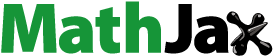
Abstract
The morphology of the polymer network – porous/less porous – plays predominant role in the amidase activities of the polymer catalysts in the hydrolytic reactions of amino acid p-nitroanilides. Polymers with the imprints of stable phosphonate analogue of the intermediate of hydrolytic reactions were synthesized as enzyme mimics. Molecular imprinting was carried out in thermodynamically stable porogen dimethyl sulphoxide and unstable porogen chloroform, to investigate the morphological effects of polymers on catalytic amidolysis. It was found that the medium of polymerization has vital influence in the amidase activities of the enzyme mimics. The morphological studies of the polymer catalysts were carried out by scanning electron microscopy and Bruner–Emmett–Teller analysis. The morphology of the polymer catalysts and their amidase activities are found to be dependent on the composition of reaction medium. The polymer catalyst prepared in dimethyl sulphoxide is observed to be efficient in 1:9 acetonitrile (ACN)–Tris HCl buffer and that prepared in chloroform is noticed to be stereo specifically and shape-selectively effective in 9:1 ACN–Tris HCl buffer. The solvent memory effect in catalytic amidolysis was investigated using the polymer prepared in acetonitrile.
Introduction
Specific molecular recognition is a fundamental requirement of living systems. Specific recognition is accredited to binding cavities that complement molecules in size, shape, hydrogen bonding and hydrophobic and electrostatic profiles [Citation1]. The binding cavity of the macromolecular matrix is designed to stabilize the reaction transition state, thereby lowering the activation energy requirement and resulting in reaction rate acceleration [Citation2–5]. Molecular imprinting is one of the most promising approaches for artificial bio-recognition [Citation6–12]. The functional monomers and crosslinkers are copolymerized around a template molecule which acts as a target molecule in a suitable medium. The high-crosslinking degrees of the polymer matrixes were designed to stabilize the size and shape of the imprinted cavities, thereby lowering the binding site flexibility and leading to an enhanced recognition power [Citation13,Citation14]. The porogen used stabilizes the H-bonding interactions between the monomers and template in the pre-polymerization complex and influences the morphology of the polymer network. The imprinted polymers are found to exhibit higher storage life and reusability without loss of the memory effect.
Molecularly imprinted polymers found lots of applications in the area of chiral separation, binding assays, sensors, etc. [Citation15–17]. Transition state analogue (TSA) imprinted polymers are continued to be as potential catalysts in esterolytic reactions [Citation6,Citation7,Citation10,Citation18]. Amidolytic reactions catalyzed by TSA imprinted polymers are less reported. We have reported the synthesis of chymotrypsin mimics using methacrylated L-amino acids ‘histidine, aspartic acid and serine’ [Citation19,Citation20] and their organic analogues [Citation21] and investigations on their amidase activities towards amino acid p-nitroanilides. There are reports on the solvent memory of polymers in the template rebinding studies [Citation22,Citation23]. But, the effect of porogens on the morphological features of the polymer catalysts and on their amidase activities is less studied yet. In this paper, we present the effect of thermodynamically stable and unstable porogens in making the polymer porous. The stereoselectivity and the substrate shape-selectivity of the polymer catalysts in amidolytic reactions of amino acid p-nitroanilides were demonstrated in detail. The solvent memory effect of the polymer was also investigated in detail.
Experimental
Materials
N-protected and free amino acids, α-chymotrypsin, benzyl carbamate, triphenyl phosphite and phenylacetaldehyde were purchased from SRL, Mumbai, India. Dicyclohexylcarbodiimide (DCC) and ethylene glycol dimethacrylate (EGDMA) were purchased from Sigma Aldrich, St. Louis, MO. Other chemicals, especially the solvents used as porogens were purified prior to use by following the standard procedures.
Instrumentation
IR spectra were recorded on a Shimadzu FT-IR-8400S spectrophotometer. Kinetic studies were performed using Shimadzu UV 2450 spectrophotometer. 1H NMR spectra were taken using Bruker Advance DPX-300 MHz FT-NMR spectrometer (Billerica, MA, USA) in CDCl3. BET analyzer used was Thermo Fisher Scientific Surface Analyzer V-230 50/60 Hz (Monza, Italy). JEOL JSM6390 SEM analyzer (Peabody, MA, USA) was used for SEM analysis.
