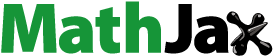
Abstract
The oral bioavailability and anti-leukaemia activity of Tanshinone IIA (TanIIA) were enhanced by using biotinylated-lipid bilayer coated mesoporous silica nanoparticles (Bio-LB-MSNs) as a vehicle. The in vitro release of TanIIA from TanIIA@MSNs was significantly higher than that of the TanIIA powder (p < .05). The in vitro cellular uptake of TanIIA by Caco-2 was increased by loading drug into the Bio-LB-MSNs more than those of the compared nanovehicles without biotin modification. The apparent in situ permeability coefficient (Papp) of TanIIA@Bio-LB-MSNs showed nearly 2.5-, 1.6- and 1.3-fold improvement compared with the TanIIA powder, TanIIA@MSNs and TanIIA@LB-MSNs. Following oral administration of TanIIA@Bio-LB-MSNs in rats, the area under the plasma concentration–time curves (AUC) of TanIIA was 3.4-, 1.9- and 2.4-fold larger than those in the groups received a pure TanIIA powder, TanIIA@MSNs or TanIIA@LB-MSNs, indicating that drug bioavailability was enhanced by using MSNs as a vehicle, and further improved significantly through biotin modification. The in vitro anti-leukaemia activity of TanIIA was also enhanced after being loaded into nanoparticles and modification, with 50% inhibitive concentration (IC50) of NB4 cells at 6.5 μM for TanIIA@Bio-LB-MSN. In conclusion, Bio-LB-MSNs are a promising vehicle to improve the oral bioavailability and anti-leukaemia activity of the poorly water-soluble drug TanIIA.
Introduction
Acute promyelocytic leukaemia (APL), an M3 type of acute myeloid leukaemia (AML), is featured by the reciprocal translocation of chromosomes 15 and 17, resulting in the generation of leukaemogenic PML-RAR fusion gene [Citation1]. Tanshinone IIA (TanIIA) (), one of the main active pharmaceutical ingredients (APIs) extracted from Salvia miltiorrhiza Bge (Danshen in Chinese) can induce the differentiation and apoptosis of NB4 cells (a human APL cell line) and has been used for the treatment of APL [Citation1,Citation2]. However, TanIIA is a typical biopharmaceutical classification system (BCS) Class II drug with a high Log p value (3.1) and a poor water solubility (2.8 ng·ml−1) [Citation3,Citation4]. Various approaches such as solid dispersion technologies and the use of colloidal carriers have been adopted to improve the solubility of TanIIA [Citation5–7]. Recently, mesoporous materials, classified as having pore diameters in the range from 2 to 50 nm by the International Union of Pure and Applied Chemistry (IUPAC) nomenclature, have been investigated as a vehicle to improve the oral bioavailability of poorly water-soluble drugs [Citation8,Citation9]. Mesoporous silica nanoparticles (MSNs), a novel mesoporous material [Citation10–12], have shown appealing characteristics as an amorphous drug stabilizer and drug carrier owing to their high pore volume and surface area, which contribute to their high drug-loading capacity [Citation13]. The nanometre-sized channels can efficiently confine the drug molecules within them and turn the drug from a crystalline form into a non-crystalline state, accounting for the increased drug solubility. A number of poorly water-soluble drugs, such as resveratrol, curcumin and griseofulvin, have been loaded into MSNs [Citation14–16]. Although MSNs are potential nanomedicine, the poor dispersion and inherently low biocompatibility of MSNs restrict their applications as drug delivery carriers [Citation17,Citation18]. To address the limitations, surface coating with lipid bilayers is proposed by exploiting the advantages of liposomes, such as low intrinsic toxicity and immunogenicity [Citation19]. Furthermore, the lipid bilayer may also be modified by conjugating with biotin, a targeting ligand [Citation20,Citation21]. Biotin, namely vitamin H or coenzyme R, is a vitamin that cannot be formed endogenously. The gastrointestinal uptake of biotin is by Na+-dependent and carrier-mediated endocytosis. Biotin receptors, which are distributed throughout the small intestine, are non-specific [Citation20]. Biotin-modified nanostructured lipid carriers and liposomes have been applied as carriers for the delivery of insulin and insoluble oridonin [Citation20,Citation21].
In this study, we synthesized MSNs, which were then coated with phospholipid membrane. The resulting lipid bilayer coated mesoporous silica nanoparticles (LB-MSNs) were then surface-modified with biotin. The performance of biotinylated-lipid bilayer coated mesoporous silica nanoparticles (Bio-LB-MSNs) as an oral vehicle for bioavailability enhancement and in vitro anti-leukaemia activity of TanIIA was studied.
