Abstract
Current treatments of oesophageal diseases, such as carcinoma, congenital abnormality or trauma, require surgical intervention and oesophageal reconstruction with the stomach, jejunum or colon. However, serious side effects are possible with each treatment option. Despite tissue engineering promising to be an effective regenerative strategy, no functional solution currently exists for oesophageal reconstruction. Here, we developed an omentum-cultured oesophageal scaffold reinforced by a 3D-printed ring. The nano-structured scaffolds were wrapped into the omentum of rats and orthotopically transplanted for the repair of circumferential oesophageal defects two weeks later. The artificial oesophagus exhibited complete healing of the surgically created circumferential defects by the second week. The integration of the omentum-cultured oesophageal scaffold and the regenerative tissue remained intact. Macroscopically, there was no evidence of a fistula, perforation, abscess formation or surrounding soft-tissue necrosis. The omentum-cultured nano-structure scaffold reinforced by a 3D-printed ring is a more practical model with better vascularization for artificial neo-oesophagus reconstruction in a rat model.
Background
Current treatments of oesophageal diseases, such as carcinoma, congenital abnormality or trauma, require surgical intervention and oesophageal reconstruction with the stomach, jejunum or colon. However, the use of these oesophageal substitutes can cause various surgical morbidities and mortality. Anastomosis site leakage and necrosis of the implanted substitutes inevitably cause extremely high mortality because the mediastinum and neck compartments become contaminated [Citation1].
Several types of auto or alloplastic circumferential oesophageal substitutes using natural and synthetic materials have been applied for oesophageal replacement [Citation2–4]. Natural biomaterials, including decellularized extracellular matrix (ECM), collagen, chitosan and gelatine derived from animal sources, have been studied due to their excellent biocompatibility and biospecific signals cued from the ECM. Despite promising results in some in vitro or in vivo experiments, problems such as source shortage, low mechanical strength and fast degradation are limitations of these materials. This is where synthetic polymers, such as poly(caprolactone) (PCL), poly(L-lactic acid) (PLLA), poly(lactide-coglycolide), PCL/PLLA, poly(glycolic acid) and poly(L-lactide-co-caprolactone), become attractive materials. In addition to high availability, low cost and ease of design and production, a key advantage of synthetic polymers is that they can be optimized for their specific mechanical properties and applications. However, many synthetic materials have limitations to their hydrophobic and biologically inert surface [Citation2–4].
The oesophageal scaffold must provide adequate mechanical properties, which are vital for allowing natural food passage with varying stresses and strains [Citation4]. Despite tissue engineering promising to be an effective strategy for oesophageal regeneration, the development of optimal biomaterials that mimic the unique intrinsic nature of native oesophagus remains challenging. Post-transplantation leakage, strictures, infections, inflammations, immune rejection, poor re-epithelization and poor muscle regeneration in the grafts are still the main problems to solve to date. For the best surgical outcome, the mechanical strength and compliance of the scaffold should be similar to the value of normal oesophagus, and it should be possible to optimally align the wound edges during anastomosis [Citation2].
To overcome these problems, we propose a hybrid technology combining a 3D-printed reinforcement ring for mechanical strength, especially at the anastomosis site, and evaluated merits of using omentum for the growth and vascularization of tissues prior to implantation.
Methods
Fabrication of hybrid scaffolds reinforced by a3D-printed ring
The scaffold blended with a reinforcement ring was fabricated by combining 3D printing and electrospinning methods. The first step was to fabricate poly(ε-caprolactone) (PCL) rings on a stainless rod. The 3D-plotted scaffolds were prepared using BT-3000 (NBR-Tech Co., Ltd., Chuncheon-si, Gangwon-do, Korea). Biodegradable PCL (average molecular weight of 45,000 Da, Sigma-Aldrich, St. Louis, MO) was transferred into the melting cartilage, which is similar to a single air dispensing system. The melted polymer was plotted with a 300 μm dispensing stainless steel needle. The serial PCL rings were plotted over a 200 μm distance. The geometric size of the fabricated PCL rings was 2.5 × 2.5 × 1 mm [Citation3]. After creating the serial PCL rings, tubular PCL was fabricated by electrospinning a 10% (w/v) PCL (MW 80,000) solution. The electrospun solution was prepared by dissolving polymer pellets in a solvent mixture of DMF and THF (Sigma-Aldrich, 50:50 (v/v)), which stood overnight with continuous stirring (60 °C). For the electrospinning process, the PCL solution was put in two bottles of 10-ml plastic syringes with a 22-needle gauge (0.4 mm ID ×0.7 mm OD) at a constant flow of 0.4 ml/h for 2 h using a syringe pump to maintain the solution drop at the tip of the needle without dripping. The needle was connected to a high-voltage power source, and a continuous supply of solution could be maintained at a rate of 0.4 ml/h using a syringe pump. The voltages applied for electrospinning were +30 kV and −30 kV, and the collecting distance between the needle tip and the collector was maintained at 15 cm ().
