Abstract
Carvacrol is a monoterpenoid flavonoid found abundantly in thyme plants. Its physiochemical instability and partial solubility in water is the principal limitation for its industrial use. Hence, we made a carvacrol nanoemulsion (CANE) using ultrasonication method and characterized it by dynamic light scattering (DLS) technique which revealed a negative surface charge (−29.89 mV) with 99.1 nm average droplet size. CANE effectively induced apoptosis in doxorubicin-resistant A549 lung carcinoma cells (A549DR) evident by the elevated expression of apoptotic proteins such as Bax, Cytochrome C, and Cleaved caspase 3 and 9. Also, CANE displayed cell senescence leading to cell cycle arrest by reducing CDK2, CDK4, CDK6, Cyclin E, Cyclin D1 and enhancing p21 protein expression. In addition, a potential role of CANE in the inhibition of autophagy was noted by evaluating the reduced conversion of LC-3 I to II. Beside this, a down-regulation of important autophagy markers ATG5 and ATG7 and upregulation of p62 were detected in response to CANE. We conclude that the synthesized CANE has potential to cause cell senescence, cell cycle arrest, autophagy inhibition and apoptosis in A549DR cells and could be used as a potential candidate for lung cancer therapy.
Introduction
In last more than 50 years, the occurrence of lung cancer has increased dramatically all over the world with high mortality rate [Citation1]. Treatment for lung cancer varies from radiotherapy, chemotherapy and surgery, which highly depends on the disease state and patients conditions. Although, chemotherapy of a single drug or in combination is the most frequently used methodology to cure the lung cancer. In the recent years, many incidences of chemotherapy resistance emerged as a major obstacle for the effective treatment of lung cancer [Citation2], which primarily caused by multidrug resistance (MDR) or cross-resistance for drugs. There are several molecular features accountable for irresponsive of a drug against MDR cells, one such factor is the overexpression of P-glycoprotein (P-gp) leads to drug efflux resulting in inhibition of apoptosis [Citation2]. The P-gp-related resistance was first observed and confirmed by Juliano et al. [Citation3]. Natural compounds pose a new hope to prevent cancer, due to their diverse structure and distinct mode of action. The promising character of many plant-derived compounds to prevent cell proliferation, angiogenesis, metastasis and tumour formation, has been well established [Citation4,Citation5].
Nutraceuticals known to control cancer formation by various molecular pathways such as cell cycle arrest, apoptosis, necrosis and autophagy [Citation4,Citation5]. However, several natural bioactive compounds possess limitation such as gastric residence time, instable to gastric pH and enzymes and directly effect on the efficacy of the compound [Citation6]. Therefore, it is important to enhance the self-life of these bioactive compounds by using different oil base, surfactants or linker [Citation7]. Here, we used carvacrol, a monoterpenoid phenolic compound found in various aromatic plants such as Origanum vulgare, Origanum dictammus, Origanum majorana, Thymbra capitate, Satureja hortensis, Thymus vulgaris and Thymus zygis, Thymus serpyllum, and Satureja montana which possess many biological activities such as antibacterial, antioxidant, anti-viral and anticancer [Citation8–10]. Carvacrol partial solubility in the water and instability towards light, pressure and heat are the major hurdles of its commercial application. To overcome these drawbacks, it is necessary to use carvacrol in its altered form, and emulsion proposed one of the convenient and effective ways to overcome these challenges by enhancing its self-life against the various physiochemical stresses [Citation7].
Cancer is one of the most lethal diseases of the current era with high mortality rates [Citation11]. During treatment of cancer, drug resistance can be acquired via direct suppression of apoptosis due to increased expression of anti-apoptotic proteins, such as B-cell lymphoma 2 (Bcl2) [Citation11]. Doxorubicin (DOX) is a chemotherapeutic agent from the anthracyclines, which are widely used in cancer chemotherapy [Citation12]. However, treatment of advanced non-small cell lung cancers (NSCLCs) with DOX provides an overall response rate of only 30–50% [Citation13]. Most patients with lung carcinoma developed resistance to DOX. Therefore, it is necessary to overcome the resistant mechanism of cell to make them vulnerable against anticancer drugs. Anticancer role of carvacrol has been well cited in the literature, however, its role against drug resistant cells has not been revealed yet. Hence, the present study was designed to prepare carvacrol nanoemulsion (CANE) and further evaluation of its potential role against doxorubicin resistant A549 lung carcinoma cells (A549DR).
Materials and methods
Chemicals, reagents and antibodies
All the reagents used, if not otherwise mentioned, were of the analytical grade purchased from Sigma–Aldrich (St. Louis, MO). Carvacrol, 3-Methyladenine (3-MA), N-acetyl-l-cysteine (NAC), primary antibodies against β-actin, JNK, p-JNK, caspase-3, caspase-9, Bax, Bcl2, cytochrome C, CDK2, CDK4, CDK6, Cyclin E, Cyclin D1, p21, LC-3, ATG5, ATG7, mTOR, p62, Beclin-1 as well as horseradish peroxidase (HRP)-conjugated secondary antibody were purchased from Santa Cruz Biotechnology, Inc. (Santa Cruz, CA).
Cell culture and development of drug resistant cells
A549DR, a human lung adenocarcinoma, and normal bronchial epithelium cells (BEAS-2B) were purchased from the American Type Culture Collection (Manassas, VA), and cultured in RPMI-1640 (Invitrogen, Carlsbad, CA) media, supplemented with 10% (v/v) fetal calf serum (Invitrogen, Carlsbad, CA) and 1% penicillin–streptomycin cocktail at 37 °C in 5% CO2 incubator.
The drug-resistant A549 cell line was developed in our laboratory using established protocols. In brief, the A549 cells were grown in 25 cm2 flask in the presence of 0.1 μM doxorubicin (Dox) until the surviving cells grown to an obvious confluency. After the completion of 3 cycles, the drug concentration was doubled and a procedure was repeated up to 25 μM concentration of drug (Dox). Finally, the drug resistant cells were maintained at 25 μM of doxorubicin and expressed as A549DR. For experiments, A549DR cells were maintained at 80–90% confluency (1 × 105 cells/well) and further treated with different concentrations of the prepared nanoemulsion.