Synthesis of print molecule
The phosphonate TSA, phenyl-1-(N-benzyloxycarbonylamino)-2-(phenyl)ethyl phosphonate was synthesized as the print molecule by acid catalyzed condensation of 2.0 g (13.20 mmol) benzyl carbamate, 4.10 mL (13.20 mmol) triphenyl phosphite and 2.38 mL (19.80 mmol) phenylacetaldehyde in glacial acetic acid (2.0 mL) for 4 h at 100 °C in an oil bath [Citation19]. FTIR: −1301 cm−1 (P=O stretching), 946 cm−1 (P–OH stretching) and 1252 cm−1 (P–O–phenyl stretching).
Design and synthesis of artificial amidase polymers
The amidase MIP C1 was prepared by radical initiated polymerization of methacrylated amino acid functional monomers; 296.59 mg MA-L-His (1.33 mmol), 231.42 mg MA-L-Ser (1.33 mmol), 259.35 mg MA-L-Asp (1.33 mmol) and 5.18 mL of the crosslinking agent EGDMA (9 mmol) in the presence of 205.5 mg TSA (0.50 mmol) in the porogen DMSO (40 mL) for 6 h at 80 °C. The print TSA was absolutely leached out by washing with methanol and then subjected to Soxhlet extraction with chloroform. The polymer obtained was collected and dried under vacuum.
The polymer catalysts C2 and C3 were also prepared as per the same methodology using ACN and CHCl3 as porogen. The corresponding non-imprinted control polymers (CP1–CP3) were also prepared by the same procedure in the absence of TSA.
The total functional group contents (TFGCs) in the polymer catalysts were determined by estimating the amino acid content by ninhydrin reagent by hydrolyzing the amino acids from the polymer backbone. The morphology of the polymers was characterized SEM and BET analysis.
Synthesis of the substrate Z-L-phenylalanine p-nitroanilide
The substrate Z-L-Phe-PNA was synthesized by means of DCC coupling by dissolving 299 mg (1 mmol) Z-L-phenylalanine and 138 mg (1 mmol) p-nitroaniline in ethyl acetate (30 mL). The product obtained was recrystallized from hot ethanol containing 1% acetic acid. The substrates, t-Boc/Nphth/Fmoc-L-phenylalanyl-p-nitroanilide and Z-L-tyrosine/alanine/tryptophan p-nitroanilide were also prepared following the same procedure.
Evaluation of amidase activity of the artificial amidases
The hydrolytic reaction of amino acid p-nitroanilide was carried out in acetonitrile (ACN)–Tris HCl buffer (5 mL, 1:9 by volume, pH 7.75). A suspension of 10 mg (0.00542 mmol) of the amidase MIP C1 was taken in a sealed reaction bottle, to which 271 mg (0.542 mmol) of the substrate Z-Phe-PNA in acetonitrile (50 mL) was added. The reaction mixture was placed in a water bath shaker at 45 °C and shaken gently. The amidolytic activity of the mimic was determined by measuring the absorbance of released p-nitroaniline spectrophotometrically at 374 nm. The degree of self-amidolysis of the substrate was also evaluated without the polymer catalysts under the same conditions. The reactions were monitored for 7 d to estimate the absorbance at infinite time A∞ and the rate constant k of the reaction was calculated from the slope of the plot ln [A∞/(A∞−At)] versus time plot.
Hydrolase activity of the amidase MIPs C2 and C3 was also evaluated in a similar manner. Activity measurements were conducted at least four times and the average of the results were reported here. The experimental errors of the results were less than ±5%. Since the heterogeneity of the polymer catalysts and solvation problems, the results show some deviations in reproducibility.
TSA rebinding studies
To investigate the specific rebinding of the imprinted TSA molecules by the imprinted sites in the polymer matrix, 10 mg of various amidase MIPs and NIPs were shaken with 8 mL TSA solution of fixed concentration for 4 h. From the collected centrifugate, the concentration at which maximum amount of TSA bound by the polymer is determined. The experiments were done in triplicate and the average values are reported.