Figure 1. Fabrication of the oesophageal scaffold reinforced by 3D-printed rings. The scaffold blended with reinforcement rings was fabricated by combining 3D printing and electrospinning methods. (A) The first step involved the fabrication of PCL rings on a stainless rod. (B and C) After creating the serial PCL rings, tubular PCL was collected from electrospinning. (D) The final oesophageal scaffold with reinforced rings. Abbreviations: PCL: Poly ε-caprolactone.
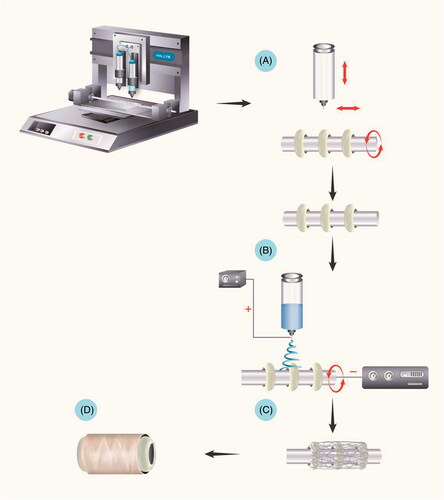
Characterization
The cross-sectional morphology of the PCL scaffold was investigated using an environmental scanning electron microscope (E-SEM, S-3500 N, Hitachi, Tokyo, Japan) in low vacuum mode at the Korean Basic Science Institute, Chuncheon. The process prior to viewing included PCL scaffold paste preparation on a carbon tape and sputter-coating with a thin layer of silver/palladium (10 nm) for two consecutive cycles of 30 s at 15 mA with anion sputter (Hitachi, Tokyo, Japan). After sample coating, the micrographs from each sample were taken at an accelerating voltage of 10 kV and at a magnification of 1 K.
Mechanical properties of the scaffolds
Mechanical properties were measured using a Universal Testing Machine (QM100S, Qmesys, Korea) with a 10 kgf maximum load cell under a crosshead speed of 5 mm/min. A tensile strength test was carried out with cylindrically shaped scaffolds measuring 2.61 mm in outer diameter and 5 mm in thickness. The tensile strength of each scaffold sample (three dry specimens and three wet specimens) was measured by computing the maximum stress. The samples were conditioned in constant temperature and constant humidity (25 °C, 65% RH) prior to measurement.
Cytotoxicity assay
NIH 3T3 cells were cultured onto the scaffolds, and the CCK-8 (Enzo Life Sciences, Farmingdale, NY) reduction assay was used for the assessment of enzyme activity in cells. Samples were uniformly cut into round-shaped discs with 2.61 mm outer diameters with the help of biopsy punchers (Kasco, Keys Cutaneous Punch, Sialkot, Pakistan), which gave them a suitable size to attach to the bottom of a 96-well plate. The samples were sterilized for 30 min in 70% ethanol, washed twice in phosphate-buffered solution and dipped in excess Dulbecco’s Modified Eagle Medium (DMEM) supplemented with 10% foetal bovine serum (Welgene Fresh mediaTM) and 1% penicillin-streptomycin. Finally, DMEM was forced to penetrate deep inside the pores of the scaffolds by vacuum mixing before cell seeding. Cell seeding was carefully performed by pouring 100 μl of DMEM containing (20,000 cells/well) on top of scaffolds.
The cells were cultured for 1, 7 and 14 days, and the culture media was replaced every 48 h. After the required incubation time, the medium in the 96-well plate was suctioned out. The medium was pipetted out, and the cell-bound scaffolds were carefully transferred into micro-centrifuge tubes, followed by treatment with 200 μl of a CCK-8 solution (original CCK-8 solution mixed with DMEM in a 1:10 ratio). These plates were incubated for 2 h at 37 °C to enable the reaction with live cells. Only the cells associated with the scaffolds were evaluated using the CCK-8 assay because of cell escape from the scaffolds and growth at the bottom of the plates after cell seeding. The tubes containing the scaffolds with attached cells were sonicated using a bath sonicator (60 kHz, Shenzhen Codyson Electrical Co., Ltd., China) for 3 min. After sample sonication, the red-coloured CCK-8 solution that contained solubilized formazan was obtained from each sample and added to a 96-well plate for spectrophotometric analysis measured by an ELISA microplate reader (Molecular Devices, SpectraMax® Plus 384, San Jose, CA) capable of reading at 450 nm. To determine the cell viability, the absorbance of the cells cultured on the scaffolds was compared to that of the control well containing a CCK-8 solution, which was mixed with DMEM at a ratio of 1:10.