Preparation of carvacrol nanoemulsion
Oil in water (O/W) carvacrol nanoemulsion (NE) was prepared by adding carvacrol (1.5%), medium chain triglyceride (MCT), lecithin and non-ionic surfactant and emulsifier polysorbate 80 in water under constant shaking conditions. NE was prepared in an aqueous medium by adding carvacrol with MCT, lecithin and polysorbate 80 in the weight ratio (1:1:0.01:2) and vortexed vigorously up to 5 min. Finally, sonicated at 25 khz in a sonicator (Ultrasonic Processor, GEX 750, Newtown, CT) with 750 W for 10 min. Tubes were kept in an ice bucket throughout the sonication process to neutralize the deleterious effect of heat generated during sonication.
Characterization of nanoemulsion
Droplet size, polydispersity index (PDI) and zeta potential of the formed CANE were analyzed by dynamic light scattering (DLS) analysis using a zeta-potential and particle size analyzer (ELSZ-2000, Otsuka Electronics Co., Ltd. Japan). The mean droplet size of CANE was determined by using software generated intensity, volume and number of distributions. The morphology of CANE was determined by scanning electron microscope (SEM) (S-4300, Hitachi High Technologies America, Schaumburg, IL).
Cytotoxic and morphology evaluation
The cytotoxic effect of CANE was first determined by MTT assay [Citation14]. Briefly, 5 × 104 A549DR cells/well were incubated in the presence of various concentrations of CANE (5–100 μg/ml) at 37 °C in a CO2 incubator for 24 h. After incubation, cells were treated with MTT solution (5 mg/ml) to produce dark blue colored formazan crystals, which were dissolved in 50 μl of DMSO. Finally, absorbance (540 nm) was measured using microplate reader (Bio-Tek instrument Co., WA).
Cytotoxicity was further confirmed by the lactate dehydrogenase (LDH) assay. Briefly, cells (5 × 104 cells/well) were treated with various concentrations of CANE (5–100 μg/ml) for 24 h at 37 °C. After incubation, media were removed and processed to evaluate extracellular LDH release using an LDH detection kit (Sigma–Aldrich, St. Louis, MO) as per the manufacturer’s guidelines. Cytotoxicity was evaluated by calculating the absorbance at 490 and 690 nm.
In addition, microscopic examination was done to determine morphological changes in A549DR cells after exposure to CANE (5, 25 and 50 μg/ml) using an inverted microscope (Nikon Eclipse TS200, Nikon Corp., Tokyo, Japan).
For agarose colony-forming assays briefly, cells (A549DR) were suspended in 1 ml of RPMI medium containing a 0.3% agarose and plated in triplicate on a firm 0.6% agarose base in 6-well plates (3 × 103 cells/well) with varying concentrations of CANE (5, 25 and 50 μg/ml). Cells were allowed to grow for 2 weeks at 37 °C in 5% CO2 incubator. Finally, colonies with a diameter greater than 20 μm were counted under the inverted microscope (Nikon Eclipse TS200, Nikon Corp., Tokyo, Japan) [Citation15].
Clonogenic assay
A total of 5 × 104 cells/well were seeded in a 12-well culture plate and allowed to attach for a period of 24 h, followed by the treatment of CANE (5, 25 and 50 μg/ml). After seven days incubation at 37 °C in a CO2 incubator, cells were washed with PBS and stained with 0.5% crystal violet [Citation16].
Senescence-associated β-galactosidase activity (SA-β-gal)
Cells were seeded in a 6-well culture plate (5 × 104 cells/well) and allowed to attach for a period of 24 h, followed by the treatment of CANE (5, 25 and 50 μg/ml). Furthermore, senescence-associated β-galactosidase (SA-β-gal) activity was determined by using a cellular senescence assay kit (Millipore; KAA002) as per the manufacturer’s instructions [Citation17].
Fluorescence staining
Apoptotic and live-dead morphological evaluation
Nuclear fragmentation as a marker of apoptosis was evaluated by Hoechst–propidium iodide staining. Briefly, cells (5 × 104 cells/well) were seeded in a 24-well-plate and allowed to attach for 24 h, followed by CANE (5, 25 and 50 μg/ml) treatment for an additional 24 h. After incubation, cells were stained with Hoechst–propidium iodide (1 µg/ml), and images were captured under an EPI fluorescence microscope (Nikon, Japan). Apoptotic cells were calculated as percent apoptotic nuclei compared with a total number of cells [Citation14].
Oxidative stress staining
Intracellular reactive oxygen species (ROS) production in response to CANE was determined using H2DCFDA fluorescent stain. Briefly, cells (5 × 104 cells/well) were seeded in a 24-well culture plate and allowed to attach for 24 h, followed by CANE (5, 25 and 50 μg/ml) treatment. After 24 h of treatment, cells were washed with PBS and subsequently incubated with 20 µM H2DCFDA dye for 30 min [Citation18]. Cells were washed twice with PBS, and ROS formation was immediately detected using an EPI fluorescence microscope (Nikon, Japan). Fluorescent intensity was quantified by using the Image-J software.
The potential effect of CANE on the production of mitochondrial superoxide was evaluated by staining the cells with MitoSOX Red, a mitochondrial superoxide indicator. Briefly, attached cells were treated with different concentrations of CANE (5, 25 and 50 μg/ml) for 24 h. After incubation, cells were washed twice with PBS and stained with 5 μM MitoSOX Red for 10 min. Finally, fluorescent images were captured using an EPI fluorescence microscope (Nikon, Japan). Mean fluorescence intensity was quantified using the Image-J software [Citation19].
Detection and quantification of autophagic vacuole formation
Briefly, cells (1 × 104 cells/well) were seeded in 24 well plates and allowed to adhere for overnight. After treatment with different concentrations of CANE (5, 25 and 50 μg/ml) for 24 h, autophagic vacuoles were detected with the help of acridine orange stain (100 μg/ml). Images were captured under the fluorescent microscope at 40× magnification. Moreover, the lysosome activity was evaluated by LysoTracker® Red autophagy detection kit (Enzo Life Sciences, Plymouth Meeting, PA) according to the manufacturer’s instruction. DAPI was used as a counter stain for nucleus; images were captured under the fluorescent microscope at 40× magnification. Mean fluorescence intensity was quantified using the Image-J software [Citation20].
