Abstract
There is a close relationship between autophagy and apoptosis during cancer cell death. We used chitosan nanoparticles (CS NPs) to explore the effects of internalized NPs on the induction of autophagy and to confirm the role of autophagic responses elicited by nanomaterials on the tumour cell’s fate. CS NPs at nontoxic concentrations ranging from 10–100 μg/mL triggered the induction of autophagy. With the addition of CS NPs, the aggregation of endogenous LC3 was significantly enhanced and acidic autophagic bodies had been accumulated. CS NPs significantly triggered the occurrence of autophagy by increasing the ratio of LC3 II to LC3 I and CS NPs-mediated autophagy was implicated in reactive oxygen species (ROS) generation and the ROS scavenger N-acetylcysteine (NAC) attenuated CS-induced autophagy. The addition of blank NPs produced a negative effect on cytotoxicity and cellular apoptosis of free Dox, and with the pre-treatment of chloroquine (CQ) as a known autophagy inhibitor, the inhibition rates of cells treated with the combination of Dox and blank CS NPs had been significantly increased. The findings demonstrated that CS NPs have the ability to induce protective autophagy via ROS generation and they were believed to inhibit tumour cell death.
Introduction
Chitosan nanoparticles (NPs) as potential drug delivery carriers have been widely studied in cancer treatment, as well as in the early diagnosis and detection of tumour biomarkers [Citation1–3] in vivo and in tumour-targeted therapy [Citation4–7]. CS as a carrier material is biodegradable, non-toxic and easily prepared when obtaining NPs featuring a suitable particle size, round shape and high dispersibility. Antitumour drugs were encapsulated in NPs and slowly released in a smart and controllable pattern. CS NPs were easily adsorbed at the cell surface, owing to their positive zeta potential, thus improving the internalization efficiency of the drug and the bioavailability. In the light of their smaller particle size, CS NPs can escape from newly formed tumour vessels, which are usually abnormal in form and architecture, around the tumour site and they have a higher tendency of accumulating within tumor tissues than they do in normal tissues [Citation8–10]. The intrinsic functional mechanism of NPs had garnered increasingly more attention. It was reported that some NPs could induce cell mitochondria-mediated autophagy [Citation11–14] and that they could also enhance the cytotoxicity of anti-cancer drugs [Citation15–18]. Epidermal growth factor receptor (EGFR)-targeted hybrid plasmonic magnetic NPs synergistically induced autophagy and apoptosis in non-small cell lung cancer [Citation5,Citation19]. On the other hand, the antineoplastic effects of some anti-cancer drugs were reduced by triggering NPs to induce so-called “protective autophagy”, [Citation20] and they further inhibited the cellular apoptosis pathway by removing damaged proteins and organelles within the cells [Citation21,Citation22]. Therefore, there is a possibility that NPs may inhibit or promote the growth of cells and regulate antitumor efficacy via NPs-mediated autophagy-induced apoptosis.
To investigate whether NPs triggered the occurrence of autophagy, and to confirm the positive or negative effects on antitumour efficacy between autophagy and apoptosis, CS NPs were prepared in this study by ionic crosslinking. The characterizations of NPs, such as their particle size, zeta potential, and polydispersity index (PDI), were determined. Cytotoxicities of free doxorubicin (Dox) and a mixture of free Dox and CS NPs were determined by 3-(4,5-dimethylthiazol-2-yl)-2, 5-diphenyltetrazolium bromide (MTT) assay and flow cytometry to evaluate whether the combination of Dox and NPs could significantly control cell viability and regulate the subsequent apoptotic effects. The cellular uptake of CS NPs was investigated to locate the NPs’ distribution using a fluorescence microscope. Furthermore, the level of proteins related to autophagy and apoptosis, ROS determination and cellular apoptosis were assessed to confirm the extent of protective autophagy that occurred, as induced by CS NPs, to reduce tumour cytotoxicity upon NP mediation.