Animal study
The in vivo studies were conducted in accordance with the guidelines and approval of the Institutional Animal Care and Use Committees (IACUC) of Hallym University (Hallym-2013–20). The serial process of scaffold culture and implantation is shown in . Ten adult Sprague-Dawley rats that weighed 250–270 g each were used in the study. Animals were anaesthetized with inhalation anaesthetics. Induction of the inhalational anaesthesia was achieved by means of an induction chamber filled with 3% isoflurane in a nitrous oxide and oxygen mixture (75:25) (Air Liquid America, Houston, TX). After anaesthesia, the rats were mechanically ventilated with a rodent ventilator (Harvard Apparatus, South Natick, MA). Animals were placed on a heating blanket to maintain their core temperature at 36.5–37.5 °C. The animals were prepared for surgery by removing hair from the surgical site, followed by disinfection; then, the skin incisions were made.
Stage I: Oesophageal scaffold implantation into the greater omentum
The peritoneal cavity was entered through a small midline incision, and the greater omentum was brought out from the peritoneal cavity. The oesophageal scaffold was embedded within the lower part of the greater omentum and wrapped using 4–0 Vicryl sutures (Ethicon, Inc., Somerville, NJ). All incisions were closed in layers (). After two weeks of greater omentum culture, the abdomen was reopened through the previous incision. The grafts were found, and the greater omentum with the graft was dissected (). The grafts and cultured tissue around the scaffold were excised and flushed with physiologic saline solution containing penicillin G (200 units per mL).
Stage II: Orthotopic oesophageal scaffold implantation into the cervical oesophagus
After two weeks of greater omentum culture, the harvested oesophageal scaffold implantation was performed. The lumen of the oesophagus is originally very small (approximately 3 mm in diameter); therefore, a major part of the lumen seemed to be closed after omentum culture because of tissue growth inside the scaffold. However, most this obstruction is membranous. We implanted this scaffold after bougination with a small needle (1 cc syringe), which made the lumen patent. This procedure did not have any clinical problems when we implanted this scaffold in a rat model. After exposure of the tracheoesophageal column, a circumferential defect including all layers of the cervical oesophagus was produced. The circumferential replacement of the oesophageal defect by omentum-cultured scaffold was conducted with the proximal and distal anastomoses under a surgical microscope (). One side of strap muscle was laid over the implanted scaffold for reinforcement.
Figure 3. Gross morphology of oesophageal implantation into the greater omentum. (A) The greater omentum was brought out of the peritoneal cavity. (B) The oesophageal scaffold was embedded into the lower portion of the greater omentum and wrapped using 4–0 Vicryl sutures. (C) After two weeks of implantation, the cultured scaffold was harvested. (D) Orthotopic-cultured oesophageal scaffold implantation into the cervical oesophagus.
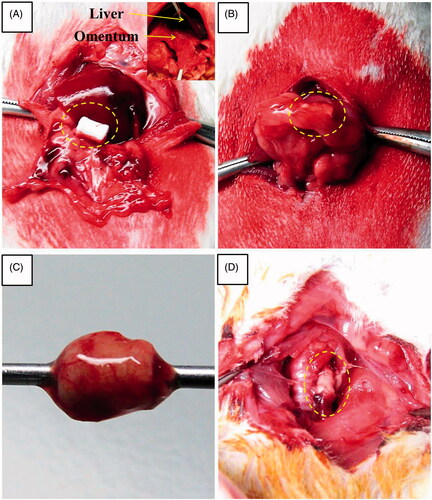
Postoperative procedure
The animals were given liquid food starting on the third day after surgery until the study end point. The rats were sacrificed after one and two weeks of scaffold implantation. The inside and outside of the grafts were evaluated macroscopically, and the scaffold with adjacent oesophageal tissue was fixed in 4% paraformaldehyde and embedded in a paraffin block for histological analysis.
Histological examination
The collected tissue specimens were fixed in 4% buffered paraformaldehyde for at least 24 h, progressively dehydrated in solutions containing an increasing percentage of ethanol (70%, 80%, 90% and 100% (v/v)), cleared in xylene, embedded in paraffin, sectioned at 7-μm thickness, de-paraffinized, and stained with haematoxylin and eosin (H&E) and Verhoeff's van Gieson Stain and Masson’s trichrome (MT). After embedding the tissue in paraffin, tissue slices were de-paraffinized for the expression of platelet endothelial cell adhesion molecule (CD31/PECAM-1) and vascular endothelial growth factor (VEGF). The primary Abs used was CD31/PECAM-1 (Novus) at a dilution of 1:100 and VEGF (Santa Cruz) at a dilution of 1:100. IgG-Alexa Fluor 488 (dilution 1:200, Invitrogen) was used as the second Abs, and DAPI was used for counterstaining. Histological observation was performed under a light microscope (Eclipse 80i, Nikon, Tokyo, Japan).
