus (Koen.); assessment of antibacterial activity. Res Plant Biol. 2012;2:32–42.
- Nabikhan A, Kandasamy K, Raj A, et al. Synthesis of antimicrobial silver nanoparticles by callus and leaf extracts from saltmarsh plant, Sesuvium portulacastrum L. Colloids Surf B Biointerfaces. 2010;79:488–493.
- Iravani R. Green synthesis of metal nanoparticles using plants. Green Chem. 2011;13:2638–2650.
- Park Y, Hong YN, Weyers A, et al. Polysaccharides and phytochemicals: a natural reservoir for the green synthesis of gold and silver nanoparticles. IET Nanobiotechnol. 2011;5:69–78.
- Richardson A, Janiec A, Chan BC, et al. Synthesis of silver nanoparticles: an undergraduate laboratory using a green approach. J Chem Educ. 2006;11:331–333.
- Durán N, Marcato PD, Durán M, et al. Mechanistic aspects in the biogenic synthesis of extracellular metal nanoparticles by peptides, bacteria, fungi, and plants. Appl Microbiol Biotechnol. 2011;90:1609–1624.
- Abdin MZ. Enhancing bioactive molecules in medicinal plants. In: Zhu Y, Tan B, Bay B, et al., editors. Natural products-essential resources for human. Singapore: World Scientific Publishing Co. Pvt. Ltd; 2007, p. 45–57.
- Kusnadi AR, Nikolov ZL, Howard JA. Production of recombinant proteins in transgenic plants: practical considerations. Biotechnol Bioeng. 1997;56:473–484.
- Kim KI, Shin KS, Jun WJ, et al. Effects of polysaccharides from rhizomes of Curcuma zedoaria on macrophage functions. Biosci Biotechnol Biochem. 2011;65:2369–2377.
- Haslam RP, Ruiz-Lopez N, Eastmond P, et al. The modification of plant oil composition via metabolic engineering – better nutrition by design. Plant Biotechnol J. 2013;11:157–168.
- Larrick JW, Yu L, Chen J, et al. Production of antibodies in transgenic plants. Res Immunol. 1998;149:603–608.
- Sala F, Manuela Rigano M, Barbante A, et al. Vaccine antigen production in transgenic plants: strategies, gene constructs and perspectives. Vaccine. 2003;21:803–808.
- Weaver JC, Morse DE. Molecular biology of demosponge axial filaments and their roles in biosilicification. Microsc Res Tech. 2003;62:356–367.
- Shkryl YN, Bulgakov VP, Veremeichik1 GN, et al. Bioinspired enzymatic synthesis of silica nanocrystals provided by recombinant silicatein from the marine sponge Latrunculia oparinae. Bioprocess Biosyst Eng. 2016;39:53–58.
- Guinel MJ-F, Norton MG. Blowing of silica microforms on silicon carbide. J Non Cryst Solids. 2005;351:251–257.
- Tahir MN, Théato P, Müller WEG, et al. Formation of layered titania and zirconia catalysed by surface-bound silicatein. Chem Commun (Camb). 2005;28:5533–5535.
- Tahir MN, Eberhardt M, Therese HA, et al. From single molecules to nanoscopically structured functional materials: au nanocrystal growth on TiO2 nanowires controlled by surface-bound silicatein. Angew Chem Int Ed Engl. 2006;45:4803–4809.
- Schröder HC, Wang X, Tremel W, et al. Biofabrication of biosilica-glass by living organisms. Nat Prod Rep. 2008;25:455–474.
- Sumerel JL, Yang W, Kisailus D, et al. Biocatalytic structuredirecting synthesis of titanium dioxide. Chem Mater. 2003;15:4804–4809.
- Bansal V, Rautaray D, Bharde A, et al. Fungus-mediated biosynthesis of silica and titania particles. J Mater Chem. 2005;15:2583–2589.
- Kozhemyako VB, Veremeichik GN, Shkryl YN, et al. Silicatein genes in spicule-forming and nonspicule-forming Pacific demosponges. Mar Biotechnol. 2010;12:403–409.
- Shkryl YN, Veremeichik GN, Makhazen DS, et al. Increase of anthraquinone content in Rubia cordifolia cells transformed by native and constitutively active forms of the AtCPK1 gene. Plant Cell Rep. 2016;35:1907–1916.