Materials and methods
Materials
Doxorubicin hydrochloride was purchased from Beijing Huafeng Lianbo Technology Co., Ltd. (Beijing, PR China). CS, with a deacetylation degree of 80% and a molecular weight of about 400,000, was purchased from Haixin Biological Product Co., Ltd. (Zhejiang, PR China). N-acetylcysteine (NAC), 3-(4,5-dimethylthiazol-2-yl)-2, 5-diphenyltetrazolium bromide (MTT), trimeric sodium phosphate (TPP) and acetic acid were obtained from Sigma-Aldrich Co. (St Louis, MO). All of the other chemicals were of analytical grade and they were obtained from a variety of vendors. Hela cells and SMMC-7721 cells were obtained from Jinzhou Medical University (Liaoning, PR China).
Preparation and characterization of CS NPs
CS was selected as a biocompatible material in the preparation of NPs by an ionic crosslinking method. Accurately weighted (0.2 g) CS was dissolved in 500 ml of acetic acid (2%, v/v) and it showed a positive potential. TPP was also dissolved in distilled water and it showed a negative potential. With the continuous and quick dropping of 3 ml of TPP reserve liquid into the CS solution, the mixture demonstrated opalescence and was continuously stirred for 60 min at a controlled ambient temperature of 2–8 °C, indicating that blank CS NPs were formed by electrostatic interaction between the protonated amino groups of CS and the negatively charged groups from TPP. The average particle diameter, zeta potential and morphology were characterized using dynamic light scattering (DLS) (Nano ZS; Malvern Instruments, Malvern, UK) and transmission electron microscope (TEM) (JEM-1200EX; JEOL, Tokyo, Japan). Confocal laser scanning microscopy (FluoView FV10i; Olympus, Tokyo, Japan) was used to trace the internalization process of NPs within the cells.
Reactive oxygen species (ROS) production and measurement
CS NPs were incubated with the cells for certain amount of time, followed by continuous incubation with 10 μM of 2,7-dichlorofluorescein diacetate (DCFH-DA) (Sigma Aldrich Co.) for about 30 min. After washing with PBS, the intracellular DCF fluorescence intensity, which is excited at 485 nm and emitted at 530 nm, was detected using a microplate reader (Synergy-2; BioTek Instruments, Winooski, VT) and imaged by a confocal microscopy to investigate the extent of oxidative stress.
Green fluorescent protein-light chain 3 (GFP-LC3B) plasmid transfection
Hela cells and SMMC-7721 cells stably transfected with GFP-LC3B plasmid were seeded into 24-well cell culture plates for incubation for 24 h, and the number of cells in each well reached a density of 1 × 105/mL. Cells were treated with CS NPs at different concentrations for 24 h. Finally, these cells were washed and the GFP-LC3B was able to emit bright green fluorescence by confocal laser scanning microscope.
Acridine orange staining
After the Hela cells and SMMC-7721 cells were treated with the test groups, trypsin was used to digest the cells, and they were washed twice with phosphate buffered saline (PBS). Acridine orange solution was added into the medium and was incubated with the cells at a concentration of 2 μg/mL for 15 min, away from light, at 37 °C. The staining morphology was observed by detecting the fluorescence of acridine orange, which was excited at 488 nm and emitted at 640 nm.
Cell viability assay
Free Dox and the combination of Dox and blank NPs were chosen to treat the Hela and SMMC-7721 cells to evaluate cell viability using the MTT assay. Briefly, precisely 100 μL of prepared cell suspension was added into each well in a 96-well cell culture plate and reached a concentration of 1 × 105 cells/mL for 24 h at 37 °C under 5% CO2 and 95% O2. Test groups containing different concentration of Dox were added to each well, and following incubation for 48 h, 20 μL of MTT with a concentration of 5 μg/mL was added into a 96-well plate and incubated for 4 h at 37 °C. A microplate reader was used to detect the optical density (OD) of each well at 570 nm, and the half maximal inhibitory concentration (IC50) was also calculated.