Results
Characterization
The average outer and inner diameters of the printed PCL ring were 2119 ± 26 µm and 1577 ± 11 µm, respectively, and the average thickness of the printed PCL ring was 261 ± 59 µm. The nano-fibres prepared by the electrospun technique had an average fibre diameter of 2.69 ± 2.41 µm and an average pore size of 5.13 ± 3.31 µm. The mean electrospun thickness was 281 ± 98 µm. The cross-sectional and surface morphology of the PCL scaffolds were investigated with scanning electron microscopy (SEM). shows the surface morphology and cross-sectional images of the nano-fibres with the diameter-distribution histogram. The oesophageal scaffold was composed of a PCL scaffold with a reinforced ring. We could clearly observe the nano-fibre structure and the porous structure from the cross-sectional image of the scaffold (). This high porosity might play an important role when cells migrate on the scaffold.
Figure 4. Scanning electron microscopy images of the PCL scaffold with reinforcement ring. (A and B) The scaffold was composed of a PCL with reinforcement rings. (C) The nano-fibre-based structure of the scaffold. (D) The highly porous structure of the scaffold. (E and F) Gross appearance of hybrid scaffolds reinforced by a 3D-printed ring before implantation. (G and H) The diameter-distribution histogram. PCL: Poly ε-caprolactone.
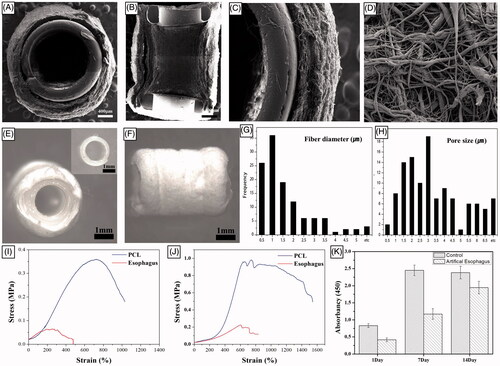
Mechanical properties
The maximal horizontal elongation percentage of PCL scaffolds was 1543 ± 94.2 at a horizontal ultimate tensile strength of 982 ± 35.63 gf and vertical elongation percentage of PCL scaffold was 1032 ± 60.7 at a vertical ultimate tensile strength of 359 ± 14.12 gf. In addition, the maximal horizontal elongation percentage of a normal rat oesophagus was 229.5 ± 6.36 at a horizontal ultimate tensile strength of 229.5 ± 6.36 gf and vertical elongation percentage of a normal rat oesophagus was 764 ± 41.01 at a vertical ultimate tensile strength of 67 ± 2.82 gf (). Based on these values, the scaffold exhibited high ultimate elongation, which suggested that the scaffold can mimic native oesophageal characteristics. Although the tensile test was not conducted in a cyclic manner, these results demonstrate that the developed method may be useful for producing a strong artificial oesophagus with optimal scaffold suturability.
Cytotoxicity assay
shows the data from the CCK-8 assay after culture for 1, 7 and 14 days of incubation. The NIH 3T3 fibroblasts gradually increased their cell proliferation, indicating the favourable environment provided by the high porosity scaffold. As shown in , we found that the growth of the NIH 3T3 cells increased from day 1 to 14 in an exponential manner. These results suggest that no cytotoxicity was observed in the prepared scaffolds.
Gross inspection
Cultured oesophageal scaffold in the greater omentum
After two weeks of greater omentum culture, the embedded implant was retrieved from the omentum. The graft maintained a tubular structure without any infection or adhesion. In addition, the scaffold was easily removed. The cultured oesophageal graft was intact, and tissue regeneration from the greater omentum inside and outside of the scaffold was observed. The regenerated scaffold surface alignment was symmetric and optimal for surgical anastomosis ().
Figure 5. Macroscopic appearance of reconstructed artificial neo-oesophagus constructs (A and B). After two weeks of greater omentum culture, the embedded implant was retrieved from the omentum. The cultured oesophageal graft was intact, and tissue regeneration from the greater omentum inside and outside of the scaffold was observed. The regenerated scaffold surface alignment became symmetric. Gross morphology after the implantation. (C) Orthotopic-cultured oesophageal scaffold implantation into the cervical oesophagus. (D) Gross morphology of reconstructed artificial oesophagus constructs after the second week from the implant. There was no evidence of a fistula, perforation, abscess formation or surrounding soft-tissue necrosis. The dotted line shows the continuity of the artificial oesophagus and the native oesophagus.