Mitochondrial membrane potential (Δψm)
As mentioned above, A549DR cells were grown in a 24-well culture plate and treated with various concentrations of CANE (5, 25 and 50 µg/ml). After 24 h of incubation, cells were washed with PBS and stained with Rhodamine-123 (1 μg/ml) for 30 min. Finally, fluorescent images were analyzed under an EPI fluorescence microscope (Nikon, Japan) [Citation19].
Western blotting
The A549DR cells were cultured in a 3 ml culture dish followed by the treatment of CANE (5, 25 and 50 µg/ml) for 24 h. Furthermore, cells were washed with PBS and lysed using RIPA lysis buffer, and centrifuged at 12,000 rpm for 15 min at 4 °C. The obtained supernatant was used for Western blotting after protein quantification. Protein sample (25 μg) was loaded onto 12% SDS-PAGE and further transferred onto Polyvinylidene fluoride (PVDF) membranes. Next, 5% skim milk used for membrane blocking and incubated with specific antibodies overnight at 4 °C and further visualized using enhanced chemiluminescence Western blotting detection reagents (Amersham Biosciences Inc., Piscataway, N.J.).
In vivo animal studies
Antitumour potential of CANE was evaluated by inducing tumour in 4 week old male nude mice by injecting A549DR cells (1 × 106) subcutaneously. Once tumour reached 50 mm3 in size, mice were randomly divided into three groups (four mice in each group). Group I served as control (without treatment of CANE), while group II and III were given a treatment of CANE at 50 and 100 mg/kg body weight (BW), respectively. Treatment of CANE was given intraperitoneally (I.P) three times in a week up to one month. Body weight, tumour volume was calculated every week in all the three groups. Tumour volume was calculated by the equation adopted by Rong et al. 2017 [Citation21]. After one month, mice were sacrificed and the tumour was removed and process for Western blotting.
Statistical analysis
All experiments were carried out in triplicates. The results were expressed as the mean ± standard deviation (SD) of three independent experiments. Multiple comparisons were performed using a one-way ANOVA followed by Duncan test for post hoc analysis using the SPSS16 software. p values <.05 were considered statistically significant.
Results
Characterization carvacrol nanoemulsion
The formulated CANE was analyzed with the help of dynamic light scattering (DLS) technique in order to determine the average droplet size, surface charge and polydispersity index (PDI) (). The average droplet size, PDI and net surface charge in the formulated nanoemulsion were found to be 99.1 nm, 0.147 and −29.89 mV, respectively. To validate the size and morphology of CANE, SEM examination was carried out which revealed a spherical shape with 112.5 nm average droplet size (). The size obtained from the SEM is nearly similar to the size obtained from the DLS analysis.
CANE promotes dose-dependent cytotoxicity in A549DR cells
The cytotoxic potential was primarily evaluated by the MTT and LDH assay which revealed a dose-dependent cytotoxicity of CANE against A549DR cells (). The MTT assay revealed 52.5 μg/ml of CANE, as fifty percent inhibitory concentration (IC50) against A549DR cells. On the other side, CANE did not display any cytotoxic effect up to 50 μg/ml, against normal bronchial epithelial cells (). Results of the microscopic examination revealed distorted and shrunken cell morphology in the CANE-treated cells, unlike to control group where regular and smooth morphology occur (). The cytotoxicity was further confirmed by the clonogenic and soft agar assay which revealed a diminished colony formation in a dose-dependent manner after treatment with CANE (). All the results collectively suggest the cytotoxic potential of CANE against A549DR cells.
Figure 2. Cytotoxic potential of CANE against A549DR cells. (A) and (B) MTT and LDH assay, respectively, of CANE (5–100 μg/ml)-treated A549DR cells after 24 h of incubation. Emulsion without carvacrol was also used as a control. (C) MTT assay of CANE (25–100 μg/ml)-treated normal bronchial epithelium cells (BEAS-2B). (D) Morphological changes in A549DR cells after treatment with CANE (25–100 μg/ml). Images were captured at 40× magnification [scale bar= 0.1 mm]. (E) Clonogenic assay, A549DR cells were cultured in the presence and absence of CANE over 7 days, followed by crystal violet staining. (F) Soft agar colony assays showed that CANE treatment led to a significant decrease in anchorage-independent colony-forming ability of A549DR cells. (G) The ABCB, MDR protein found to be overexpressed in prepared DR cells. Densitometry analysis was determined by Image J software.
![Figure 2. Cytotoxic potential of CANE against A549DR cells. (A) and (B) MTT and LDH assay, respectively, of CANE (5–100 μg/ml)-treated A549DR cells after 24 h of incubation. Emulsion without carvacrol was also used as a control. (C) MTT assay of CANE (25–100 μg/ml)-treated normal bronchial epithelium cells (BEAS-2B). (D) Morphological changes in A549DR cells after treatment with CANE (25–100 μg/ml). Images were captured at 40× magnification [scale bar= 0.1 mm]. (E) Clonogenic assay, A549DR cells were cultured in the presence and absence of CANE over 7 days, followed by crystal violet staining. (F) Soft agar colony assays showed that CANE treatment led to a significant decrease in anchorage-independent colony-forming ability of A549DR cells. (G) The ABCB, MDR protein found to be overexpressed in prepared DR cells. Densitometry analysis was determined by Image J software.](/cms/asset/76d6b0bd-5b45-4395-85f1-f878ca892584/ianb_a_1434187_f0002_c.jpg)
To evaluate whether the cells (A549) have become the drug-resistant or not, the expression of ABCB protein was determined by Western blotting, which revealed higher expression in doxorubicin-treated A549 cells as compared to wild-type cells that confirmed the drug resistance nature of A549 cells ().
CANE mediates apoptotic morphology and oxidative stress in A549DR cells
The apoptotic potential of CANE was evaluated by Hoechst–propidium iodide staining which revealed a dose-dependent increased in apoptotic nuclei formation in the CANE treated cells as compared to control (). A significantly (p < .05) 6.5 times higher apoptotic nuclei were observed at 50 μg/ml CANE-treated cells as compared to control. Furthermore, results obtained from H2DCFDA and Mito-SOX staining indicate a dose-dependent enhancement in the cellular and mitochondrial ROS level after treatment with CANE (). A significantly 2.9 and 3.8-fold higher expression of cytosolic and mitochondrial ROS, respectively, was observed in 50 μg/ml CANE-treated cells as compared to control.