Cell apoptosis evaluation by flow cytometry and western blot assay
Free Dox and the combination of Dox and blank NPs were chosen to treat Hela cells and SMMC-7721 cells. Annexin V–fluorescein isothiocyanate (FITC)/propidium iodide staining assay was performed, and the apoptotic and necrotic cells were quantified by flow cytometry. A western blot assay was performed to determine the level of apoptosis-related proteins, such as cleaved caspase-3 and PARP, while the expressions of Beclin1 and LC3 as autophagy-related proteins were also detected to evaluate the degree of autophagy. Briefly, the proteins were transferred to a membrane (typically, nitrocellulose or polyvinylidene fluoride (PVDF)), where they were stained with enhanced chemiluminescence (ECL). The levels of the targeted proteins were photographed and analysed using a UVP gel analysis system (iBox Scientia 600; UVP, LLC, CA).
Results
Preparation and characterization of CS NPs
As shown in , the CS NPs appeared to have a spherical shape and a smaller-than-average particle diameter of about 100.0 ± 6.7 nm. Due to their highly positive zeta potential of about 18.9 ± 5.6 mV, the CS NPs were easily adsorbed at the surface of the cells, thus improving the internalization efficiency of the drug, while also enhancing their bioavailability. The obtained NPs were dispersed homogeneously.
NPs distribution in the cells
To accurately observe the distribution of CS NPs in the cells, rhodamine B (RhB) and nuclear dye Hoechst 33342 were used to label the NPs and the nucleus, respectively. To prepare Rhodamine B-labeled CS NPs, 5 ml of stock solution (0.1 mg/ml) containing rhodamine B was added into the CS solution prior to adding the obtained TPP reserve liquid; the rest of the procedure was the same as that described earlier for CS NPs. From , it was found that the spontaneous red fluorescence emitted from rhodamine B loaded in CS NPs was primarily localized in the cytoplasm. With the continuous incubation of cells with the NPs, the red fluorescence intensity inside the cells was gradually increased, suggesting that CS NPs were focused within the cytoplasm, and with the passage of time, they were effectively internalized into the cells.
ROS production induced by CS NPs
2, 7-Dichlorofluorescin diacetate (DCFH-DA) probes was used to quantify NPs-induced intracellular ROS levels by using a fluorescence microplate reader and imaged by a confocal microscopy. The results in demonstrated that after being treated with different amounts of CS NPs, CS NPs enhanced ROS generation in Hela cells and SMMC-7721 cells, and a strong green fluorescence was observed in cells, indicating that with the mediation of NPs, a large amount of reactive oxygen species ROS was produced. ROS levels in Hela cells and SMMC-7721 cells exposed to CS NPs with a low concentration of 10.0 μg/ml for 24 h had increased to 156.7% and 149.8%, respectively, when compared with 103.4% and 105.4% for the untreated Hela and SMMC-7721 cells. Similarly, with the increasing amount of CS NPs, the ROS levels also continued to increase, and NPs accelerated the production of intracellular ROS in a dose-dependent manner. CS NPs with a high concentration of 100.0 μg/ml for 24 h significantly upregulated the ROS levels to 197.5% in Hela cells and to 191.3% in SMMC-7721 cells. In contrast, pre-treatment with the ROS inhibitor NAC markedly attenuated the increase in the generation of ROS in the two cell lines treated with CS NPs, the intensity of intracellular green fluorescence was weaken.
Figure 3. ROS generation in cells treated with different amount of NPs at different times. Results were expressed as means ± SD (n = 3). *p < .05, versus the ROS levels in untreated cells at 6 h; #p < .05, versus the ROS levels in untreated cells at 12 h; +p < .05, versus the ROS levels in untreated cells at 24 h. Scale bar: 50 μm.