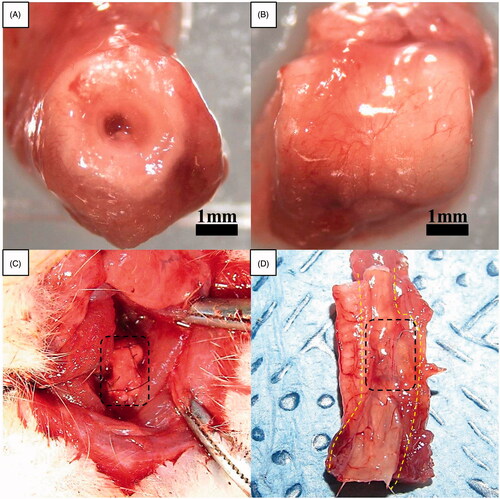
Orthotopic oesophageal scaffold implantation into the cervical oesophagus
The groups of rats were sacrificed after the first and second weeks. All rats were sacrificed or died prior to the 15th postoperative day in our study due to a nutritional problem caused by graft obstruction with coprophagy or hair, originating from the animals’ grooming. However, there was no gross evidence of perforation, fistula, seroma accumulation, abscess formation or surrounding soft-tissue necrosis in most of the rats sacrificed after the first and second weeks ().
Histology
Cultured oesophageal scaffold in the greater omentum
Histological results for the haematoxylin-eosin–stained axial and longitudinal section of the omentum-cultured scaffold were obtained. The scaffold material was integrated into the omentum, and a mixture of inflammatory cells and fibroblasts infiltrated inside and outside of the scaffold. The host cells were aligned with the cylindrical direction inside and outside of the scaffold. Several newly developed blood vessels on the outer side of the scaffold indicated a tissue regeneration process (). The merged file of DAPI (4,6-diamidino-2-phenylindole)/F-Actin indicated that the host cells infiltrated into the scaffold and along the inner/outer wall of the scaffold ().
Figure 6. Histological results for the haematoxylin-eosin–stained axial (A) and longitudinal (B) section of omentum-cultured scaffold. The scaffold material was integrated into the omentum, and a mixture of inflammatory cells and fibroblasts infiltrated inside and outside of the scaffold. The host cells were aligned with the cylindrical direction inside (C) and outside (D) of the scaffold. (E) Several newly developed blood vessels in the outer side of the scaffold revealed the tissue regeneration process. Immunohistochemistry of omentum-cultured scaffold. DAPI (4,6-diamidino-2-phenylindole) staining (F). Rhodamine phalloidin staining for F-actin (G). The merged file of DAPI (4,6-diamidino-2-phenylindole)/F-Actin (H) indicated that the host cells infiltrated into the scaffold and along the inner/outer wall of the scaffold.
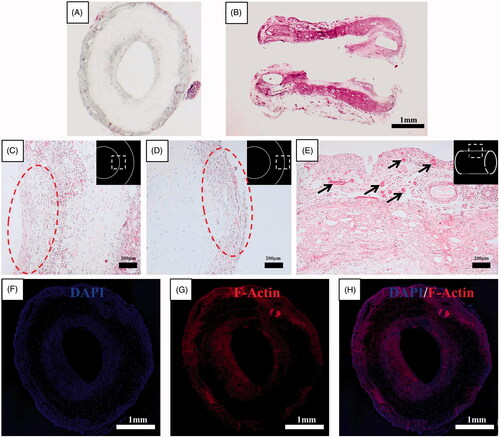
Orthotopic oesophageal scaffold implantation into the cervical oesophagus
The histological results in the haematoxylin-eosin–stained sections two weeks after orthotopic scaffold implantation revealed that the integration of the omentum-cultured oesophageal scaffold and the regenerative tissue remained intact. The artificial oesophagus exhibited complete healing of surgically created circumferential defects by the second week. The structural integrity was maintained with the alignment of the infiltrated host cells into the scaffold, and there were only mild inflammatory reactions with minimal granulation tissue. Squamous epithelium and soft-tissue regeneration developed from the periphery of the oesophageal defect. This epithelial regeneration started from both oesophageal stumps along the PCL ring, but the centres of the regenerated oesophagi had not become re-epithelialized. Elastin and collagen fibre deposition were observed in all parts of the artificial oesophagus with layered structures. Tissue regeneration originated from the natural oesophagus and connected to either end of the artificial oesophagus (). Collagen and elastin are two important structural proteins of the ECM that contribute to optimal mechanical properties [Citation4]. The merged file of DAPI/CD31 and DAPI/VEGF indicated that neovascularization developed in the implanted scaffold ().