Figure 3. CANE induces apoptotic morphology and oxidative stress in A549DR. (A) The apoptotic morphology detected with the help of Hoechst–Propidium iodide staining and quantification done with the help of image J software. Apoptotic cells were calculated as percent apoptotic nuclei compared with a total number of cells. (B) and (C) The oxidative stress induction by CANE in A549DR evaluated with the help of H2DCHFDA (cellular ROS) and Mito-Sox (mitochondrial ROS). Image J software was used for quantification of oxidative stress. Images were taken at 40× magnification [scale bar= 0.1 mm]. Each value in the graph represents as the mean ± SD of three independent experiments. Values with different superscripts differ significantly from each other (p < .05).
![Figure 3. CANE induces apoptotic morphology and oxidative stress in A549DR. (A) The apoptotic morphology detected with the help of Hoechst–Propidium iodide staining and quantification done with the help of image J software. Apoptotic cells were calculated as percent apoptotic nuclei compared with a total number of cells. (B) and (C) The oxidative stress induction by CANE in A549DR evaluated with the help of H2DCHFDA (cellular ROS) and Mito-Sox (mitochondrial ROS). Image J software was used for quantification of oxidative stress. Images were taken at 40× magnification [scale bar= 0.1 mm]. Each value in the graph represents as the mean ± SD of three independent experiments. Values with different superscripts differ significantly from each other (p < .05).](/cms/asset/c8e66f84-9740-4694-b27c-90eb17f6b58c/ianb_a_1434187_f0003_c.jpg)
CANE provokes cell senescence and cell cycle arrest in A549DR cells
Cell senescence potential of CANE was evaluated by the SA-β-gal expression, a hallmark of the cell senescence. Results revealed a dose-dependent increase in SA-β-gal expression in the CANE-treated A549DR cells, suggesting its potential to induce senescence (). A significantly (p < .05) 7.5-fold higher SA-β-gal expression was observed in the cells treated with 50 μg/ml CANE compared to control. Furthermore, the role of CANE in cell cycle arrest was evaluated by examining the expression of cyclin-dependent kinases (CDK2, CDK4 and CDK6), cyclin D1, cyclin E and p21. As depicted in , a significantly (p < .05) dose-dependent reduced expression of CDK2, 4, 6, and a marked increase in p21 expression was noted in the cells treated with CANE. In addition, a dose-dependent reduced expression of cyclin D1 and cyclin E was observed in response to CANE. All the results collectively suggest that CANE induces the cell senescence and cell cycle arrest in A549DR cells.
Figure 4. CANE induces cell senescence and cell cycle arrest. (A) Senescence-associated β-galactosidase (SA-β-Gal) staining of A549DR cells. The percentage of SA-β-Gal-positive cells was calculated from 5 randomly chosen fields. At least 100 cells were analyzed per experiment, each value in the graph represents as the mean ± SD of three independent experiments. Values with different superscripts differ significantly from each other (p < .05). (B) CANE was treated to A549DR cells for 24 h and cell cycle protein levels detected such as CDK2, CDK4, CDK6, Cyclin E, Cyclin D1 and p21. Densitometry analysis of the respective proteins was evaluated by Image J software, and results were normalized with β-actin.
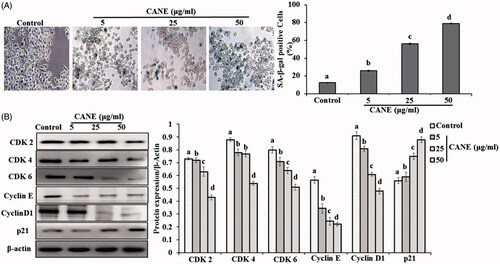
CANE inhibits autophagy in A549DR cells
Several studies have been already investigated regarding the role of autophagy in survival and death of cancer cells. To further, elucidate the role of CANE in autophagy, we examined the expression of important autophagic marker proteins in A549DR cells. Results revealed a dose-dependent decrease of autophagy marker proteins in A549DR cells treated with CANE. A significantly (p < .05) decline in the LC3-I to LC3-II conversion and expression of Beclin-1, ATG 5 and ATG 7 were observed in the A549DR cells treated with the varying concentration of CANE. In contrast to this, a marked dose-dependent enhancement in the expression of p62 and mTOR was observed (). The results clearly exhibited the autophagy inhibition potential of CANE in A549DR cells.
Figure 5. CANE abolishes autophagy in A549DR. (A) CANE was treated to A549DR cells for 24 h and autophagic protein levels detected such as LC-3, p62, Beclin-1, mTOR, ATG5 and ATG7. Densitometry analysis of the respective proteins was evaluated by Image J software, and results were normalized with β-actin with respect to controls. (B) For further confirmation, autophagic vacoule formation detected with the help of fluorogenic dye LysoTracker® Red, DAPI was used as counter stain to stain nucleus. Acridine orange was used to detect the autopaghic vacoule formation. Image J software was used for quantification of oxidative stress. Images were taken at 40× magnification [scale bar= 0.1 mm]. Each value in the graph represents as the mean ± SD of three independent experiments. Values with different superscripts differ significantly from each other (p < .05).
![Figure 5. CANE abolishes autophagy in A549DR. (A) CANE was treated to A549DR cells for 24 h and autophagic protein levels detected such as LC-3, p62, Beclin-1, mTOR, ATG5 and ATG7. Densitometry analysis of the respective proteins was evaluated by Image J software, and results were normalized with β-actin with respect to controls. (B) For further confirmation, autophagic vacoule formation detected with the help of fluorogenic dye LysoTracker® Red, DAPI was used as counter stain to stain nucleus. Acridine orange was used to detect the autopaghic vacoule formation. Image J software was used for quantification of oxidative stress. Images were taken at 40× magnification [scale bar= 0.1 mm]. Each value in the graph represents as the mean ± SD of three independent experiments. Values with different superscripts differ significantly from each other (p < .05).](/cms/asset/22f1bfa7-6683-4f76-ab97-ebbb5b7e15a8/ianb_a_1434187_f0005_c.jpg)
To validate the findings, we used autophagy specific Acridine orange and LysoTracker® Red dye, which helps to detect the progress of autophagy formation by determining the formation of vacuole and lysosome activity, respectively. Autophagic vacuole formation is the vital event during autophagy and we noticed a dose-dependent reduced vacuole formation in A549DR cells treated with CANE. In addition, to this, a dose-dependent inhibition of lysosomal activity was observed in A549DR cells treated with CANE (). These results collectively indicated that CANE inhibits autophagy process in A549DR cell.