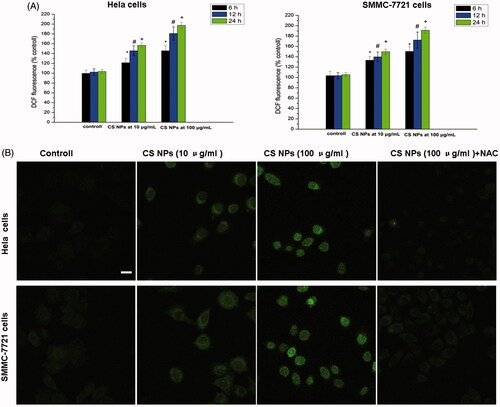
Confocal microscopic images of GFP-LC3B-transfected hela cells and SMMC-7721 cells treated with blank CS NPs
When autophagy occurred, LC3-I in the cytoplasm was modified to LC3-II [Citation23–25], which was positioned at the autophagic membranes. Thus, with the aid of transfecting a plasmid containing GFP-LC3B into the cells, intracellular LC3B protein aggregation was investigated by observing the GFP-LC3B proteins emitted by immunofluorescent double labelling. When autophagy occurred, GFP-LC3B was transferred to the autophagic membranes, where they aggregated, as represented by the gathering of bright green fluorescent dots. The results () showed that compared with the control group, CS NPs caused a significant green fluorescence intensity, indicating the aggregation of endogenous LC3B in both cell types. With the increasing amount of CS NPs, the green fluorescence intensity was also significantly enhanced. This indicated that CS NPs induced autophagy and showed a dose-dependent pattern, and thus, the aggregation of endogenous LC3B was significantly enhanced. After cells were treated with the combination of CS NPs and l0 mM oxygen free radical scavenger acetylcysteine (NAC), the level of autophagy was significantly reduced and the intensity of intracellular green fluorescence was weaken. It further proved that the internalization of NPs enhanced production of reactive oxygen species and was necessarily required for promoting cancer cell autophagy.
Acridine orange staining
An autophagosome was also observed by acridine orange staining fluorescence [Citation25]. As shown in , cells treated with CS NPs were shown in green, while red fluorescence represented the existence of acidic autophagic bodies. It can be clearly seen that both Hela cells and SMMC-7721 cells treated with different amounts of CS NPs showed an enhanced accumulation of red spots. This suggested that when compared with the control group, CS NPs triggered autophagy and enhanced the aggregation of autophagic bodies in the cells. The intensity and number of the red fluorescent spots were significantly higher than those of the untreated group, which demonstrated that the mediation of NPs accelerated the induction of autophagy and triggered the accumulation of acidic autophagic bodies. In order to verify the effect of ROS in NPs-induced autophagy, we incubated cells with the combination of CS NPs and NAC. The results indicated that pre-treatment with NAC could decrease the accumulation of acidic autophagic bodies, thus inhibiting NPs-induced autophagy.
Cell viability analysis
To investigate the impact of NPs on cell activity (viability), different test groups containing a combination of free Dox and CS NPs were treated with Hela and SMMC-7721 cells, and MTT was applied to examine cell viability. We found that in , the inhibition rates of blank CS NPs toward tumor cells were very low and that different amounts of blank CS NPs showed no obvious cell inhibition within 48 h. To further confirm whether the mediation of NPs triggered the higher or lower cytotoxicity of anticancer drugs, mixtures of free Dox and blank NPs were also incubated with the cells to evaluate their cytotoxicity. It was noted that when the same concentration of Dox was used, the addition of different doses of blank NPs reduced the cell inhibition rates induced by Dox. When compared with free Dox, a combination of Dox and blank NPs (100 μg/ml) induced the lowest inhibition effects with respect to cell viability at 48 h, and the IC50 value at 48 h was 11.4 μg/ml in Hela cells and 15.0 μg/ml in SMMC-7721 cells. Free Dox induced relatively higher inhibition effects in terms of cell viability at 48 h, and the IC50 value of free Dox at 48 h was 8.6 μg/mL in Hela cells and 10.2 μg/mL in SMMC-7721 cells. Interestingly, with the pre-treatment of chloroquine (CQ), a known inhibitor of autophagolysosomes formation, the inhibition rates of cells treated with the combination of free Dox and blank CS NPs were significantly increased. The IC50 value at 48 h was 9.6 μg/mL in Hela cells and 13.0 μg/ml in SMMC-7721 cells. This finding implied that the autophagy induced by NPs played a protective role in inhibiting the cytotoxicity of drugs, and NPs reduced apoptotic effects of Dox in tumour cells. The coaddition of CQ and the combination of free Dox and blank NPs enhanced cell apoptosis by downregulating autophagy, and it also significantly reduced cell viability.