Materials and methods
Materials
Tetraethyl orthosilicate (TEOS), cholesterol and cetyltrimethylammonium bromide (CTAB) were supplied by Sinopharm Chemical Reagent Co., Ltd. (Shanghai, China). DSPE-PEG2000 was obtained from Shanghai Advanced Vehicle Technology Co., Ltd. (Shanghai, China). Soybean lecithin (S75) was provided by Lipoid (Ludwigshafen, Germany). Biotin-DSPE-PEG2000 (Biotin molecules link DSPE-PEG2000 molecules with covalent bonding, the mass spectrogram is shown in Figure S1 in the supplementary materials) was purchased from Pengsheng Biological Co., Ltd. (Shanghai, China). TanIIA (purity 95%) was obtained from Shanghai Yuanye Biotech Co., Ltd. (Shanghai, China). Biotin, Fluorescent Hoechst 33258 and coumarin-6 (Cou-6) were purchased from Sigma-Aldrich (St. Louis, MO). Cell Counting Kit-8 (CCK-8) was obtained from Beyotime Biotechnology (Shanghai, China). Foetal bovine serum (FBS) was purchased from Gibco (Gibco, Carlsbad, CA). RPMI 1640 and Dulbecco’s modified Eagle’s medium (DMEM) were obtained from KeyGEN BioTECH Corp., Ltd. (Nanjing, China). Deionized water was generated using a Millipore Milli-Q system (Millipore, Billerica, MA).
Healthy male Sprague–Dawley (SD) rats with the weight from 180 to 200 g, registered under the license number SCXK2013–0016, were randomly allocated to different groups. The experiment was approved by the Animal Ethics Committee of Shanghai University of Traditional Chinese Medicine. Caco-2 cell line and human promyelocytic leukaemia NB4 cell line were purchased from KeyGEN BioTECH Corp., Ltd. (Nanjing, China).
Synthesis of MSNs, drug loading and lipid coating
Based on the previously published protocols, MSNs were synthesized with some modifications [Citation22,Citation23]. Briefly, NaOH (0.28 g) and CTAB (1 g) were sequentially dissolved in 480 ml of deionized water with magnetic stirring (600 rpm) at 80 °C for 30 min. Then, 5 ml of TEOS used as a silicon source was added dropwise within 10 min. After 2 h, the synthesized milk-white material was collected, washed first with 300 ml of water and then with 300 ml of ethanol, and dried at 45 °C in vacuum. The structure-templating CTAB was removed from the pores by dispersing the synthesized material in a mixture of ethanol (120 ml) and 11.9 M hydrochloric acid (15 ml). Then, the mixture was refluxed for 12 h twice in an acid ethanol solution. After that, the synthesized material was collected and washed in turn with water and ethanol. The final product was dried at 45 °C in vacuum to obtain MSNs. For drug loading, MSNs (36 mg) were soaked in 4 ml of TanIIA trichloromethane solution (15 mg·ml−1) and sonicated for 10 min. After stirring for 26.77 h, TanIIA-loaded mesoporous silica nanoparticles (TanIIA@MSNs) were centrifuged at 10,000 rpm. The final product was kept at 45 °C in vacuum to remove the residual solvent.
Cou-6 was used to label the nanovehicles for the cellular uptake study. For Cou-6 loading, 100 mg MSNs were soaked in 5 ml of Cou-6 trichloromethane solution (2 mg·ml−1) for 4 h at room temperature. Excess reagent was removed by centrifugation at 10,000 rpm for 10 min. The final product was kept at 45 °C in vacuum to remove the residual trichloromethane to get Cou-6 loaded MSNs (Cou-6@MSNs) for in vitro cellular uptake studies.
To form surface lipid bilayer coating, we developed a film dispersion-ultrasonic method with which the addition of MSNs suspensions to the lipid film surface was carried out [Citation24]. The lipid mix (DSPE-PEG2000 or Biotin-DSPE-PEG2000, S75 and cholesterol) was dissolved in trichloromethane. After solvent evaporation with a rotary evaporator at room temperature for 1 h, lipid film was formed. The lipid film was then placed in vacuum at 45 °C for 12 h to remove the residual solvent. At the time of coating, 4 ml of TanIIA@MSNs suspension was added drop by drop onto the surface of the lipid film. Then, the solution was processed by using the probe sonication with working cycle of 15/15 s on/off for 20 min. Since liposomes, coated MSNs and free drug existed in the suspension; the particles were centrifuged (10,000 rpm) and then washed three times in saline to get TanIIA-loaded lipid bilayer coated mesoporous silica nanoparticles (TanIIA@LB-MSNs) and TanIIA-loaded biotin modified lipid bilayer coated mesoporous silica nanoparticles (TanIIA@Bio-LB-MSNs). Biotin-modified liposomes were prepared by film dispersion-ultrasonic method described above.
A physical mixture (PM) was prepared as control, containing MSNs, lipid mix and pure TanIIA powder at the same ratio as in TanIIA@Bio-LB-MSNs. Cou-6-loaded lipid bilayer coated mesoporous silica nanoparticles (Cou-6@LB-MSNs) and Cou-6-loaded biotin modified lipid bilayer coated mesoporous silica nanoparticles (Cou-6@Bio-LB-MSNs) were also prepared by the method mentioned above. To determine the amount of TanIIA in TanIIA@LB-MSNs and TanIIA@Bio-LB-MSNs, TanIIA was extracted from nanoparticles using trichloromethane with sonication and then centrifuged (10,000 rpm, 10 min). The process was repeated three times to ensure the complete extraction. TanIIA in trichloromethane was quantified using HPLC. The drug loading was calculated using the following equation [Citation25]:
(1)
(1)
High-performance liquid chromatography assay
To detect TanIIA, a high-performance liquid chromatography (HPLC) system (LC-2010 A HT, Shimadzu, Japan) was used, with a Dikma Diamonsil Plus C18 column (4.6 × 250 mm, 5 μm) and a 75% (v/v) methanol aqueous solution as the eluent, with a flow rate of 1 ml·min−1 and the detection wavelength of 275 nm. The method was validated for intra-day, inter-day precision and the recovery, as shown in Table S1 in the supplementary materials, these were consistent with requirements of TanIIA.