CANE induces apoptosis in presence of 3-MA
To confirm the role of autophagy inhibition and induction of apoptosis, we used autophagy inhibitor 3-Methyladenine (3-MA) in presence of CANE and cell viability was evaluated by MTT assay. As depicted in , the presence of 3-MA causes the reduction in cell number, however, the combination of 3-MA and CANE decreases more cell number. The results of MTT assay were further validated with the help of fluorescence staining by LysoTracker® Red and acridine orange which revealed the autophagy inhibition in the presence of 3-MA as well as 3-MA in combination with CANE (). To get the more insight of autophagy and apoptosis crosstalk, we noticed the reduced expression of autophagic protein levels such as LC-3 I to LC-3 II cleavage, Beclin-1, and ATG-5 along with elevated expression of p62. Furthermore, an increased expression of apoptotic protein Bax, cleaved caspase-3, 9 and reduced expression of anti-apoptotic Bcl2 was observed (). Results strongly established that inhibition of autophagy contributes to CANE-induced apoptotic cell death.
Figure 6. CANE inhibits autophagy in A549DR cells. (A) Effects of autophagy inhibition by 3-MA on CANE-induced cell death evaluated by MTT assay. (B) Autophagic vacuole formation is inhibited in presence of 3-MA as well as 3-MA in combination with CANE. Image J software was used for quantification of oxidative stress. Images were taken at 40× magnification [scale bar= 0.1 mm]. (C) Western blotting of autophagy as well as apoptosis markers in presence of 3-MA, β-actin used as loading control. Densitometry analysis was determined by Image J software. Each value in the graph represents as the mean ± SD of three independent experiments. Values with different superscripts differ significantly from each other (p < .05).
![Figure 6. CANE inhibits autophagy in A549DR cells. (A) Effects of autophagy inhibition by 3-MA on CANE-induced cell death evaluated by MTT assay. (B) Autophagic vacuole formation is inhibited in presence of 3-MA as well as 3-MA in combination with CANE. Image J software was used for quantification of oxidative stress. Images were taken at 40× magnification [scale bar= 0.1 mm]. (C) Western blotting of autophagy as well as apoptosis markers in presence of 3-MA, β-actin used as loading control. Densitometry analysis was determined by Image J software. Each value in the graph represents as the mean ± SD of three independent experiments. Values with different superscripts differ significantly from each other (p < .05).](/cms/asset/3eba6c1f-18c5-4753-93fa-7c8ac51e1c2e/ianb_a_1434187_f0006_c.jpg)
CANE induces mitochondrial mediated apoptosis in A549DR cells
To elucidate the role of CANE in induction of apoptosis, the expression pattern of the important apoptotic proteins was determined by Western blotting. Results revealed a dose-dependent elevated expression of apoptotic markers p-JNK, Bax, Cyto C, cleaved caspase-3, 9 and decreased expression of anti-apoptotic Bcl2 in the CANE-treated A549DR cell (). Moreover, an adverse effect of CANE on the mitochondrial membrane potential was noticed by Rhodamine 123 fluorescent staining (). An altered mitochondrial membrane potential facilitates the Cyto C release from mitochondria to cytosol that leads to activation of caspase 9, 3 and eventually starts the apoptosis. Knowing the most vital role of caspase 3 in apoptosis, we confirmed its involvement by immunocytochemistry using a fluorescently tagged antibody against caspase 3 and observed its higher expression as evident by enhanced green fluorescence in the CANE treated cells (). Results signify that CANE-induced cell death via the events leading to mitochondria-mediated intrinsic apoptosis.
Figure 7. CANE induces mitochondrial-mediated apoptosis. CANE (0–50 μg/ml) induces apoptosis in A549DR via mitochondrial-mediated pathway. (A) Apoptotic protein levels (JNK, p-JNK, Bax, Bcl2, Cyto C, Caspase-9 and Caspase-3). Densitometry analysis of the respective proteins was evaluated by Image J software, and results were normalized with β-actin with respect to controls. (B) Fluorescent images of the cells representing mitochondrial membrane potential (Rhodamine 123). Image J software was used to determine the mean fluorescence intensity. Each value in the graphs represents as the mean ± SD of three independent experiments. Values with different superscripts differ significantly from each other (p < .05). (C) Immunocytochemical analysis of activities of caspase-3. Images show double staining using antibody against caspase (C-caspase) and DAPI to view nuclei in the same cells. Cleaved caspase-3 indicating activities of these enzymes, are stained green; DAPI staining of nuclei (blue) was performed to identify dead cells as indicated by condensed nuclei. Images were captured at 40× magnification [scale bar= 0.1 mm].
![Figure 7. CANE induces mitochondrial-mediated apoptosis. CANE (0–50 μg/ml) induces apoptosis in A549DR via mitochondrial-mediated pathway. (A) Apoptotic protein levels (JNK, p-JNK, Bax, Bcl2, Cyto C, Caspase-9 and Caspase-3). Densitometry analysis of the respective proteins was evaluated by Image J software, and results were normalized with β-actin with respect to controls. (B) Fluorescent images of the cells representing mitochondrial membrane potential (Rhodamine 123). Image J software was used to determine the mean fluorescence intensity. Each value in the graphs represents as the mean ± SD of three independent experiments. Values with different superscripts differ significantly from each other (p < .05). (C) Immunocytochemical analysis of activities of caspase-3. Images show double staining using antibody against caspase (C-caspase) and DAPI to view nuclei in the same cells. Cleaved caspase-3 indicating activities of these enzymes, are stained green; DAPI staining of nuclei (blue) was performed to identify dead cells as indicated by condensed nuclei. Images were captured at 40× magnification [scale bar= 0.1 mm].](/cms/asset/eb22bb73-1bab-4654-ab4e-63d8fc3a91c0/ianb_a_1434187_f0007_c.jpg)
NAC mitigates the CANE induced mitochondrial mediated apoptosis in A549DR cells
For a better understanding of the involvement of ROS in CANE-induced apoptosis, we used N-acetyl-l-cysteine (NAC), an effective scavenger of ROS. After co-incubation of NAC (5 mM) with CANE (50 μg/ml), the cell viability was significantly higher as compared to only CANE (50 μg/ml) (). In addition, a visible attenuation of CANE-induced ROS was observed in the NAC and NAC + CANE-treated cells by H2DCFDA fluorescent staining. A significantly (p < .05) 1.6-fold reduced ROS fluorescent intensity was observed in the NAC + CANE-treated cells as compared to only CANE-treated cells (). Moreover, NAC reduces CANE-induced phosphorylation of JNK as well as the expression levels of Bax, Cyto C, cleaved caspase-3 and caspase-9 (). Results strongly support that ROS acts as a crucial mediator of CANE induced mitochondrial-mediated apoptosis.