- Tzfira T, Tian GW, Lacroix B, et al. pSAT vectors: a modular series of plasmids for autofluorescent protein tagging and expression of multiple genes in plants. Plant Mol Biol. 2005;57:503–516.
- Shkryl YN, Veremeichik GN, Bulgakov VP, et al. Decreased ROS level and activation of antioxidant gene expression in Agrobacterium rhizogenes pRiA4-transformed calli of Rubia cordifolia. Planta. 2010;232:1023–1032.
- Goderis IJ, De Bolle MF, François IE, et al. A set of modular plant transformation vectors allowing flexible insertion of up to six expression units. Plant Mol Biol. 2002;50:17–27.
- Hood EE, Gelvin SB, Melchers LS, et al. Agrobacterium helper plasmids for gene transfer to plants. Transgenic Res. 1993;2:208–218.
- Günter EA, Shkryl YN, Popeyko OV, et al. Cell-wall polysaccharide composition and glycanase activity of Silene vulgaris callus transformed with rolB and rolC genes. Carbohydr Polym. 2015;118:52–59.
- Bulgakov VP, Gorpenchenko TY, Shkryl YN, et al. CDPK-driven changes in the intracellular ROS level and plant secondary metabolism. Bioeng Bugs. 2011;2:327–330.
- Shkryl YN, Veremeichik GN, Bulgakov VP, et al. The production of class III plant peroxidases in transgenic callus cultures transformed with the rolB gene of Agrobacterium rhizogenes. J Biotechnol. 2013;168:64–70.
- Curnow P, Bessette P, Kisailus D, et al. Enzymatic synthesis of layered titanium phosphates at low temperature and neutral pH by cell-surface display of silicatein-alpha. J Am Chem Soc. 2005;127:15749–15755.
- Müller WE, Engel S, Wang X, et al. Bioencapsulation of living bacteria (Escherichia coli) with poly(silicate) after transformation with silicatein-alpha gene. Biomaterials. 2008;7:771–779.
- Ki M-R, Jang E-K, Pack SP. Hypothetical cathepsin-like protein from Nematostella vectensis and its silicatein-like cathepsin mutant for biosilica production. Process Biochem. 2014;49:95–101.
- Haseloff J, Siemering KR, Prasher DC, et al. Removal of a cryptic intron and subcellular localization of green fluorescent protein are required to mark transgenic Arabidopsis plants brightly. Proc Natl Acad Sci USA. 1997;94:2122–2127.
- Ju H-J, Samuels TD, Wang Y-S, et al. The potato virus X TGBp2 movement protein associates with endoplasmic reticulum-derived vesicles during virus infection. J Plant Physiol. 2005;138:1877–1895.
- Chitilova E, Macas J. Galbaraith DW. Green fluorescent protein targeted to the nucleus, a transgenic phenotype useful for studies in plant biology. Ann Bot. 1999;83:645–654.
- Govarthanan M, Seo YS, Lee KJ, et al. Low-cost and eco-friendly synthesis of silver nanoparticles using coconut (Cocos nucifera) oil cake extract and its antibacterial activity. Artif Cells Nanomed Biotechnol. 2016;44:1878–1882.
- Dehghanizade S, Arasteh J, Mirzaie A. Green synthesis of silver nanoparticles using Anthemis atropatana extract: characterization and in vitro biological activities. Artif Cells Nanomed Biotechnol. 2017;3:1–9.
- Prasad KS, Pathak D, Patel A, et al. Biogenic synthesis of silver nanoparticles using Nicotiana tobaccum leaf extract and study of their antibacterial effect. Afr J Biotechnol. 2011;10:8122–8130.
- Kuchekar SR, Patil MP, Han S-H. Biosynthesis of silver nanoparticles using Nicotiana tabaccum leaf extract. World J Pharm Pharm Sci. 2015;4:1609–1616.
- Link S, El-Sayed MA. Size and temperature dependence of the plasmon absorption of colloidal gold nanoparticles. J Phys Chem B. 1999;103:4212–4217.
- Kong J, Yu S. Fourier transform infrared spectroscopic analysis of protein secondary structures. Acta Biochim Biophys Sinica. 2007;39:549–559.
- Jayaseelan C, Rahuman AA, Kirthi AV, et al. Novel microbial route to synthesize ZnO nanoparticles using Aeromonas hydrophila and their activity against pathogenic bacteria and fungi. Spectrochim Acta A Mol Biomol Spectrosc. 2012;90:78–84.