Cell apoptosis and necrosis
To justify the role of NP-mediated autophagy on cell apoptosis, an Annexin V-FITC/PI staining assay was performed and the apoptotic and necrotic cells were quantified by flow cytometry. The results () showed that with the increasing addition of NPs, the apoptotic effects were significantly reduced in both cell types. Flow cytometry analysis revealed that after being treated with free Dox for 48 h, the ratio of positive staining cells in Hela and SMMC-7721 cells was 85.9% and 76.5%, respectively. Conversely, the combination of Dox and blank NPs induced relatively lower apoptotic effects in both cell types. Flow cytometry analysis revealed that after 48 h of treatment with free Dox and blank NPs, the ratio of positive staining cells in Hela cells and SMMC-7721 cells decreased to 65.5% and 58.9%, respectively. With the addition of more CS NPs, the ratio was further reduced to 47.7% and 37.9%, respectively, with the pre-mediation of CQ serving as an inhibitor to prevent autophagy by blocking autophagosome fusion and degradation. The ratio had been obviously increased. This proved that NP-triggered autophagy adversely affected apoptosis and that the blockade of autophagy increased the cells’ sensitivity to the apoptotic signals.
Western blot assay
To further clarify the relationship between NPs-induced autophagy and cell apoptosis, we treated cells with free Dox and with a combination of free Dox and CS NPs. The expression of cleaved caspase-3 and cleaved PARP were served as the main apoptosis-relevant proteins, while Beclin1 and LC3 were the main indicators of autophagy; these factors were also assessed in a western blot experiment. As evident in , when Hela cells were treated with blank CS NPs (by increasing the amount of NPs), the expression levels of cleaved caspase-3 and cleaved PARP were very low and demonstrated no obvious enhancement when compared with the control group. This finding indicated that in accordance with the MTT results, blank NPs at concentrations ranging from 10–100 μg/mL did not induce caspase-dependent apoptosis. Conversely, the ratio of LC3 II to LC3 I and Beclin1 had been significantly increased with the increasing amount of added CS NPs, implying that the autophagic vesicles in the cells had been accumulated within the cells; indicating that the CS NPs induced autophagy in a dose-dependent manner. The results also showed that when compared to the group treated with free Dox, the group treated with a combination of free Dox and CS NPs showed a dose-dependent occurrence of autophagy, which was triggered by upregulating the ratio of LC3 II to LC3 I. The combined treatment further effectively inhibited cell apoptosis by inducing lower expression levels of cleaved caspase-3 and cleaved PARP in Hela cells. The addition of CQ significantly inhibited the NPs-induced autophagy effects by reducing the ratio of LC3 II to LC3 I, and further increasing the sensitivity of cells to Dox; this also promoted cell apoptosis by increasing the expression of cleaved caspase-3 and cleaved PARP proteins.
Figure 8. Western blot analyses of the expression levels of the cleaved caspase-3, cleaved PARP, LC3, and Beclin1 proteins when Hela cells were treated with blank CS NPs, free Dox, and a combination of free Dox and CS NPs. NPs(L) refers to the blank chitosan NPs at 10 μg/mL; NPs(H) refers to the blank chitosan NPs at 100 μg/mL.