Figure 7. Histological results for the haematoxylin-eosin–stained sections two weeks after orthotopic scaffold implantation. (A) At the end of the second week, a PCL with reinforcement rings could be identified. The artificial oesophagus constructs showed healing of the circumferential defects by the second week. The fusion of the PCL scaffold and regenerative tissue was intact. Mild inflammatory reactions and minimal granulation tissue formation were observed. (B) Several newly developed blood vessels in the outer side of the scaffold indicated tissue regeneration. The structural integrity was maintained by the alignment of infiltrated host cells into the scaffold. The dotted line represents the boundary between the omentum-cultured scaffold and the regenerated tissue covering the scaffold. (C) Squamous epithelium and soft-tissue regeneration occurred at the periphery of the oesophageal defect. Histological analysis for the Verhoeff's van Gieson Stain (D, E, F, G) and Masson's trichrome (H, I, J, K) sections two weeks after implantation. Elastin (arrows in E and F) and collagen (arrows in H, I, and J) fibre deposition were observed in all parts of the artificial oesophagus with layered structure. The tissue regeneration originated from the natural oesophagus and connected to either end of the artificial oesophagus. The dotted line represents the PCL ring. Immunohistochemistry of the implanted scaffold. DAPI (4,6-diamidino-2-phenylindole) staining (L and O). Platelet endothelial cell adhesion molecule (CD31/PECAM-1) (M) and VEGF staining (P). The merged file of DAPI/CD31 (N) and DAPI/VEGF (Q) indicated that neovascularization developed in the implanted scaffold (white arrows). PCL: Poly ε-caprolactone.
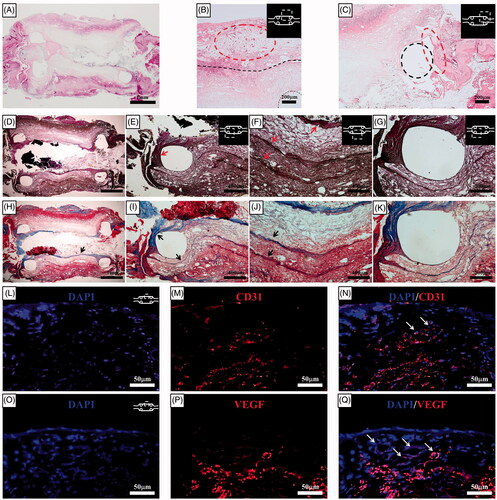
Discussion
The treatment of oesophageal disorders, such as congenital anomalies and oesophageal cancer, requires the removal of a significant portion of the affected area, but the oesophagus is a complex organ comprising non-redundant tissue that does not have the ability to regenerate. Hence, surgical repair and/or replacement of the oesophagus are the only feasible treatment options upon extensive structural damage. Several techniques have been described to reconstruct digestive continuity in patients with an oesophageal defect. Gastric pull-up is the most frequent reconstruction, and colon or jejunum conduits are also used [Citation5–7]. However, the use of these oesophageal substitutes can cause various surgical morbidities and mortality that lead to failure due to surgical constraints [Citation1]. Hence, tissue engineering and regenerative medicine are upcoming alternatives to develop a mimic of the native oesophagus that could be used as artificial graft.
The ideal oesophageal substitute should have adequate biocompatibility and resistance to gastric acid. In addition, the substitute should attract cells (i.e. epithelial cells, smooth muscle cells and fibroblasts) to infiltrate inside the scaffold, stimulate proliferation and not cause infection or inflammation. The substitute should be mechanically stable with optimal mechanical and suture retention strengths to withstand the pressure from a food bolus. The viscoelasticity of the scaffold is a very important factor for adequate peristaltic movement of food through the oesophagus. Most of the all, the dilatation and bursting strength of the scaffold should match the native oesophagus because it should neither leak nor shrink [Citation8].
However, conventional animal research on an artificial oesophagus is challenging due to several critical factors. (1) Circumferential reconstruction of the oesophagus by an all acellular matrix that is used alone without cell seeding invariably leads to the absence of tissue remodelling and stricture formation [Citation8]. Anastomosis site leakage and necrosis of the implanted scaffold in a circumferential oesophageal defect inevitably causes extremely high mortality because the mediastinum and neck compartments become contaminated. (2) Any type of scaffold manufactured from electrospun nano-fibres that are composed of biocompatible materials suffer from poor mechanical properties. For the best surgical outcome to minimize anastomosis site leakage or stenosis, the mechanical properties, including compliance, should be comparable to physiological values, and an optimal alignment of the wound edges during anastomosis should be possible. Therefore, the development of superior biomaterials that mimic the native extracellular milieu remains challenging [Citation2].
In our study, we attempted 3D printing of the reinforcement ring for mechanical strength and temporary implantation of the scaffold in a natural bioreactor, such as the greater omentum, prior to implantation. This is the first study to assess the use of an artificial oesophagus with a PCL reinforcement ring scaffold cultured in the greater omentum in a rat model.