Characterization of the particles
N2 adsorption–desorption isotherms
To confirm the porosity characteristics, N2 adsorption–desorption isotherms of MSNs and TanIIA@MSNs were measured using a TriStar II 3020 V1.02 instrument (Micromeritics Instrument Corp., Norcross, GA). The samples were outgassed at 300 °C for 3 h under nitrogen before the analysis. The pore size, pore volume and specific surface area of the silica nanoparticles were determined by nitrogen adsorption at 77 K, and adsorption–desorption isotherms were recorded. The surface areas were calculated using the Brunauer–Emmett–Teller (BET) procedure, the pore sizes were determined using the Barrett–Joyner–Halenda (BJH) method based on the adsorption isotherms while the total pore volume was assessed using the t-plot method of de Boer [Citation26–28].
Particle size and analysis of ζ-potential
Nano ZS90 Zetasizer (Malvern Instruments, Malvern, UK) was utilized to measure the Z-averaged hydrodynamic particle size (nm), polydispersity index (PDI) and ζ-potential (mV) of the nanoparticles. All the samples were dispersed in deionized water and sonicated before the analysis.
Morphological analysis
The morphology of TanIIA@Bio-LB-MSNs was characterized using a JEM-1400 TEM instrument (JEOL, Ltd., Tokyo, Japan). Prior to the examination, the sample was dispersed in deionized water and a drop of the resulting solution (20 μl) was deposited on a carbon-coated copper grid, followed by air-drying at room temperature for 30 min. Then, the sample was negatively stained with phosphotungstic acid (with pH between 6 and 7) for 30 s and subsequently visualized with TEM. The images presented are representative of the whole sample.
Fourier transform infrared spectroscopy (FTIR) analysis
FTIR spectra of the Bio-liposomes, TanIIA@MSNs and TanIIA@Bio-LB-MSNs were collected on an AVATAR 330 instrument (Thermo Scientific, Waltham, MA) using a KBr disk method. The FTIR spectra were obtained between 400 and 4000 cm−1 in a transmittance mode.
Differential scanning calorimetry (DSC) analysis
The pure TanIIA powder, physical mixture, TanIIA@MSNs, TanIIA@LB-MSNs and TanIIA@Bio-LB-MSNs was examined using a NETZSCH DSC 204 F1 DSC apparatus (NETZSCH–Gerätebau GmbH, Selb, Germany). The samples were run over a range of 25–300 °C in aluminium pans under a nitrogen flow and the heating rate was 10 °C per minute.
X-ray powder diffraction (XRD) analysis
The pure TanIIA powder, PM, TanIIA@MSNs, TanIIA@LB-MSNs and TanIIA@Bio-LB-MSNs was analysed using a D8 Advance X-ray diffractometer (Bruker, Karlsruhe, Germany) with Cu/Ka radiation (voltage, 40 kV; current, 30 mA). XRD patterns of the samples were recorded from an initial angle of 2θ = 3° to a final angle of 2θ = 45° at a scanning speed of 4° (2θ)·min−1.
Cell culture
The Caco-2 cells and NB4 cells were cultured in high glucose DMEM and RPMI-1640 medium supplemented with 10% FBS and 1% antibiotics (penicillin/streptomycin) and 1% non-essential amino-acid at 37 °C and 5% CO2, respectively. The cells were subcultured at 80–90% confluency.
In vitro cellular uptake studies
For confocal laser scanning microscopy (CLSM), the Caco-2 cells were seeded in confocal dishes at the density of 5 × 104 cells per well overnight prior to study. The Caco-2 cells were then incubated with fluorescent Cou-6@MSNs, Cou-6@LB-MSNs, Cou-6@Bio-LB-MSNs with Cou-6 concentration of 5 μg·ml−1 at 37 °C for 1.5 h, followed by rinsing with PBS, fixation with 4% paraformaldehyde for 20 min and staining of cell nuclei with Hoechst 33258. For the competitive receptor assay, cells were pre-incubated with biotin solution (100 μg·ml−1) for 1 h at 37 °C before the addition of nanoparticles. The cells, which were washed with PBS for three times, were viewed under Leica SP8 confocal laser scanning microscope (Leica Microsystems, Wetzlar, Germany).
For flow cytometry analysis, the Caco-2 cells were seeded in 6-well plates at the density of 5 × 105 cells per well overnight before the study. After the medium being removed, the Caco-2 cells were incubated with Cou-6@MSNs, Cou-6@LB-MSNs, Cou-6@Bio-LB-MSNs with Cou-6 concentration of 10 μg·ml−1 for 1.5 h at 37 °C.
To understand the cellular uptake mechanism, a receptor competitive experiment was carried out. The cells were preincubated with free biotin solution (100 μg·ml−1) for 1 h at 37 °C prior to addition of nanoparticles. Then, they were washed twice with PBS, detached with 0.25% trypsin, centrifuged, re-suspended in PBS and analysed by a FACSCanto II flow cytometer (BD Biosciences, San Jose, CA).