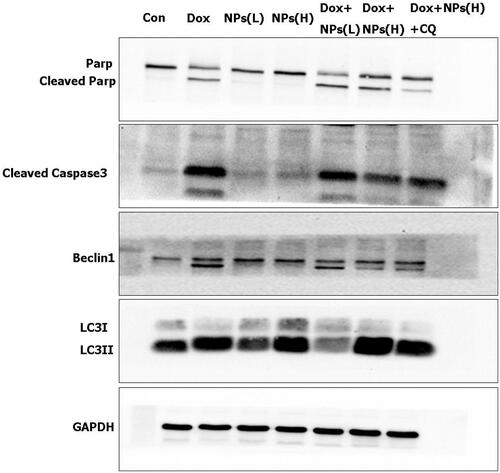
Discussion
Autophagy plays an important role in tumour development, survival and cell death. In some cases, such as when there is a lack of nutrients or the presence of an infection, autophagy is triggered and acts to degrade non-essential components within the cells, thus protecting the cells by responding to the source of danger [Citation26,27]. In our research, we found that CS NPs as a new potential autophagy initiator at nontoxic concentrations ranging from 10–100 μg/ml triggered the induction of autophagy and acted in regulating cellular apoptosis. With the addition of CS NPs, the aggregation of endogenous LC3 was significantly enhanced and acidic autophagic bodies had been accumulated. CS NPs significantly triggered the occurrence of autophagy by increasing the ratio of LC3 II to LC3 I. At the same time, treatments with CS NPs caused oxidative stress as demonstrated by increased intensity of the ROS indicator DCF and contributed to the induction of autophagy. Pre-treatment with N-acetylcysteine, an ROS scavenger, attenuated CS NPs-induced autophagy. To confirm the relationship between autophagy triggered by CS NPs and apoptosis during cancer cell death, we evaluated whether the combination of Dox and NPs could significantly control cell viability while regulating the cells’ apoptotic effects. It was found that the addition of blank NPs produced a negative effect on cytotoxicity and cellular apoptosis induced by free Dox; it depended on the dose of the blank NPs to reduce the cell inhibition rates. With the pre-treatment of CQ as a known inhibitor of autophagy, the inhibition rates of cells treated with the combination of free Dox and blank CS NPs had been significantly increased. This indicated that the autophagy induced by NPs played a protective role in inhibiting the drug’s cytotoxicity.
Conclusions
CS NPs were used to explore the effects of NPs on the induction of autophagy, as well as to determine their synergistic effects on tumour cell death. This investigation demonstrated that the internalization of CS NPs accelerated the accumulation of ROS and further led to the induction of ROS-mediated protective autophagy. This protective effect resulted in the obvious inhibition of tumour cell death induced by anticancer drugs.
Disclosure statement
The authors report no conflicts of interest in this work.
Additional information
Funding
References
- Mansur AA, Mansur HS, Soriano-Araújo A, et al. Fluorescent nanohybrids based on quantum dot-chitosan-antibody as potential cancer biomarkers. ACS Appl Mater Interfaces. 2014; 6:11403–11412.
- Sayari E, Dinarvand M, Amini M, et al. MUC1 aptamer conjugated to chitosan nanoparticles, an efficient targeted carrier designed for anticancer SN38 delivery. Int J Pharm. 2014; 473:304–315.
- Chen X, Ma Z. Multiplexed electrochemical immunoassay of biomarkers using chitosan nanocomposites. Biosens Bioelectron. 2014;55:343–349.
- Wang X, Tang H, Wang C, et al. Phenylboronic acid-mediated tumor targeting of chitosan nanoparticles. Theranostics. 2016;6:1378–1392.
- Nascimento AV, Gattacceca F, Singh A, et al. Biodistribution and pharmacokinetics of Mad2 siRNA-loaded EGFR-targeted chitosan nanoparticles in cisplatin sensitive and resistant lung cancer models. Nanomedicine (Lond). 2016;11:767–781.
- Corbet C, Ragelle H, Pourcelle V, et al. Delivery of siRNA targeting tumor metabolism using non-covalent PEGylated chitosan nanoparticles: identification of an optimal combination of ligand structure, linker and grafting method. J Control Release. 2016;223:53–63.
- Zarrin A, Sadighian S, Rostamizadeh K, et al. Design, preparation, and in vitro characterization of a trimodally-targeted nanomagnetic onco-theranostic system for cancer diagnosis and therapy. Int J Pharm. 2016;500:62–76.
- Hari K, Kumpati P. Chitosan tethered colloidal gold nanospheres for drug delivery applications. J Nanosci Nanotechnol. 2016;16:229–241.
- Assa F, Jafarizadeh-Malmiri H, Ajamein H, et al. Chitosan magnetic nanoparticles for drug delivery systems. Crit Rev Biotechnol. 2017;37:492–509.