TSA inhibition studies
The influence of the phosphonate TSA in inhibiting amidolytic reactions catalyzed by the amidase MIPs was investigated. Three different inhibitor concentrations such as half, equal and twice the concentration of the substrate were added to the hydrolysis media. The initial velocities were monitored in the same way. The rate constant for TSA rebinding was evaluated as inhibition constant Ki from the double-reciprocal plots. The substrate recognition power of the artificial enzyme was then estimated from the ratio Km / Ki.
Results and discussions
Design, synthesis and characterization of artificial amidases in different porogens
Porogenic solvents have an eminent role in making the polymer matrix macroporous to assure good flow-through properties. The nature of porogens determines the strength of non-covalent interactions and the morphology of the imprinted polymer. For comparing the effect of a thermodynamically stable and an unstable solvent on the morphology of the polymer matrix and its catalytic activity, the polymerization is carried out using DMSO (C1), ACN (C2) and chloroform (C3) as the porogen, the medium of polymerization. The details of the artificial amidases synthesized and their characterization are given in . The inner surface area and pore volume measurements gave good indication of morphological differences of the polymer catalysts [Citation24]. The polymer prepared in DMSO (C1) and ACN (C2) showed comparable surface area; but that prepared in chloroform (C3) showed less porous with surface area 201.35 m2/g. The enzyme mimic catalysts were characterized by SEM analysis. The control polymers have plain surfaces without pores. The surface of amidase MIP C1 prepared in DMSO is found to be highly porous and that of C3 prepared in CHCl3 is less porous ().
Table 1. Details of polymer synthesis and characterization.
Kinetics of catalytic amidolysis: porogen effect
Chymotrypsin specific and non-specific substrates are synthesized by DCC coupling of p-nitroaniline and amino acids ().
Table 2. Details of amino acid p-nitroanilides synthesized.
The amidolysis of substrate Z-L-Phe-PNA (S1) was carried out in 1:9 ACN–Tris HCl buffer of pH 7.75 at 45 °C in the frame work of Michaelis–Menten kinetics using the amidase MIPs and NIPs prepared in different porogens (DMSO, ACN & CHCl3) as catalysts. The rate acceleration ratios – (1.23),
(17.29) and
(14.11) – provide strong evidence for the effect of the medium of TSA imprinting on the amidase activity of the mimics. The catalytic amidolysis shows that, for preparing an effective enzyme mimic with macroporous matrix, DMSO is the best porogen, which leads to saturation in amidase activity within 22 h; the polymer C3 did not attain saturation even after several days (). The imprinting efficiency observed are 4.22 (C1), 3.67 (C2) and 1.01 (C3); which indicate that the mimic C3 prepared in CHCl3 behaves like control polymers.
Table 3. Kinetic parameters for the amidolysis of S1.
The polar, non-protic solvents stabilize the template-functional monomer pre-polymerization complex by maximizing the H-bonding interactions between the template and functional monomers. The possible mechanism of catalytic amidolysis is shown in .
The nucleophilic attack of the hydroxyl group was found to be facilitated by the cooperative interaction of imidazole and carboxyl functionalities as in the case of natural chymotrypsin.
Composition of reaction medium and catalytic amidolysis
shows that the artificial amidases prepared in different porogens exhibit remarkable dependence on the composition of the reaction medium ACN–Tris HCl buffer. The mimic C1 prepared in DMSO shows significant decrease in rate acceleration kacc as the solvent composition changes from 1:9 (75.38) to 3:7(49.65), 5:5 (27.34), 7:3 (12.78) and then to 9:1 (4.21). A similar trend is observed for C2 prepared in ACN. But, the mimic C3 prepared in CHCl3 shows its maximum catalytic efficiency in 9:1 ACN-Tris HCl buffer (52.32). As the acetonitrile content in the solvent mixture increases, the amidase activity of C1 and C2 becomes similar to that of uncatalyzed reaction due to the swelling of the macroporous polymer matrix in the reaction medium. The molecular imprint with specific reactive functionalities gets deformed which limits the correct positioning of the TS of the amidolysis in the cavity.