In vitro release
The in vitro release of the drug from the pure TanIIA powder and various drug-loaded nanovehicles was compared. The dissolution experiments were conducted with 1% sodium dodecyl sulphate (pH 6.8) as a release medium at 37 ± 0.5 °C and the paddle speed of 100 ± 1 rpm. Sodium dodecyl sulphate was added to maintain a sink condition. The tested formulations equivalent to 2 mg of TanIIA were put into to the dissolution medium. The release medium (1 ml) was withdrawn at predetermined intervals. An equivalent volume of fresh medium (37 °C) was added to maintain a constant release volume. All the samples were analysed using HPLC.
In situ single-pass intestinal perfusion (SPIP) study
To investigate the absorption and permeation behaviour of TanIIA in the pure TanIIA powder and various drug-loaded nanovehicles, in situ SPIP study was conducted according to the published literature [Citation29]. Before experiments, the animals were fasted for 12 h with free access to water and then were anaesthetized with 20% urethane aqueous solution (w/v) by intraperitoneal injection (0.6 ml per 100 g rat). The abdomens of rats were incised up to about 3 cm along the midline of abdomen to create inlet and outlet (approximately 10 cm in length) at the proximal part of the jejunum. After being equilibrated for 30 min with the Krebs–Ringer (K–R) solution, the perfusion fluid consisting of the different formulations (the pure TanIIA powder and various drug-loaded nanovehicles containing 2 μg·ml−1 TanIIA) was infused at the speed of 0.2 ml·min−1 into the selected intestinal segment. The perfusion fluid was withdrawn at predetermined intervals. All the samples were analysed using HPLC. The apparent in situ permeability coefficient (Papp) was calculated according to the following equation:
(2)
(2)
where Q is the infusion rate (0.2 ml·min−1), Qin and Qout are the volumes of perfusion fluid at inlet and outlet, respectively. Cin and Cout are the concentrations of the tested formulations at inlet and outlet, respectively. l (cm) and r (cm) are the length and effective radius of the selected intestinal segment.
In vivo pharmacokinetic studies in rats
Prior to oral drug administration, the rats were assigned to four formulation groups (n = 5): pure TanIIA powder, TanIIA@MSNs, TanIIA@LB-MSNs and TanIIA@Bio-LB-MSNs. Before experiments, the animals were fasted for 12 h with free access to water. Formulations at doses of 35 mg·kg−1 of TanIIA was administered to the rats, as a single dose using an intragastric gavage technique. After the administration of different formulations, blood samples were withdrawn from the retro-orbital plexus and then stored in heparinized tubes at predetermined time. To separate the plasma, the blood samples were centrifuged (Eppendorf, Hamburg, Germany) for 10 min. Before analysis, each plasma sample (200 μl) was mixed with 0.6 ml of acetonitrile, and the mixture was vortexed for 5 min and then centrifuged for 10 min. The supernatant was collected and the residue was extracted with the other 0.4 ml of acetonitrile. The supernatants were combined and dried under nitrogen. The resultant residue was dissolved in 200 μl of methanol, centrifuged at 12,000 rpm for 10 min before analysis with HPLC, as described above.
Cell viability assay
The Cell Counting Kit-8 (CCK-8) was utilized to investigate the cellular toxicity of TanIIA@MSNs, TanIIA@LB-MSNs and TanIIA@Bio-LB-MSNs against NB4 cells [Citation30]. In brief, approximately 10 × 104 cells per well were seeded in 96-well plates and set in an incubator for 24 h. Then, NB4 cells were treated by increasing concentrations of TanIIA, TanIIA@MSNs, TanIIA@LB-MSNs and TanIIA@Bio-LB-MSNs. After 24 h exposure, 10 μl CCK-8 was added and incubated with NB4 cells for another 4 h to quantity the living cells. At last, the absorbance of the 96-well plate was measured by using a microplate reader (Bio-Rad Laboratories, Hercules, CA) at 450 nm. The percentage of cell inhibition rate was calculated by the following equation:
(3)
(3)
where ODt is the mean absorbance of wells treated with the tested samples and ODc is the mean absorbance of untreated cells. The 50% inhibitive concentration (IC50) of NB4 cells was calculated by the CompuSyn software (ComboSyn, Inc., Paramus, NJ).
Data analysis
The pharmacokinetic parameters were analysed with the WinNolin version 6.2 software (Pharsight Corporation, Mountain View, CA). A multiple comparison test was carried out to analyse the differences between the groups by one-way analysis of variance using the SPSS version 19.0 software (SPSS Inc., Chicago, IL). The criterion for significance was p ≤ .05.