The mechanical strength of the oesophagus is extremely unique and important. The oesophageal scaffold must be distensible, but not flaccid, because the native oesophagus is constantly exposed to cyclic stretching by food boluses. Hence, the engineered oesophagus should have similar characteristics to the native one. To transport food boluses from the mouth to the stomach properly, the compliance of the tissue-engineered oesophagus should withstand the repeated cycles of applied stress associated with swallowing [Citation5]. If the scaffolds are too weak, it may rupture or leak and cause serious complications, such as mediastinitis. In contrast, a scaffold that is too stiff could also block the oesophageal lumen and prevent the passage of food. Tan et al. [Citation4] reported that the elastin and collagen fibres in the submucosal layer of the oesophagus can contribute towards extraordinary synergy in its mechanical properties.
In addition, the neo-oesophageal scaffold should be implanted and connected between the upper and lower oesophagus. Thus, it is also important that the ideal oesophageal scaffold must possess optimal suturability from a surgical perspective. This suturability allows suture materials and needles to easily pass through, and the native tissue should resist tearing while being sutured. In this aspect, the scaffold must possess enough suture retention strength to hold under longitudinal and radial stresses, which have great importance in animal studies in which excessive movements from the animal may lead to scaffold rupture and mortality.
Electrospinning has been introduced as a good method for fabricating scaffolds that mimic the native ECM in the human body [Citation9,Citation10]. However, the scaffolds have limitations for artificial oesophagus applications due to their low mechanical strength [Citation11]. To overcome this problem, we propose a hybrid technology involving sequential rings for reinforcement of the anastomosis site using a 3D-printing technique to produce a hybrid biomedical scaffold. Combining electrospinning and 3D-printing techniques could improve the ability to control the mechanical performances of the scaffolds. This hybrid scaffold showed excellent mechanical properties compared to pristine electrospun grafts, preserving the optimal fibrillary arrangement for initial cell attachment [Citation12].
Temporary implantation of the substitute in a natural bioreactor, such as the greater omentum, is beneficial [Citation2]. Angiogenesis is a fundamental process for wound healing and also prevents the grafts failure of transplanted artificial scaffold. However, once the artificial scaffold is transplanted in vivo, it should be alive in the initial stage through the diffusion of nutrients and oxygen, and that would be the main cause of graft failure [Citation13]. We chose the greater omentum as in vivo bioreactor because of its rich vascular network, and expected vascularized tissue growth before implantation. Newly grown vascular tissue in omentum was expected to prevent postoperative ischaemia and fibrosis.
The lumen of oesophagus is originally very small (approximately 2–3 mm in diameter); therefore, a major part of the lumen seemed to be closed after omentum culture in our results because of tissue growth inside the scaffold. However, most this obstruction was membranous. We implanted this scaffold after bougination with a small needle (1 cc syringe), which made the lumen patent. This procedure did not cause any clinical problems when we implanted this scaffold in a rat model. We used scaffold autotransplantation in a greater omentum culture, and these omentum-cultured scaffolds were more practical for oesophageal scaffold graft survival.
The use of PCL for scaffold fabrication is generally increasing. It is a Food and Drug Administration (FDA) approved biocompatible, bioresorbable and a low-cost synthetic polymer. PCL also has some rheological and viscoelastic properties that allow it to be formed from a wide range of scaffold fabrication technologies. One disadvantage of PCL, however, is its hydrophobic nature, resulting in poor wettability, lack of cell attachment and uncontrolled biological interactions with the material. To rectify this issue, surface modification techniques can alter the chemical and/or physical properties of the surface [Citation14].
The use of small animal models for circumferential oesophageal defects, particularly murine species, offers a number of advantages, including cost efficiency, the ability to adequately statistically power studies, the availability of genetic modification tools that facilitate mechanistic studies, and the possibility to evaluate multiple innate physiologic variables that cannot be mimicked in vitro. However, the small animal models used for circumferential oesophageal defects are technically challenging, and a great degree of expertise is required to perform surgical procedures in the murine oesophagus [Citation5,Citation12,Citation15,Citation16]. Although the PCL scaffold exhibited complete healing of surgically created circumferential defects by the second week, all rats died postoperatively within 15 days. The deaths occurred in conjunction with malnutrition and leakage of the proximal anastomosis site because of intraluminal obstruction by a foreign material. We speculate that these results are significantly related to the very small diameter of the rat oesophagus and the absence of peristaltic movement. The absence of peristaltic activity after adynamic scaffold implant most likely prevents normal oesophageal swallowing. Although it was possible to prevent coprophagy and bedding ingestion, nutritional support was limited by a diet of exclusively liquid foods, and it was impossible to prevent rats from ingesting their own hair. It was also difficult to control progressive obstruction by the discarded scaffold and suture material into the lumen. Lopes et al. [Citation15] performed semi-circumferential and circumferential oesophageal defects in a rat model and repaired them with porcine small intestinal submucosa patch grafts for partial oesophageal defects and tube grafts for circumferential defects. The survival rate was 0%, and all rats died between postoperative day 4 and 17 for the circumferential defect group, which was similar to our results. The partial defect group showed no signs of oesophageal dysfunction and returned to normal weight. In contrast, large animal models (i.e. dogs) with circumferential oesophageal defect reconstruction are technically easier to implement and permit the evaluation of technologies at their intended therapeutic physical dimensions. The size of the oesophagus is bigger, enabling easy surgical implantation and continuous food passage even without local peristalsis. Moreover, large animals can be fed only by intravenous parenteral nutrition up to four weeks after the implantation of the prosthesis [Citation17,Citation18]. Therefore, artificial oesophagus constructs with better mechanical properties and an alternative parenteral feeding model should be established to achieve optimal regeneration for small animal models. In conclusion, an omentum-cultured nano-structure scaffold reinforced by a 3D-printed ring is a more practical model with better vascularization for artificial neo-oesophagus reconstruction in a rat model. However, further studies on circumferential defect reconstruction in a rat model are still needed.