In the case of C3, rate acceleration is found to be increasing as the ACN in the reaction medium increases. The recognition sites of the rigid polymer C3 synthesized in chloroform are expected to be less deformed even though the ACN content in the solvent mixture increases. The accessibility of the catalytic sites increases leading to remarkably higher rate acceleration as the acetonitrile content in the solvent changes from 1:9 (5.87) to 9:1 (52.32). The amidase MIP C3 exhibited higher swelling capacity in 9:1 ACN–Tris HCl buffer and then only the polymer can enfold well-defined imprinted sites and hence increased catalytic activity. The details of the swelling studies are given in .
Table 4. Swelling studies.
In 1:9 ACN–Tris HCl buffer, the rate acceleration ratio observed are 1.23 () and 10.48 (
). But, in the 9:1 ACN–Tris HCl buffer, the reverse trend observed is 12.52 (
) and 12.43 (
). Further, the saturation time St (h) for C1 in 1:9 ACN–Tris HCl buffer is only 22 h, while C3 in 9:1 ACN-Tris HCl buffer did not show saturation in amidolysis even after 120 h. Hence, C1 exhibits superior catalytic activity over C3 (). Further, the CPs did not exhibit any remarkable rate acceleration towards S1.
Michaelis–Menten kinetic studies
A fine perceptive of the catalytic amidolysis can be obtained by the Michaelis–Menten kinetic study correspondingly as it is carried out with enzymes [Citation25,Citation26].
As shown in , in the case of C1 and C2, typical saturation kinetics is obtained at higher substrate concentrations in 1:9 ACN–Tris HCl buffer; while C3 shows saturation kinetics in 9:1 ACN–Tris HCl buffer. The unique form of the Michaelis–Menten plot as it is also established for natural enzymes clearly shows that although the mimic correspond to insoluble catalyst, they really perform very similar to soluble enzymes.
In 1:9 ACN–Tris HCl buffer, the amidase MIP C1 exhibits similar substrate affinity to C2, while higher substrate affinities than C3 which is evidenced from the ratios (1.293) and
(32.777). The corresponding value in 9:1 ACN–Tris HCl buffer (
=18.836 and
1.284) provides a good indication of the higher substrate affinities of C3. Even though C3 is catalytically efficient in 9:1 ACN–Tris HCl buffer, it exhibits 1.678 times lower substrate affinity than C1 which is effectual in 1:9 ACN–Tris HCl buffer (). The amidase MIP C1 showed 1.424 times overall catalytic efficiency than C3 in 9:1 ACN–Tris HCl buffer which is estimated as
. The plots reveal that C1 and C2 obey enzyme behaviour towards S1 in 1:9 ACN–Tris HCl buffer and C3 in 9:1 ACN–Tris HCl buffer. The kinetic parameters revealing enzyme behaviour are evaluated from the double reciprocal plots ().
Table 5. Kinetic parameters for the amidolysis S1 using the amidase MIPs.
depicts a comparison of the active sites from the natural chymotrypsin and the mimic. The imprinted cavity displays an analogous spatial structure with the ability for the stabilization of the tetrahedral TS of amidolytic reaction and with a comparable orientation of catalytic moieties being accountable for high amidase activity.
Effect of porogen on enantioselectivity of the polymer catalysts
The substrate stereo specificity of the amidase MIPs is investigated by carrying out the amidolysis of S1/S2 in 1:9 and 9:1 ACN–Tris HCl buffer. The substrate stereo specificity is evaluated as and
based on the values of
and
. The free energy values of the polymer catalysts are given in .
Table 6. Kinetic parameters for substrate stereospecificity of the artificial amidases.
The polymer C1 shows 8.961 times substrate affinity towards Z-L-phenylalanine p-nitroanilide than C3 in 1:9 ACN–Tris HCl buffer. The values showed better stabilization (15 times) of the TS of Z-L-Phe-PNA in the catalytic cavity of C1 1:9 ACN–Tris HCl buffer. Further, the enzyme-like behaviour of C1 is strongly evidenced from
value. A reverse trend is observed in 9:1 ACN–Tris HCl buffer solution and the results clearly reveal the nucleophilic catalyst like behaviour of C1 and C2; but enzyme-like behaviour of C3. A remarkable reduction in
values (6.77 times) give strong evidence for the deformation of the catalytic cavity in 9:1 ACN–Tris HCl buffer and poor stabilization of the T.S of Z-L-Phe-PNA. The shape fitting between transition state of the substrate and imprinted cavity in 9:1 ACN–Tris HCl buffer is better for C3, resulting in 6.480 and 61.550-fold larger
and
.