Figure 8. NAC mitigates the CANE induced mitochondrial-mediated apoptosis in A549DR cells. (A) Effects of ROS scavenger NAC on CANE-induced cell death evaluated by MTT assay. (B) CANE-induced ROS production abolished H2DCFDA fluorescence in presence of NAC. Images were taken at 40× magnification [scale bar= 0.1 mm]. (C) Western blotting of apoptosis pathway markers, β-actin used as loading control. Densitometry analysis was determined by Image J software. Each value in the graph represents as the mean ± SD of three independent experiments. Values with different superscripts differ significantly from each other (p < .05).
![Figure 8. NAC mitigates the CANE induced mitochondrial-mediated apoptosis in A549DR cells. (A) Effects of ROS scavenger NAC on CANE-induced cell death evaluated by MTT assay. (B) CANE-induced ROS production abolished H2DCFDA fluorescence in presence of NAC. Images were taken at 40× magnification [scale bar= 0.1 mm]. (C) Western blotting of apoptosis pathway markers, β-actin used as loading control. Densitometry analysis was determined by Image J software. Each value in the graph represents as the mean ± SD of three independent experiments. Values with different superscripts differ significantly from each other (p < .05).](/cms/asset/b2a10117-252e-45cc-90a6-f7d608ca0551/ianb_a_1434187_f0008_c.jpg)
CANE reduces tumour formation in nude mice
As depicted in , CANE effectively reduces tumour weight and volume in a dose-dependent manner (). Furthermore, Western blotting results suggests inhibition of autophagy and cell cycle arrest in the CANE treated groups compared with control (). An induction of apoptosis was noted in the CANE treated group evident by the upregulation of apoptotic proteins Bax, caspase 9 and 3.
Figure 9. In vivo study. CANE inhibits tumour growth. (A) Tumour images excised from different groups of mice after 4 weeks. (B) Tumour volume with respect to time. (C) Tumour weight after 4 weeks. (D) Body weight with respect to time. (E) Expression of p-JNK, Bax, Bcl2, caspase-9, caspase-3, LC-3, p62, CDK2, CDK4 and β-actin in tumour tissue. Each value in the bar graph represents the mean ± SD of three independent experiments. Values with different superscripts differ significantly from each other (p < .05).
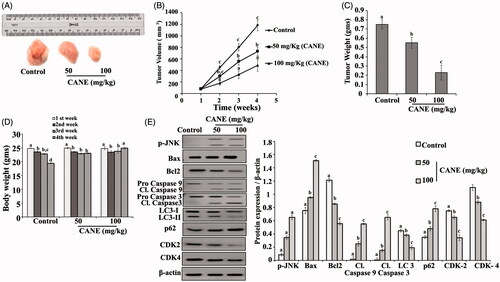
Discussion
Various plants and their components have been utilized worldwide due to their health beneficiary effect. Carvacrol a monoterpenoid is one of the imperative segments of thyme and oregano plants [Citation8–10], has been reported for anti-proliferative property against neuroblastoma, colon and lung carcinoma cell lines [Citation10,Citation22–26]. However, its application often suffers owing to its physicochemical instability [Citation26,Citation27]. Nanoemulsions, prepared by combining oil, water and detergents [Citation28], are an effective way to improve physicochemical instability, drug permeability and bioavailability [Citation29–32]. Nanoemulsion can be made by many ways, however; ultrasonication is an energy intensive, easy and rapid way to synthesize nanoemulsion with greater efficiency in the small level [Citation33,Citation34]. Dynamic light scattering and electron microscopy are the common way to characterized nano-preparation by analyzing size, charge, PDI and morphology features [Citation35,Citation36]. We observed that prepared CANE has nano-sized with sufficient negative surface charge and least PDI, indicating a stable nanoemulsion formation. Results are in accordance with the published reports where electrostatic repulsion occurs due to high negative charge thus stabilized emulsion [Citation37].
Cells can enter into the death phase through different pathways, including apoptosis, autophagy, cell cycle arrest and necrosis [Citation38]. In the quest to evaluate the possible mechanism by which CANE encountered the A549DR cells, we evaluated its cytotoxic properties by MTT, LDH, clonogenicity and soft agar assay. Results showed a potential cytotoxic effect of CANE, which is in accordance with the published reports suggesting the anticancer role of carvacrol [Citation10,Citation24,Citation39]. To the best of our knowledge, the present study is the first report showing the anticancer potential of CANE against drug resistant cancer cells (A549DR).
Cellular aging is a hallmark of cell-senescence which plays a crucial role in tumour suppression and we observed that cells subjected to CANE treatment undergo senescence based on the expression of x-gal, which is a major biomarker of senescence [Citation40]. Progression of the cell cycle occurs in four different phases via activation or inactivation of Cdks, which are serine–threonine kinases that are increased or decreased depending on cellular status and environment. Cyclin D1, CDK4 and CDK6 are required during the initial G1 phase, and the cyclin D-CDK complex is vital for the progression of cell cycle. Once the cell cycle enters the S-phase, the cyclin DCDK complex is not required, and now cyclin E and CDK2 are crucial for the G1 to S phase transition. Finally, the Cdc2-cyclin B complex initiates G2 to M phase transition. The role of various plant terpenes has been reported to target these cyclin-–CDK complexes, which finally hampers the cell cycle. We observed that CANE targets the cell-cycle complex and downregulates the cell cycle biomarkers (CDK2, CDK4, CDK6, Cyclin D1, Cyclin E and p21) which are well known to promote cell growth. The results demonstrated the potential role of CANE in the induction of cell senescence and cell cycle arrest, autophagy inhibition which further induces cell death via the apoptotic pathway [Citation41,Citation42].