- Abbas Y, Azzazy HM, Tammam S, et al. Development of an inhalable, stimuli-responsive particulate system for delivery to deep lung tissue. Colloids Surf B Biointerfaces. 2016;146:19–30.
- Wu YN, Yang LX, Shi XY, et al. The selective growth inhibition of oral cancer by iron core-gold shell nanoparticles through mitochondria-mediated autophagy. Biomaterials. 2011;32:4565–4573.
- Li X, Feng J, Zhang R, et al. Quaternized chitosan/alginate-Fe3O4 magnetic nanoparticles enhance the chemosensitization of multidrug-resistant gastric carcinoma by regulating cell autophagy activity in mice. J Biomed Nanotechnol. 2016;12:948–961.
- Yu KN, Chang SH, Park SJ, et al. Titanium dioxide nanoparticles induce endoplasmic reticulum stress-mediated autophagic cell death via mitochondria-associated endoplasmic reticulum membrane disruption in normal lung cells. PLoS One 2015;10:e0131208.
- Friedhuber AM, Chandolu V, Manchun S, et al. Nucleotropic doxorubicin nanoparticles decrease cancer cell viability, destroy mitochondria, induce autophagy and enhance tumor necrosis. J Pharm Pharmacol. 2015;67:68–77.
- Lu HY, Chang YJ, Fan NC, et al. Synergism through combination of chemotherapy and oxidative stress-induced autophagy in A549 lung cancer cells using redox-responsive nanohybrids: a new strategy for cancer therapy. Biomaterials. 2015;42:30–41.
- Ali A, Suhail M, Mathew S, et al. Nanomaterial induced immune responses and cytotoxicity. J Nanosci Nanotechnol. 2016;16:40–57.
- Wongrakpanich A, Mudunkotuwa IA, Geary SM, et al. Size-dependent cytotoxicity of copper oxide nanoparticles in lung epithelial cells. Environ Sci: Nano. 2016;3:365–374.
- Cui X, Liang T, Liu C, et al. Correlation of particle properties with cytotoxicity and cellular uptake of hydroxyapatite nanoparticles in human gastric cancer cells. Mater Sci Eng C Mater Biol Appl. 2016;67:453–460.
- Yokoyama T, Tam J, Kuroda S, et al. EGFR-targeted hybrid plasmonic magnetic nanoparticles synergistically induce autophagy and apoptosis in non-small cell lung cancer cells. PLoS One. 2011;6:e25507.
- Li Y, Wang S, Wang Z, et al. Cationic poly(amidoamine) dendrimers induced cyto-protective autophagy in hepatocellular carcinoma cells. Nanotechnology. 2014;25:365101.
- Stern ST, Adiseshaiah PP, Crist RM. Autophagy and lysosomal dysfunction as emerging mechanisms of nanomaterial toxicity. Part Fibre Toxicol. 2012;9:20.
- Zhang R, Piao MJ, Kim KC, et al. Endoplasmic reticulum stress signaling is involved in silver nanoparticles-induced apoptosis. Int J Biochem Cell Biol. 2012;44:224–232.
- Pizzurro GA, Tapia IJ, Sganga L, et al. Cytokine-enhanced maturation and migration to the lymph nodes of a human dying melanoma cell-loaded dendritic cell vaccine. Cancer Immunol Immunother. 2015;64:1393–1406.
- Zhang J, He Z, Xiao W, et al. Overexpression of BAG3 attenuates hypoxia-induced cardiomyocyte apoptosis by inducing autophagy. Cell Physiol Biochem. 2016;39:491–500.
- Ristic B, Bosnjak M, Arsikin K, et al. Idarubicin induces mTOR-dependent cytotoxic autophagy in leukemic cells. Exp Cell Res. 2014;326:90–102.
- Tan Q, Wang M, Yu M, et al. Role of autophagy as a survival mechanism for hypoxic cells in tumors. Neoplasia. 2016;18:347–355.
- Pla A, Pascual M, Guerri C. Autophagy constitutes a protective mechanism against ethanol toxicity in mouse astrocytes and neurons. PLoS One. 2016;11:e0153097.