Porogen effect on substrate shape-selectivity of the polymer catalysts
Four different substrates S1, S3, S4 and S5 were used to investigate the role of size and shape of the imprinted cavity in governing the kinetics of amidolysis. The relative reactivities are summarized in . The substrate shape-selectivity of the amidase MIPs are evaluated in terms of substrate affinity ratio. The selectivity is observed in the order S1 > S3 > S4 > S5 because of the substrate S1 whose structure is most similar to that of the imprinted TSA molecule. The lower selectivities towards substrates S3, S4 and S5 are due to the difference in the structure of the N∞-protecting groups of the substrates. The substrate S5 provided the lowest reaction rate in all the substrates because the shape and the size of the N∞-protecting group which makes it quite different from the imprinted molecule. Based on these facts, it is found that the artificial amidase polymer recognized the structure of the print molecule and selectively catalyzed the substrate that has a similar structure of the print TSA molecule.
Table 7. Porogen effect on substrate shape-selectivity of the polymer catalysts.
The macro-porous amidase MIPs C1 (0.898 cm3/g) and C2 (0.676 cm3/g) gets suitably swelled in 1:9 ACN–Tris HCl buffer to access its cavities to S1. The less porous amidase MIP C3 (0.354 cm3/g) shows comparatively poor swelling in 1:9 ACN–Tris HCl buffer and due to the difficulty in accessing catalytic sites; negligibly small differences is noticed in Km values. As the ACN content in the solvent mixture increases, C3 exhibited higher swelling capacity; then only the polymer can have well defined imprinted sites and hence the accessibility of the catalytic sites increases leading to remarkably higher memory effect.
Porogen effect on the substrate specificity of the polymer catalysts
To investigate the effect of morphology of the artificial amidases on substrate specificity, amidolytic reactions of chymotrypsin specific substrates S1, S6 and S8 and non-specific substrate S9 are carried out. As the ACN content in the reaction medium increases, owing to extensive swelling and deformation of the substrate recognition sites, a reduction in substrate specificity is noticed for the macro porous polymer C1 (). The less porous polymer matrix C3 shows proper swelling and better accessibility of catalytic sites in 9:1 ACN–Tris HCl buffer and exhibits excellent substrate specificity. In 1:9 ACN–Tris HCl buffer, polymer C3 could not show any specificity towards S1, S6, S8 and S9 due to the lack of sufficient swelling and hence diffusion problems. The optimum specificity ratio observed for C1 in 1:9 ACN–Tris HCl buffer is 5.31 and for C3 in 9:1 ACN–Tris HCl buffer is 5.14.
Table 8. Porogen effect on the substrate specificity of the polymer catalysts.
Study on TSA inhibition effects
A typical property of enzymes is the inhibition by certain molecules. An artificial amidase MIP should show competitive inhibition by the template. A direct measurement of catalysis to occur inside the imprinted cavity can obtained from the competitive inhibition of TSA in the amidolysis catalyzed by the amidase MIPs and NIPs [Citation27,Citation28]. The TSA inhibition studies were carried out in the amidolytic reaction in the presence of the phosphonate TSA using the polymer catalysts C1–C3. As shown in , the amidase activity of the amidase MIPs was fairly inhibited, as the concentration of the template TSA was increased due to “the shape-selective rebinding” of the imprinted TSA over the substrate S1. This would be a further proof for catalysis to occur inside the imprinted cavity. In this respect, the catalytic the amidolysis of S1 was stopped completely under the condition of [TSA]/[S1] = 2.0, 3.0 and 1.0 for the polymers C1, C2 and C3, respectively. A better rebinding within shorter time is observed for C3 due to solvent memory of the polymer catalyst. There are reports on the solvent memory of polymers in the template rebinding studies. Molecularly imprinted polymers always exhibit maximum recognition capacity when the same porogen is used as the preparation and selectivity studies. The use of other solvents will cause destruction of the 3D structure of the imprints which are shape-complementary to the target molecule. It is notable that MIPs prepared in organic solvent work poorly in aqueous media because of the “solvent memory” [Citation21].