Autophagy is a catabolic process activated in response to various external stimuli and plays dual roles such as tumour promotion and tumour suppression. However, it is unclear if autophagy activation leads to cell survival or cell death in response to cancer therapy [Citation43]. Moreover, the role of autophagy depending on cancer treatment remains controversial. A majority of the pertinent research has shown that the autophagic process is a protective mechanism associated with resistance to chemotherapy. Moreover, autophagy plays a protective role in drug resistant cells promoting the survival of tumour cells. Hence, targeted inhibition of autophagy is an effective approach to killing the drug resistant cancer cells. Autophagic cell death is also an alternative mechanism when apoptosis is impaired in cells. Moreover, in drug resistant cells, autophagy plays an important role in cell survival as well as cell resistance against the drug or other metabolic stresses [Citation44,Citation45]. Previous studies have reported that macro-autophagy or autophagy promotes tumour growth by inhibiting apoptosis and promoting autophagy in resistant cancer cells [Citation46]. Thus induction of apoptosis and inhibition of autophagy by any drug will provide an effective way to irradiate such resistant cells. We observed that CANE effectively reduced LC-3 I to II expression and downregulated autophagic markers, which helps to induce apoptosis. Further, data obtained using 3-MA autophagy inhibitor clearly demonstrated that CANE inhibited autophagy that leads to apoptosis in A549DR cells.
Apoptosis is programmed cell death maintained by cells to achieve homeostasis. In the present study, we observed that CANE dose-dependent elevated nuclear fragmentation and chromatin condensation, a hallmark of apoptosis [Citation38]. Moreover, the role of CANE on the induction of ROS has been demonstrated by fluorescent staining. ROS plays a crucial role in inducing apoptosis and is responsible for activation of many apoptotic biomarkers, including JNK. Activated JNK further induces Bax induction, as a result of mitochondrial dysfunction via the inhibition of complex I. The phosphorylated form of JNK (p-JNK) activates Bax, which plays an important role in apoptosis by altering mitochondrial membrane permeability, facilitating the release of Cyto C. A549DR cells treated with CANE induced ROS and upregulate Bax leads to dissipation of mitochondrial membrane potential (Δψm) and membrane weakening. Once the mitochondrial membrane potential (Δψm) changes, cytochrome C is released from it to the cytosol, which further activates proteases such as caspase 9, caspase-3 commencing to apoptotic cell death. To validate the role of ROS in CANE induced mitochondrial-mediated apoptosis, we used NAC as ROS scavenger which indicates ROS inhibition diminishes the CANE-induced apoptotic cell death. Results are consistent with the published reports suggesting a mitochondrial dependent apoptotic potential of many natural compounds [Citation37,Citation39].
The in vitro results suggest a strong cytotoxic potential of CANE, hence, we moved further and examined its in vivo efficacy by xenografting, A549DR cells in nude mice. The outcome of the study revealed that CANE effectively suppresses tumour growth by the inhibition of autophagy, cell cycle and induction of apoptosis. In vivo results are consistent with our in vitro findings suggesting a great efficiency of CANE to deal with A549DR cells.
In conclusion, results revealed that CANE induces cell cycle arrest and cell senescence. In addition, a potential role of CANE in the inhibition of autophagy and induction of apoptosis was noted. To the best of our knowledge, this is the first report representing the anticancer potential role of CNAE (in vitro and in vivo) against drug resistant cells by the inhibition of autophagy mediated apoptosis. We conclude that CANE can be useful for cancer therapy after further clinical study.
Disclosure statement
No potential conflict of interest was reported by the authors.
Additional information
Funding
References:
- Dela Cruz CS, Tanoue LT, Matthay RA. Lung cancer: epidemiology, etiology, and prevention. Clin Chest Med. 2011;32:605–644.
- Alfarouk KO, Stock CM, Taylor S, et al. Resistance to cancer chemotherapy: failure in drug response from ADME to P-gp. Cancer Cell Int. 2015;15:71.
- Juliano RL, Ling V. A surface glycoprotein modulating drug permeability in Chinese hamster ovary cell mutants. Biochim Biophys Acta. 1976;455:152–162.
- Mondal S, Bandyopadhyay S, Ghosh MK, et al. Natural products: promising resources for cancer drug discovery. Anticancer Agents Med Chem. 2012;1:49–75.
- Safarzadeh E, Sandoghchian Shotorbani S, Baradaran B. Herbal medicine as inducers of apoptosis in cancer treatment. Adv Pharm Bull. 2014;4:421–427.
- Rein MJ, Renouf M, Cruz-Hernandez C, et al. Bioavailability of bioactive food compounds: a challenging journey to bioefficacy. Br J Clin Pharmacol. 2013;3:588–602.
- Renukuntla J, Vadlapudi AD, Patel A, et al. Approaches for enhancing oral bioavailability of peptides and proteins. Int J Pharm. 2013;447:75–93.
- Baser KH. Biological and pharmacological activities of carvacrol and carvacrol bearing essential oils. Curr Pharm Des. 2008;29:3106–3119.
- Khan I, Bahuguna A, Kumar P, et al. Antimicrobial potential of carvacrol against uropathogenic Escherichia coli via membrane disruption, depolarization, and reactive oxygen species generation. Front Microbiol. 2017;8:2421.
- Khan I, Bahuguna A, Kumar P, et al. In vitro and in vivo antitumor potential of carvacrol nanoemulsion against human lung adenocarcinoma A549 cells via mitochondrial mediated apoptosis. Sci Rep. 2018;8:144.
- Hassan M, Watari H, AbuAlmaaty A, et al. Apoptosis and molecular targeting therapy in cancer. Biomed Res Int. 2014:2014:150845.
- Abdolahpour S, Toliyat T, Omidfar K, et al. Targeted delivery of doxorubicin into tumor cells by nanostructured lipid carriers conjugated to anti-EGFRvIII monoclonal antibody. Artif Cells Nanomed Biotechnol. 2018;1:89–94.
- Mi J, Zhang X, Rabbani ZN, et al. RNA aptamer-targeted inhibition of NF-kappa B suppresses non-small cell lung cancer resistance to doxorubicin. Mol Ther. 2008;16:66–73.
- Khan I, Paul S, Jakhar R, et al. Novel quercetin derivative TEF induces ER stress and mitochondria-mediated apoptosis in human colon cancer HCT-116 cells. Biomed Pharmacother. 2016;84:789–799.