Results and discussion
Characterization of MSNs
TanIIA@Bio-LB-MSNs with drug loading of 5.3% were prepared. The particle size (), PDI and zeta potential measured by Nano ZS90 Zeta sizer are shown in . The PDI of TanIIA@Bio-LB-MSN and TanIIA@LB-MSN was lower than that of the non-coated MSNs, indicating that lipid bilayer coating could improve the dispersibility of MSNs. After lipid bilayer coating, the particle size of TanIIA@Bio-LB-MSN and TanIIA@LB-MSN became bigger than that of TanIIA@MSN. The TEM images of TanIIA@Bio-LB-MSNs () revealed that the nanovehicles were coated with uniform lipid bilayer, with thickness of about 7 nm. In addition, the particle size distribution in the TEM image showed smaller than that measured by dynamic light scattering (DLS) method, which may be because that the DLS method can hardly distinguish the aggregated particles and then exaggerate the mean particle size of the tested MSNs. Similar findings were reported by Jia et al. [Citation22]. FTIR spectra of the Bio-liposomes, TanIIA@MSNs and TanIIA@Bio-LB-MSNs, as illustrated in , verified the successful coating of lipid bilayer onto MSNs. Characteristic peaks at 1068 cm−1 due to asymmetric vibration of Si–O groups appeared in the FTIR spectrum of MSNs. The characteristic peaks of Bio-liposomes were present around 2920 and 1720 cm−1 which were consistent with the stretching vibrations of alkyl groups and ester groups, respectively [Citation31]. Similar peaks were identified in the Bio-LB-MSNs which confirmed lipid bilayer coating. The nitrogen adsorption–desorption isotherms of the MSNs and TanIIA@MSNs, shown in , with all the isotherms classified as type IV isotherms according to IUPAC, confirmed the pore structure observed by TEM. The shapes of hysteresis loops at high P/P0 have often been associated with specific pore structures [Citation32]. After drug loading, the BET surface, total pore volume, and BJH average pore diameter all decreased, as shown in , indicating the encapsulation of the drug.
Figure 2. Size distribution (a) and TEM images (b) of TanIIA@MSNs (A), TanIIA@LB-MSNs (B) and TanIIA@Bio-LB-MSNs (C). Scale bars for TEM images are 100 nm, 50 and 50 for A, B and C, respectively.
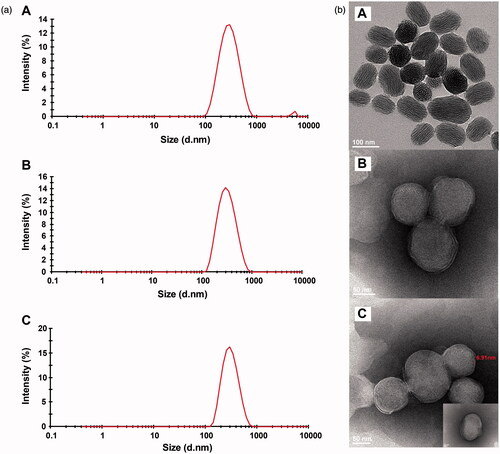
Table 1. Characterization concerning the size, polydispersity and zeta potential of TanIIA@MSNs, TanIIA@LB-MSNs and TanIIA@Bio-LB-MSNs.
Table 2. The nitrogen adsorption–desorption characteristic parameters of MSNs and TanIIA@MSNs.
DSC assay results are shown in . A single sharp endothermic melting peak was observed at 215.0 °C in the pure TanIIA powder and PM, which corresponded to the TanIIA melting point as reported by Yan et al. [Citation5]. However, no melting peaks of TanIIA were observed in the tested nanoparticles, indicating that the drug dispersed into the pores of nanoparticles was converted into an amorphous or non-crystalline state, which was in agreement with the XRD experiments, as shown in . Strong characteristic crystalline diffraction peaks were observed at 2θ = 7.2°, 2θ = 9.6° and 2θ = 12.0° in the XRD curves of the pure TanIIA powder. These characteristic diffraction peaks were also observed in the XRD curves of PM. In contrast, the crystalline diffraction peaks of TanIIA disappeared from the nanoparticle formulations, indicating that TanIIA existed in an amorphous or non-crystalline state.
In vitro cellular uptake studies
The result of CLSM in showed that the intensity of Cou-6 in Caco-2 cells is the strongest for the biotin modified nanoparticles, compared with unmodified and uncoated nanoparticles, suggesting the uptake of nanoparticles by Caco-2 cells was improved after biotin modification. The data of flow cytometry in further verified the result of focal microscopy. Compared with the unmodified or uncoated nanoparticles, biotin modified nanoparticles had significant higher (p < .05) mean fluorescence intensity (MFI).
Figure 6. Confocal laser scanning microscopy (CLSM) images (a) and Flow cytometry (b) of Caco-2 cells incubated with Cou-6@MSNs, Cou-6@LB-MSNs, Cou-6@Bio-LB-MSNs or free biotin solution pretreated Cou-6@Bio-LB-MSNs for 1.5 h at 37 °C. (Scale bars for CLSM images are 25 μm. From left to right, cell nuclei stained by Hoechst 33258 (blue), nanoparticles labelled by Cou-6 (green) and the merge of the two images. The cells without any treatment were used as control).
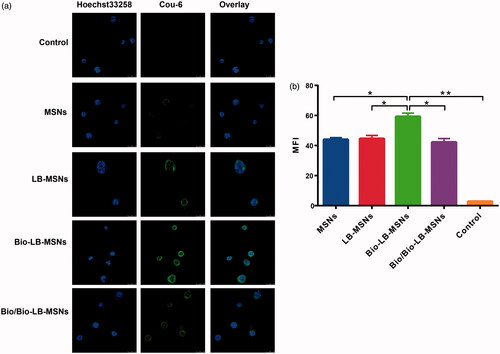
The effect of receptor competition was also studied. CLSM and flow cytometry indicated that the presence of excessive free biotin decreased the improved uptake of biotin-modified nanoparticles by Caco-2 cells. Biotin is a vitamin that cannot be formed endogenously. Reports have demonstrated that the gastrointestinal uptake of biotin is by Na+-dependent and carrier-mediated endocytosis [Citation20]. Biotin receptors, which are distributed throughout the small intestine, are non-specific. Biotin-modified nanostructured lipid carriers and liposomes have been applied as carriers for the delivery of insulin and insoluble oridonin [Citation20,Citation21]. Combined with the results, we considered that the biotin modification promoted the uptake of nanoparticles by Caco-2 cells through biotin receptor-mediated endocytosis.