Now it is exciting to compare the binding of the mimic to the substrate anilide and to the TSA. Binding to the anilide is evaluated from the Michaelis–Menten constant Km and binding to the TSA can be estimated from the competitive inhibition caused by the added TSA during the catalytic amidolysis (Ki). The influence of the inhibiting template molecule was monitored with amidase MIP C1, prepared in DMSO. Two different inhibitor concentrations were added to the amidolysis media. The velocities were monitored in the same way under the same conditions of amidolysis (1:9 ACN–Tris HCl buffer of pH 7.75 and 45 °C). Evidently, the phosphonate TSA is a very effective inhibitor that binds much better than the substrate S1 by a factor of 33.56 when TSA is present in half the concentration of the substrate PNA [Ki = 0.075 mmol and Km = 2.516 mmol] and by a factor of 66.70 when equal concentration of TSA and substrate are used [Ki = 0.056 mmol and Km = 3.735 mmol]. Since the straight lines intersect in the negative part of the plot the TSA not only bind with the polymer catalyst (C1–TSA), but also with the catalyst–substrate complex (TSA–C1–PNA). Since the exactness of the kinetic evaluation is not high enough, we have not calculated the different inhibitor constants.
Conclusions
The porogenic solvents have a prominent role in fabricating the well-defined 3D cavity in the polymer matrix. The catalytic properties of the polymer catalysts like substrate stereo-specificity, substrate shape-selectivity, substrate specificity and Michaelis–Menten behaviour are found to be dependent on the solvent composition of the amidolytic reaction medium. The polymer prepared in DMSO is catalytically more efficient and obeys Michaelis–Menten kinetics in 1:9 ACN–Tris HCl buffer, while that prepared in CHCl3 is hydrolytically active in 9:1 ACN–Tris HCl buffer solution. The polymer catalyst exhibits solvent memory in the amidolytic reactions as in the case of template rebinding. Binding to the anilide is evaluated from the Michaelis–Menten constant Km and binding to the TSA can be estimated from the competitive inhibition caused by the added TSA during the catalytic amidolysis.
Acknowledgements
The authors gratefully acknowledge the support from CSIR for JRF and SRF (Divya CSIR-SRF Mathew). The authors are also thankful to IIRBS-Mahatma Gandhi University for providing facilities for spectral analysis and School of Biosciences-Mahatma Gandhi University for providing facilities for incubation studies.
Disclosure statement
No potential conflict of interest was reported by the authors.
References
- Vlatakis G, Andersson LI, Mosbach K, et al. Drug assay using antibody mimics made by molecular imprinting. Nature. 1993;361:645–647.
- Chen L, Xu S, Li J. Recent advances in molecular imprinting technology: current status, challenges and highlighted applications. Chem Soc Rev. 2011;40:2922–2942.
- Haupt K, Mosbach K. Molecularly imprinted polymers and their use in biomimetic sensors. Chem Rev. 2000;100:2495–2504.
- Wulff G, Liu J. Design of biomimetic catalysts by molecular imprinting in synthetic polymers: the role of transition state stabilization. Acc Chem Res. 2012;45:239–247.
- Alexander C, Davidson L, Hayes W. Stereoselective reduction of menthone by molecularly imprinted polymers. Tetrahedron. 2003;59:2025–2057.
- Kawanami Y, Yunoki T, Nakamura A. Rationally designing active molecularly imprinted polymer toward a highly specific catalyst by using metal as an assembled pivot. J Mol Catal A Chem. 1999;145:107–110.
- Ohkubo K, Urata K, Hirota S. Homogeneous esterolytic catalysis of a polymer prepared by molecular imprinting of a transition state analogue. J Mol Catal. 1994;93:189–193.
- Wulff G, Gross T, Schonfeld R. Enzyme models based on molecularly imprinted polymers with strong esterase activity. Angew Chem Int Ed Engl. 1997;36:1962–1964.
- Kim JM, Ahn KD, Wulff G. Cholesterol esterase activity of a molecularly imprinted polymer. Macromol Chem Phys. 2001;202:1105–1108.