- Satyavani K, Ramanathan T, Gurudeeban S. Green synthesis of silver nanoparticles by using stem derived callus extract of Bitter apple (Citrullus colocynthis). Dig J Nanomater Biostruct. 2011;6:1019–1024.
- Ingle A, Rai M, Gade A, et al. Fusarium solani: a novel biological agent for the extracellular synthesis of silver nanoparticles. J Nanopart Res. 2009;11:2079–2085.
- Shankar SS, Ahmad A, Sastry M. Geranium leaf assisted biosynthesis of silver nanoparticles. Biotechnol Prog. 2003;19:1627–1631.
- Mansur HS, Orefice RL, Vasconcelos WL, et al. FTIR and UV-vis study of chemically engineered biomaterial surfaces for protein immobilization. Spectroscopy. 2002;16:351–360.
- Ballottin D, Fulaz S, Souza ML, et al. Elucidating protein involvement in the stabilization of the biogenic silver nanoparticles. Nanoscale Res Lett. 2016;11:313.
- Natalio F, Link T, Müller WEG, et al. Bioengineering of the silica-polymerizing enzyme silicatein-a for a targeted application to hydroxyapatite. Acta Biomater. 2010;6:3720–3728.
- Singh H, Du J, Singh P, et al. Ecofriendly synthesis of silver and gold nanoparticles by Euphrasia officinalis leaf extract and its biomedical applications. Artif Cells Nanomed Biotechnol. 2017;8:1–8.
- Du J, Singh H, Yi TH. Biosynthesis of silver nanoparticles by Novosphingobium sp. THG-C3 and their antimicrobial potential. Artif Cells Nanomed Biotechnol. 2017;45:211–217.
- Bhattacharjee S. DLS and zeta potential – What they are and what they are not? J Control Release. 2016;235:337–351.
- Egerton TA, Tooley IR. Physical characterization of titanium dioxide nanoparticles. Int J Cosmet Sci. 2014;36:195–206.
- Vůjtek M, Kubínek R, Zbořil R, et al. AFM monitoring of size changes during thermal syntheses of ferric oxide nanoparticles. Modern Research and Educational Topics in Microscopy. FORMATEX Microscopy Ser. 2007;2:528–533.
- Hall BD, Zanchet D, Ugarte D. Estimating nanoparticle size from diffraction measurements. J Appl Crystallogr. 2000;33:1335–1341.
- Akbari V, Moghim S, Mofid MR. Comparison of epothilone and taxol binding in yeast tubulin using molecular modeling. Avicenna J Med Biotechnol. 2011;3:167–175.
- Monshi A, Foroughi MR, Monshi MR. Modified Scherrer equation to estimate more accurately nano-crystallite size using XRD. World J Nano Sci Eng. 2012;2:154–160.
- Bantz C, Koshkina O, Lang T, et al. The surface properties of nanoparticles determine the agglomeration state and the size of the particles under physiological conditions. Beilstein J Nanotechnol. 2014;5:1774–1786.
- Chen CL, Rosi NL. Peptide-based methods for the preparation of nanostructured inorganic materials. Angew Chem Int Ed Engl. 2010;49:1924–1942.
- Amemiya Y, Arakaki A, Staniland SS, et al. Controlled formation of magnetite crystal by partial oxidation of ferrous hydroxide in the presence of recombinant magnetotactic bacterial protein Mms6. Biomaterials. 2007;28:5381–5389.
- Elfick A, Rischitor G, Mouras R, et al. Biosynthesis of magnetic nanoparticles by human mesenchymal stem cells following transfection with the magnetotactic bacterial gene mms6. Sci Rep. 2017;7:39755.
- Naik RR, Stringer SJ, Agarwal G, et al. Biomimetic synthesis and patterning of silver nanoparticles. Nat Mater. 2002;1:169–172.
- Sedlak RH, Hnilova M, Grosh C, et al. Engineered Escherichia coli silver-binding periplasmic protein that promotes silver tolerance. Appl Environ Microbiol. 2012;78:2289–2296.
- Asiani KR, Williams H, Bird L, et al. SilE is an intrinsically disordered periplasmic “molecular sponge” involved in bacterial silver resistance. Mol Microbiol. 2016;101:731–742.
- Ramanathan R, O'Mullane AP, Parikh RY, et al. Bacterial kinetics-controlled shape-directed biosynthesis of silver nanoplates using Morganella psychrotolerans. Langmuir. 2011;27:714–719.