In vitro release
As shown in , a burst release of TanIIA from TanIIA@MSNs occurred with 67.2% of the drug released in the first 1 h, and increased to approximately 91.3% at 8 h. The cumulative drug release of TanIIA from TanIIA@LB-MSNs and TanIIA@Bio-LB-MSNs was 82.9 and 88.2% at 8 h, respectively. The cumulative release percent of drug from all of the tested nanocarriers was significantly higher than those of the pure TanIIA powder at all-time points (p < .05). The enhanced solubility of TanIIA was also attributed to the TanIIA loaded into mesochannels of small size and to the amorphous form, as verified by the DSC and XRD experiments. As illustrated by the Ostwald–Freundlich equation, as the particle size decreases, the surface area increases [Citation33] and hence the saturated solubility also increases. For lipid-coated nanoparticles, the release of TanIIA from TanIIA@LB-MSNs and TanIIA@LB-MSNs was slower than that of TanIIA@MSNs, which may be due to the drug diffusion being hindered by the coated lipid [Citation34].
In situ single-pass intestinal perfusion (SPIP) study
As shown in , the Papp of TanIIA@Bio-LB-MSNs showed nearly 2.5-, 1.6- and 1.3-fold improvement compared with the TanIIA powder, TanIIA@MSNs and TanIIA@LB-MSNs. These results confirmed that the biotin modification promoted the absorption and permeation of nanoparticles through jejunum by biotin receptor-mediated endocytosis.
Table 3. The Papp of TanIIA solution, TanIIA@MSNs, TanIIA@LB-MSNs and TanIIA@Bio-LB-MSNs in jejunum (n = 3).
Pharmacokinetic studies following oral administration to rats
The bioavailability of BCS II drugs is generally dissolution rate-limited [Citation35]. Enhanced TanIIA dissolution was observed in the in vitro tests with TanIIA loaded into nanoparticles as an amorphous or non-crystalline form. Moreover, biotin modification promoted the uptake of nanoparticles. Therefore, improved bioavailability was expected for TanIIA@Bio-LB-MSNs. The plasma concentration–time curves obtained in rats after oral administration of the pure TanIIA powder, TanIIA@MSNs, TanIIA@LB-MSNs and TanIIA@Bio-LB-MSNs to rats are shown in . The pharmacokinetic parameters were calculated and are shown in . Significant improvement in the bioavailability of TanIIA was achieved with all the nanoparticles compared to that of the pure TanIIA powder, in the order: TanIIA@Bio-LB-MSNs>TanIIA@LB-MSNs≈TanIIA@MSNs. The maximum plasma drug concentration (Cmax) of TanIIA@Bio-LB-MSNs 9.6-, 3.6- and 3.6-fold compared with those of the TanIIA powder, TanIIA@MSNs and TanIIA@LB-MSNs, respectively. Following oral administration of TanIIA@Bio-LB-MSNs in rats, the area under the plasma concentration–time curves (AUC) was 3.4-, 1.9- and 2.4-fold larger than those in the groups received a TanIIA powder, TanIIA@MSNs or TanIIA@LB-MSNs. The data suggested that oral bioavailability of TanIIA was significantly improved after biotin modification. The increased rate (indicated by the shorter Tmax) and extent of absorption from all the MSNs nanoparticles could be due to the higher release rate of TanIIA compared with the dissolution rate of the TanIIA powder. The future significant improvement of TanIIA@Bio-LB-MSNs was attributed to biotin receptor-mediated endocytosis whereby the ligand-gifted carriers are more easily absorbed into the circulation system. The latter was certified by the cellular uptake studies, thus promoting drug absorption during gastrointestinal transit as reported in the literature [Citation20]. All the results confirm that TanIIA@Bio-LB-MSNs improve the dissolution rate and adsorption of TanIIA, thus improve the bioavailability of TanIIA.
Figure 8. Plasma concentration–time profiles of the pure TanIIA powder, TanIIA@MSNs, TanIIA@LB-MSNs and TanIIA@Bio-LB-MSNs following oral administration in SD rats at a dose of 35 mg/kg (n = 5).
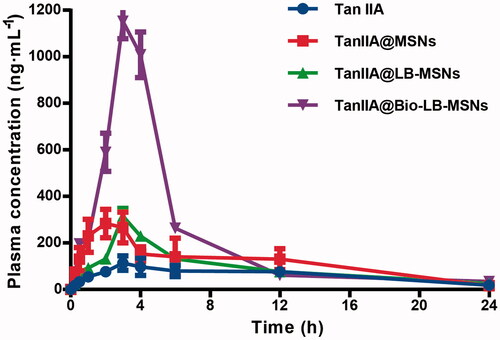
Table 4. Main pharmacokinetic parameters of the pure TanIIA powder, TanIIA@MSNs, TanIIA@LB-MSNs and TanIIA@Bio-LB-MSNs after oral administration to SD rats at a dose of 35 mg·kg−1 (n = 5).