- Robinson KD, Mosbach K. Molecular imprinting of a transition state analogue leads to a polymer exhibiting esterolytic activity. J Chem Soc Chem Commun. 1989;28:969–970.
- Liu XC, Mosbach K. Catalysis of benzisoxazole isomerization by molecularly imprinted polymers. Macromol Rapid Commun. 1998;19:671–674.
- Carboni D, Flavin K, Resmini M, et al. The first example of molecularly imprinted nanogels with aldolase type I activity. Chemistry. 2008;14:7059–7065.
- Ohkubo K, Urata Y, Hirota S, et al. Catalytic activities of novel L-histidyl group-introduced polymers imprinted by a transition state analogue in the hydrolysis of amino acid esters. J Mol Catal. 1995;101:L111–L114.
- Lele BS, Kulkarni MG, Mashelkar RA. Molecularly imprinted polymer mimics of chymotrypsin: 2. Functional monomers and hydrolytic activity. React Funct Polym. 1999;40:215–229.
- Corman ME, Akgol S. Preparation of molecular imprinted hydrophobic polymeric nanoparticles having structural memories for lysozyme recognition. Artif Cells Nanomed Biotechnol. 2012;40:245–255.
- Akgonullu S, Yavuz H, Denizli A. Preparation of imprinted cryogel cartridge for chiral separation of L-phenylalanine. Artif Cells Nanomed Biotechnol. 2016;45:800–807.
- Gokturk I, Uzek R, Denizli A, et al. Synthesis of a specific monolithic column with artificial recognition sites for L-glutamic acid via cryo-crosslinking of imprinted nanoparticles. Artif Cells Nanomed Biotechnol. 2015;44:1133–1140.
- Ohkubo K, Urata Y, Yoshinaga K. Preparation and catalytic property of L-histidyl group-introduced, crosslinked poly(ethylene imine)s imprinted by a transition-state analogue of an esterolysis reaction. Polymer. 1994;35:5372–5374.
- Mathew D, Thomas B, Devaky KS. Amidase activity of phosphonate analogue imprinted chymotrypsin mimics in shape-selective, substrate-specific and enantioselective amidolysis of L-phenylalanine-p-nitroanilides. J Mol Catal A: Chem. 2016;415:65–73.
- Mathew D, Thomas B, Devaky KS. Phosphonate TSA-built macromatric polymer catalysts as chymotrypsin mimics for the amidolysis of amino acid p-nitroanilides:-Effect of the nature and extent of crosslinker on amidase activities. Polymer. 2017;111:285–296.
- Mathew D, Thomas B, Devaky KS. Amidase activity of phosphonate TSA-built polymer catalysts derived from organic monomers in the amidolysis of amino acid p-nitroanilides. Appl Catal A: Gen. 2016;528:93–103.
- Umpleby RJ, Rushton GT, Shah RN, et al. Recognition directed site-selective chemical modification of molecularly imprinted polymers. Macromolecules. 2001;34:8446–8452.
- Haupt K, Dzgoev A, Mosbach K. Assay system for the herbicide 2,4-dichlorophenoxy acetic acid using a molecularly imprinted polymer as an artificial recognition element. Anal Chem. 1998;70:628–631.
- Golker K, Nicholls IA. The effect of crosslinking density on molecularly imprinted polymer morphology and recognition. Eur Polym J. 2016;75:423–430.
- Ohkubo K, Sawakuma K, Sagawa T. Shape- and stereo-selective esterase activities of cross-linked polymers imprinted with a transition-state analogue for the hydrolysis of amino acid esters. J Mol Catal. 2001;165:1–7.
- Karmalkar RN, Kulkarni MG, Mashelkar RA. Molecularly imprinted hydrogels exhibit chymotrypsin-like activity. Macromolecules. 1996;29:1366–1368.
- Ohkubo K, Funakoshi Y, Sagawa T. Catalytic activity of a novel water-soluble cross-linked polymer imprinted by a transition-state analogue for the stereoselective hydrolysis of enantiomeric amino acid esters. Polymer. 1996;37:3993–3995.
- Emgenbroich M, Wulff G. A New Enzyme Model For Enantioselective Esterases Based On Molecularly Imprinted Polymers. Chem Eur J. 2003;9:4106–4117.