Disclosure statement
No potential conflict of interest was reported by the authors.
Additional information
Funding
References
- Sjöqvist S, Jungebluth P, Lim ML, et al. Experimental orthotopic transplantation of a tissue-engineered oesophagus in rats. Nat Commun. 2014;15:3562?3577.
- Poghosyan T, Gaujoux S, Sfeir R, et al. Bioartificial oesophagus in the era of tissue engineering. J Pediatr Gastroenterol Nut. 2011;52:S16–S17.
- Shen Q, Shi P, Gao M, et al. Progress on materials and scaffold fabrications applied to esophageal tissue engineering. Mater Sci Eng C Mater Biol Appl. 2013;33:1860–1866.
- Tan JY, Chua CK, Leong KF, et al. Esophageal tissue engineering: an in-depth review on scaffold design. Biotechnol Bioeng. 2012;109:1–15.
- Lv Q, Cao C, Zhang Y, et al. The preparation of insoluble fibroin films induced by degummed fibroin or fibroin microspheres. J Mater Sci Mater Med. 2004;15:1193–1197.
- Li J, Xu P, Chen H. Successful tracheal autotransplantation with two-stage approach using the greater omentum. Ann Thorac Surg. 1997;64:199–202.
- Londono R, Badylak SF. Regenerative medicine strategies for esophageal repair. Tissue Eng Part B Rev. 2015;21:393–410.
- Kuppan P, Sethuraman S, Krishnan UM. Tissue engineering interventions for esophageal disorders-promises and challenges. Biotechnol Adv. 2012;30:1481–1492.
- Eatemadi A, Daraee H, Zarghami N, et al. Nanofiber: synthesis and biomedical applications. Artif Cells Nanomed Biotechnol. 2016;44:111–121.
- Mohammadian F, Abhari A, Nejati-Koshki K, et al. New state of nanofibers in regenerative medicine. Artif Cells Nanomed Biotechnol. 2016;45:204–210.
- Giannitelli SM, Mozetic P, Trombetta M, et al. Combined additive manufacturing approaches in tissue engineering. Acta Biomater. 2015;24:1–11.
- Badylak S, Meurling S, Chen M, et al. Resorbable bioscaffold for esophageal repair in a dog model. J Pediatr Surg. 2000;35:1097–1103.
- Suh S, Kim J, Shin J, et al. Use of omentum as an in vivo cell culture system in tissue engineering. ASAIO J. 2004;50:464–467.
- Cipitria A, Skelton A, Dargaville TR, et al. Design, fabrication and characterization of PCL electrospun scaffolds—a review. J Mater Chem. 2011;21:9419–9453.
- Lopes MF, Cabrita A, Ilharco J, et al. Esophageal replacement in rat using porcine intestinal submucosa as a patch or a tube-shaped graft. Dis Esophagus. 2006;19:254–259.
- Chung EJ, Ju HW, Park HJ, et al. Three-layered scaffolds for artificial esophagus using poly(ε-caprolactone) nanofibers and silk fibroin: an experimental study in a rat model. J Biomed Mater Res. 2015;103:2057–2065.
- Takimoto Y, Nakamura T, Yamamoto Y, et al. The experimental replacement of a cervical esophageal segment with an artificial prosthesis with the use of collagen matrix and a silicone stent. J Thorac Cardiovasc Surg. 1998;116:98–106.
- Yamamoto Y, Nakamura T, Shimizu Y, et al. Intrathoracic esophageal replacement in the dog with the use of an artificial esophagus composed of a collagen sponge with a double-layered silicone tube. J Thorac Cardiovasc Surg. 1999;118:276–286.