In vitro anti-leukaemia activity of TanIIA@Bio-LB-MSNs
As shown in , a dose-dependent inhibition effect on NB4 cells was observed after 24 h incubation with TanIIA-loaded nanoparticles or pure TanIIA powder. The IC50 values for TanIIA powder, TanIIA@MSNs, TanIIA@LB-MSNs and TanIIA@Bio-LB-MSNs were 17.2, 7.7, 7.3 and 6.5 μM. The IC50 values of TanIIA@MSNs, TanIIA@LB-MSNs and TanIIA@Bio-LB-MSNs were significantly lower (p < .05) than that of the TanIIA powder. The results may be attributed to the fact that small molecular TanIIA dissolved in DMSO is passively diffused into cancer cells, while nanoparticles (TanIIA@MSNs, TanIIA@LB-MSNs and TanIIA@Bio-LB-MSNs) are transported into cancer cells by both diffusion and endocytosis process [Citation36]. Moreover, the biotin modification may improve the endocytosis process of nanoparticles by cancer cells [Citation37], which explains the lower IC50 values of TanIIA@Bio-LB-MSNs, compared with TanIIA@MSNs and TanIIA@LB-MSNs. As reported, TanIIA can induce APL cell apoptosis through the intrinsic pathway, which inhibits the MMP, leading to the increased release of cytosolic cyto-c, and the extrinsic apoptosis pathway by increasing TNF-α expression and caspase-8 activation. TanIIA can induce the differentiation of APL cells by increasing C/EBPβ expression [Citation2]. As a nano-carrier, according to our current work, the in vitro anti-leukaemia activity of TanIIA was improved after being loaded into nanoparticles and modification, which provided a promising approach for the treatment of APL.
Conclusions
In this study, we successfully synthesized bare MSNs, and loaded TanIIA to obtain TanIIA@MSNs by a solution–immersion method. TanIIA@MSNs were further coated with lipid bilayer on the surface to get TanIIA@LB-MSNs and TanIIA@Bio-LB-MSNs. The data shown that TanIIA in the nanoparticles was converted to amorphous thus significantly improved the dissolution rate of this BCS Class II drug. In vitro cellular uptake studies demonstrated that biotin modification improved the uptake of Bio-LB-MSNs by Caco-2 cells, which also contributed to the improvement of the oral bioavailability of TanIIA. The cytotoxicity of TanIIA@Bio-LB-MSNs against NB4 leukaemia cells was significantly enhanced, compared with the pure TanIIA powder. Therefore, Bio-LB-MSNs are a promising carrier for improving oral bioavailability and anti-leukaemia activity of the poorly water-soluble drug TanIIA.
Supplemental_File.docx
Download MS Word (516.3 KB)Disclosure statement
No potential conflict of interest was reported by the authors.
Additional information
Funding
References
- Wang L, Zhou GB, Liu P, et al. Dissection of mechanisms of Chinese medicinal formula Realgar-Indigo naturalis as an effective treatment for promyelocytic leukemia. Proc Natl Acad Sci USA. 2008;105:4826–4831.
- Zhang KJ, Li J, Meng WT, et al. Tanshinone IIA inhibits acute promyelocytic leukemia cell proliferation and induces their apoptosis in vivo. Blood Cells Mol Dis. 2016;56:46–52.
- Wang L. Study on absorption mechanism of lipophilic components of danshen and the method of enhance absorption. Chengdu: Sichuan University; 2006.
- Li SC, Liu JP, Zeng ZZ, et al. Study on the solubility and permeability of tanshinone II A and on the excipients increasing the solubility and permeability. Lishizhen Med Mater Med Res. 2008;19:1724–1726.
- Yan HM, Sun E, Cui L, et al. Improvement in oral bioavailability and dissolution of tanshinone IIA by preparation of solid dispersions with porous silica. J Pharm Pharmacol. 2015;67:1207–1214.
- Jiang YR, Zhang ZH, Huang SY, et al. Enhanced dissolution and stability of Tanshinone IIA base by solid dispersion system with nano-hydroxyapatite. Pharmacogn Mag. 2014;10:332–337.
- Li HL, Zhang ZY, Ma LL, et al. Preparation of tanshinone microemulsion and its absorption in rat intestine in situ. China J Chin Mater Med. 2007;32:1024–1027.
- Mellaerts R, Mols R, Jammaer JA, et al. Increasing the oral bioavailability of the poorly water soluble drug itraconazole with ordered mesoporous silica. Eur J Pharm Biopharm. 2008;69:223–230.
- McCarthy CA, Ahern RJ, Dontireddy R, et al. Mesoporous silica formulation strategies for drug dissolution enhancement: a review. Expert Opin Drug Del. 2016;13:93–108.
- Dement’EvaO V, Senchikhin IN, Kartseva ME, et al. A new method for loading mesoporous silica nanoparticles with drugs: sol-gel synthesis using drug micelles as a template. Colloid J. 2016;78:586–595.
- Sarkar A, Ghosh S, Chowdhury S, et al. Targeted delivery of quercetin loaded mesoporous silica nanoparticles to the breast cancer cells. Biochim Biophys Acta. 2016;1860:2065–2075.
- Varache M, Bezverkhyy I, Saviot L, et al. Optimization of MCM-41 type silica nanoparticles for biological applications: control of size and absence of aggregation and cell cytotoxicity. J Non-Ctryst Solids. 2015;408:87–97.