- Kisailus D, Truong Q, Amemiya Y, et al. Self-assembled bifunctional surface mimics an enzymatic and templating protein for the synthesis of a metal oxide semiconductor. Proc Natl Acad Sci USA. 2006;103:5652–5657.
- Kisailus D, Choi JH, Weaver JC, et al. Enzymatic synthesis and nanostructural control of gallium oxide at low temperature. Adv Mater. 2005;17:314–318.
- Tahir MN, Theato P, Muller W, et al. Monitoring the formation of biosilica catalysed by histidine-tagged silicatein. Chem Commun (Camb). 2004;24:2848–2849.
- Shankar SS, Rai A, Ahmad A, et al. Rapid synthesis of Au, Ag, and bimetallic Au core-Ag shell nanoparticles using Neem (Azadirachta indica) leaf broth. J Colloid Interface Sci. 2004;275:496–502.
- Song JY, Kim BS. Rapid biological synthesis of silver nanoparticles using plant leaf extracts. Bioprocess Biosyst Eng. 2009;32:79–84.
- Song JY, Kwon EY, Kim BS. Biological synthesis of platinum nanoparticles using Diopyros kaki leaf extract. Bioprocess Biosyst Eng. 2010;33:159–164.
- Lee E, Kim DH, Woo Y, et al. Solution structure of peptide AG4 used to form silver nanoparticles. Biochem Biophys Res Commun. 2008;376:595–598.
- Tan YN, Lee JY, Wang DI. Uncovering the design rules for peptide synthesis of metal nanoparticles. J Am Chem Soc. 2010;132:5677–5686.
- Kracht S, Messerer M, Lang M, et al. Electron transfer in peptides: on the formation of silver nanoparticles. Angew Chem Int Ed Engl. 2015;54:2912–2916.
- Bansal V, Rautaray D, Ahmad A, et al. Biosynthesis of zirconia nanoparticles using the fungus Fusarium oxysporum. J Mater Chem. 2004;14:3303–3305.
- Ahmed Q, Gupta N, Kumar A, et al. Antibacterial efficacy of silver nanoparticles synthesized employing Terminalia arjuna bark extract. Artif Cells Nanomed Biotechnol. 2017;45:1–9.
- Hamid S, Zainab S, Faryal R, et al. Inhibition of secreted aspartyl proteinase activity in biofilms of Candida species by mycogenic silver nanoparticles. Artif Cells Nanomed Biotechnol. 2017;25:1–7.
- Amro NA, Kotra LP, Wadu-Mesthrige K, et al. High-resolution atomic force microscopy studies of the Escherichia coli outer membrane: structural basis for permeability. Langmuir. 2000;16:2789–2796.
- Lu Z, Rong K, Li J, et al. Size-dependent antibacterial activities of silver nanoparticles against oral anaerobic pathogenic bacteria. J Mater Sci Mater Med. 2013;24:1465–1471.
- Morones JR, Elechiguerra JL, Camacho A, et al. The bactericidal effect of silver nanoparticles. Nanotechnology. 2005;16:2346–2353.
- Pal S, Tak YK, Song JM. Does the antibacterial activity of silver nanoparticles depend on the shape of the nanoparticle? A study of the gram-negative bacterium Escherichia coli. Appl Environ Microbiol. 2007;73:1712–1720.
- Habash MB, Park AJ, Vis EC, et al. Synergy of silver nanoparticles and aztreonam against Pseudomonas aeruginosa PAO1 biofilms. Antimicrob Agents Chemother. 2014;58:5818–5830.
- Jung WK, Koo HC, Kim KW, et al. Antibacterial activity and mechanism of action of the silver ion in Staphylococcus aureus and Escherichia coli. Appl Environ Microbiol. 2008;74:2171–2178.
- Rai M, Kon K, Ingle A, et al. Broad-spectrum bioactivities of silver nanoparticles: the emerging trends and future prospects. Appl Microbiol Biotechnol. 2014;98:1951–1961.
- Sotiriou GA, Pratsinis SE. Antibacterial activity of nanosilver ions and particles. Environ Sci Technol. 2010;44:5649–5654.
- Xiu ZM, Zhang QB, Puppala HL, et al. Negligible particle-specific antibacterial activity of silver nanoparticles. Nano Lett. 2012;12:4271–4275.