- Borowicz S, Van Scoyk M, Avasarala S, et al. The soft agar colony formation assay. J Vis Exp. 2014;92:51998.
- Franken NA, Rodermond HM, Stap J, et al. Clonogenic assay of cells in vitro. Nat Protoc. 2006;1:2315–2319.
- Li Z, Jiang K, Zhu X, et al. Encorafenib (LGX818), a potent BRAF inhibitor, induces senescence accompanied by autophagy in BRAFV600E melanoma cells. Cancer Lett. 2016;370:332–344.
- Ahmad U, Akhtar J, Singh SP, et al. Silymarin nanoemulsion against human hepatocellular carcinoma: development and optimization. Artif Cells Nanomed Biotechnol. 2017;1–11. doi: https://doi.org/10.1080/21691401.2017.1324465
- Khan I, Kang SC. Apoptotic activity of Lactobacillus plantarum DGK-17-fermented soybean seed extract in human colon cancer cells via ROS-JNK signaling pathway. J Food Sci. 2017;82:1475–1483.
- Bhardwaj M, Paul S, Jakhar R, et al. Vitexin confers HSF-1 mediated autophagic cell death by activating JNK and ApoL1 in colorectal carcinoma cells. Oncotarget. 2017;8:112426–112441.
- Rong L, Zhou S, Liu X, et al. Trastuzumab-modified DM1-loaded nanoparticles for HER2+ breast cancer treatment: an in vitro and in vivo study. Artif Cells Nanomed Biotechnol. 2017;1–11. doi: https://doi.org/10.1080/21691401.2017.1391821
- Xu H, Delling M, Jun JC, et al. Oregano, thyme and clove-derived flavors and skin sensitizers activate specific TRP channels. Nat Neurosci. 2006;5:628–635.
- Bukovská A, Cikos S, Juhás S, et al. Effects of a combination of thyme and oregano essential oils on TNBS-induced colitis in mice. Mediators Inflamm. 2007;2007:23296.
- Hotta M, Nakata R, Katsukawa M, et al. Carvacrol, a component of thyme oil, activates PPAR alpha and gamma and suppresses COX-2 expression. J Lipid Res. 2010;51:132–139.
- Sobral MV, Xavier AL, Lima TC, et al. Antitumor activity of monoterpenes found in essential oils. Sci World J. 2014;2014:953451.
- Bayala B, Bassole IH, Scifo R, et al. Anticancer activity of essential oils and their chemical components – a review. Am J Cancer Res. 2014;4:591–607.
- Bilia AR, Guccione C, Isacchi B, et al. Essential oils loaded in nanosystems: a developing strategy for a successful therapeutic approach. Evid Based Complement Alternat Med. 2014;2014:651593.
- Gupta S, Kesarla R, Omri A. Formulation strategies to improve the bioavailability of poorly absorbed drugs with special emphasis on self-emulsifying systems. ISRN Pharm. 2013;2013:848043.
- Turek C, Stintzing FC. Stability of essential oils: a review. Compr Rev Food Sci Food Saf. 2013;12:40–53.
- Kesarwani K, Gupta R. Bioavailability enhancers of herbal origin: an overview. Asian Pac J Trop Biomed. 2013;3:253–266.
- Grapentin C, Barnert S, Schubert R. Monitoring the stability of perfluorocarbon nanoemulsions by cryo-TEM image analysis and dynamic light scattering. PLoS One. 2015;10:e0130674.
- Abbasi S, Radi M. Food grade microemulsion systems: canola oil/lecithin: n-propanol/water. Food Chem. 2016;194:972–979.
- Hashtjin AM, Abbasi S. Optimization of ultrasonic emulsification conditions for the production of orange peel essential oil nanoemulsions. J Food Sci Technol. 2015;52:2679–2689.
- Miastkowska MA, Banach M, Pulit-Prociak J, et al. Statistical analysis of optimal ultrasound emulsification parameters in thistle-oil nanoemulsions. J Surfact Deterg. 2017;20:233–246.
- Ahmad N, Ahmad R, Naqvi AA, et al. The effect of safranal loaded mucoadhesive nanoemulsion on oxidative stress markers in cerebral ischemia. Artif Cells Nanomed Biotechnol. 2017;45:775–787.
- Ma J, Zhuang H, Zhuang Z, et al. Development of docetaxel liposome surface modified with CD133 aptamers for lung cancer targeting. Artif Cells Nanomed Biotechnol. 2017;30:1–8.
- Harnsilawat T, Pongsawatmanit R, McClements DJ. Influence of pH and ionic strength on formation and stability of emulsions containing oil droplets coated by beta-lactoglobulin-alginate interfaces. Biomacromolecules. 2006;7:2052–2058.
- Elmore S. Apoptosis: a review of programmed cell death. Toxicol Pathol. 2007;35:495–516.
- Bhakkiyalakshmi E, Suganya N, Sireesh D, et al. Carvacrol induces mitochondria-mediated apoptosis in HL-60 promyelocytic and Jurkat T lymphoma cells. Eur J Pharmacol. 2016;772:92–98.
- Lee BY, Han JA, Im JS, et al. Senescence-associated beta-galactosidase is lysosomal beta-galactosidase. Aging Cell. 2006;5:187–195.
- Zhu J, Zhou Y, Wang GN, et al. Cell cycle arrest, apoptosis and autophagy induced by iminosugars on K562 cells. Eur J Pharmacol. 2014;15:65–72.
- Ren J, Xu Y, Huang Q, et al. Chabamide induces cell cycle arrest and apoptosis by the Akt/MAPK pathway and inhibition of P-glycoprotein in K562/ADR cells. Anticancer Drugs. 2015;26:498–507.
- Maycotte P, Thorburn A. Autophagy and cancer therapy. Cancer Biol Ther. 2011;11:127–137.
- Sui X, Chen R, Wang Z, et al. Autophagy and chemotherapy resistance: a promising therapeutic target for cancer treatment. Cell Death Dis. 2013;4:e838.
- Towers CG, Thorburn A. Therapeutic targeting of autophagy. EBioMedicine 2016;14:15–23.
- Glick D, Barth S, Macleod KF. Autophagy: cellular and molecular mechanisms. J Pathol. 2010; 221:3–12.