- Laine AL, Price D, Davis J, et al. Enhanced oral delivery of celecoxib via the development of a supersaturable amorphous formulation utilizing mesoporous silica and co-loaded HPMCAS. Int J Pharm. 2016;512:118–125.
- Summerlin N, Qu Z, Pujara N, et al. Colloidal mesoporous silica nanoparticles enhance the biological activity of resveratrol. Colloids Surf B Biointerfaces. 2016;144:1–7.
- Jambhrunkar S, Karmakar S, Popat A, et al. Mesoporous silica nanoparticles enhance the cytotoxicity of curcumin. RSC Adv. 2014;4:709–712.
- Jambhrunkar S, Qu Z, Popat A, et al. Modulating in vitro release and solubility of griseofulvin using functionalized mesoporous silica nanoparticles. J Colloid Interf Sci. 2014;434:218–225.
- van Schooneveld MM, Vucic E, Koole R, et al. Improved biocompatibility and pharmacokinetics of silica nanoparticles by means of a lipid coating: a multimodality investigation. Nano Lett. 2008;8:2517–2525.
- Wang LS, Wu LC, Lu SY, et al. Biofunctionalized phospholipid-capped mesoporous silica nanoshuttles for targeted drug delivery: improved water suspensibility and decreased nonspecific protein binding. ACS Nano. 2010;4:4371–4379.
- Butler KS, Durfee PN, Theron C, et al. Protocells: modular mesoporous silica nanoparticle-supported lipid bilayers for drug delivery. Small. 2016;12:2173–2185.
- Zhou XT, Zhang XW, Ye YH, et al. Nanostructured lipid carriers used for oral delivery of oridonin: an effect of ligand modification on absorption. Int J Pharm. 2015;479:391–398.
- Zhang XW, Qi JP, Lu Y, et al. Biotinylated liposomes as potential carriers for the oral delivery of insulin. Nanomedicine. 2014;10:167–176.
- Jia LJ, Shen JY, Li ZY, et al. Successfully tailoring the pore size of mesoporous silica nanoparticles: exploitation of delivery systems for poorly water-soluble drugs. Int J Pharm. 2012;439:81–91.
- He QJ, Shi JL, Chen F, et al. An anticancer drug delivery system based on surfactant-templated mesoporous silica nanoparticles. Biomaterials. 2010;31:3335–3346.
- Meng H, Wang M, Liu H, et al. Use of a lipid-coated mesoporous silica nanoparticle platform for synergistic gemcitabine and paclitaxel delivery to human pancreatic cancer in mice. ACS Nano. 2015;9:3540–3557.
- Fu Q, Hargrove D, Lu XL. Improving paclitaxel pharmacokinetics by using tumor-specific mesoporous silica nanoparticles with intraperitoneal delivery. Nanomedicine. 2016;12:1951–1959.
- Yefimova SL, Bespalova II, Grygorova GV, et al. Synthesis and characterization of mesoporous CaCO3@PSS microspheres as a depot system for sustained Methylene Blue delivering. Microporous Mesoporous Mater. 2016;236:120–128.
- Barrett EP, Joyner LG, Halenda PP. The determination of pore volume and area distributions in porous substances. i. computations from nitrogen isotherms. J Am Chem Soc. 1951;73:373–380.
- Lippens BC, de BJH. Studies on pore systems in catalysts: V. The t method. J Catal. 1965;4:319–323.
- Khurana RK, Beg S, Burrow AJ, et al. Enhancing biopharmaceutical performance of an anticancer drug by long chain PUFA based self-nanoemulsifying lipidic nanomicellar system. Eur J Pharm Biopharm. 2017;121:42–60.
- Zhang Z, Wang F, Du C, et al. BRM/SMARCA2 promotes the proliferation and chemoresistance of pancreatic cancer cells by targeting JAK2/STAT3 signaling. Cancer Lett. 2017;402:213–224.
- Mudakavi RJ, Raichur AM, Chakravortty D. Lipid coated mesoporous silica nanoparticles as an oral delivery system for targeting and treatment of intravacuolar Salmonella infections. RSC Adv. 2014;4:61160–61166.
- Thommes M, Kaneko K, Neimark AV, et al. Physisorption of gases, with special reference to the evaluation of surface area and pore size distribution (IUPAC Technical Report). Pure Appl Chem. 2015;87:1051–1069.
- Horkovics-Kovats S. Dissolution and coarsening of polydisperse, polymorph drug particles liberated from a disintegrating finished dosage form:theoretical considerations. Eur J Pharm Sci. 2016;91:265–277.
- Han N, Wang Y, Bai JL, et al. Facile synthesis of the lipid bilayer coated mesoporous silica nanocomposites and their application in drug delivery. Microporous Mesoporous Mater. 2016;219:209–218.
- Lipinski C. Poor aqueous solubility-an industry wide problem in drug discovery. Am Pharm Rev. 2002;5:82–85.
- Sengel-Turk CT, Hascicek C, Dogan AL, et al. Surface modification and evaluation of PLGA nanoparticles: the effects on cellular uptake and cell proliferation on the HT-29 cell line. J Drug Deliv Sci Tec. 2014;24:166–172.
- Urama Ł, Szuster M, Filipowicz A, et al. Cellular uptake of glucoheptoamidated poly(amidoamine) PAMAM G3dendrimer with amide-conjugated biotin, a potential carrier of anticancer drugs. Bioorg Med Chem. 2017;25:706–713.