Abstract
Colorectal cancer (CRC) is the third leading cause of cancer-related deaths worldwide. 5-fluorouracil (5-FU)-based chemotherapeutic regimens are routinely used for the treatment of patients with CRC. However, recurrence and chemotherapeutic drug resistance limit the survival rates of patients with CRC. DNA methylation participates in diverse cellular processes by regulating the transcription of a large number of genes expression, cell division, apoptosis, cell adhesion and differentiation, and metabolism, thus it might mediate chemoresistance. Using an Illumina Infinium HD Assay, DNA methylation levels in a human 5-FU-resistant HCT-8 CRC cell line (HCT-8/FU) and its progenitor cell line HCT-8 were analysed. A total of 16,580 differentially methylated genes were identified, of which 8885 were hypermethylated and 7695 were hypomethylated in resistant cells. Among these genes, NME2 (nucleoside diphosphate kinase 2) exhibited a significant difference in methylation between cell lines and has known roles in gastric cancer and breast cancer; accordingly, we hypothesized that it plays a role in acquired resistance in CRC. Knockdown of NME2 restored 5-FU sensitivity in 5-FU-resistant CRC cells, reduced cell survival and increased cell apoptosis; and overexpression of NME2 in HCT-8 cells results in the acquisition of resistance to 5-FU, this alteration enhanced HCT-8 cells growth abilities and reduced apoptosis. These findings suggest that NME2 mediates chemoresistance to 5-FU in CRC and that specific NME2 inhibition could optimize 5-FU-based chemotherapy of CRC.
Introduction
Colorectal cancer (CRC) is the third leading cause of cancer-related deaths in the world [Citation1] and the second most common cancer in the Western hemisphere [Citation2]. The burden of CRC is increasing in the Asia-Pacific region [Citation3]. Its prognosis has gradually improved owing to advances in surgery, radiotherapy and chemotherapy. Despite improvements in the efficacy of therapeutics, acquired resistance to chemo- or radiotherapy remains an obstacle in CRC treatment, and the survival rate of patients with CRC is still very low, with a 5-year survival rate of ∼64% [Citation4]. Therefore, a better understanding of chemoresistance and the identification of predictive biomarkers have important clinical relevance for CRC patients. DNA methylation regulates cell differentiation, proliferation and invasion and participates in tumorigenesis and other processes [Citation5]. In contrast to genetic mechanisms, e.g. DNA sequence changes, epigenetics refers to the regulation of gene expression, often via histone modifications [Citation6]. A global change in DNA methylation is a hallmark of human cancer, which is often characterized by selective hypermethylation confined to gene promoters. Solid evidence indicates that epigenetic marks could be used as prognostic and predictive biomarkers and can explain resistance to chemotherapy in oncology [Citation7]. Accordingly, methylome analyses may facilitate the development of improved treatment strategies for patients with colorectal carcinoma. Tumours preferentially take up uracil; accordingly, 5-FU uracil (an analogue of pyrimidine) has been synthesized as a means of interfering with nucleic acid synthesis and thus slowing tumour growth. In the past decade, 5-FU-based chemotherapy regimens have been used for the routine treatment of patients with CRC in clinical settings [Citation8–10], resulting in a significant reduction in mortality (relative reduction, about 20%; absolute reduction in mortality, about 10%) in patients with CRC [Citation11]. Despite the increased survival rate of patients with CRC, many patients administered 5-FU-based chemotherapy experience recurrence. This recurrence is predominantly attributed to the development of chemoresistance to 5-FU-based treatments. Resistance is a complex and multifaceted problem involving multiple molecular pathways that facilitate increased 5-FU efflux, evasion of apoptotic pathways, bypass of replication checkpoint, increased cell proliferation and increased DNA damage repair [Citation12,Citation13]. A number of targets have been implicated in 5-FU resistance during CRC. In general, a better understanding of the molecular mechanisms that underlie chemotherapeutic resistance is required for the development of effective 5-FU-based therapeutic strategies.
The NME family of genes consists of 10 members, also known as NM23 genes; these genes are associated with invasion to local tissues [Citation13]. Among members of this gene family, NME1 and NME2 have been extensively studied in the context of carcinogenesis, especially metastasis formation. Nucleoside diphosphate kinase 2 (NME2), on chromosome 17q21 [Citation14,Citation15], encodes the B subunit of the nucleoside diphosphate (NDP) kinase. There is increasing evidence that NME2 is differentially expressed in malignant tumours and may, therefore, be involved in the pathology of carcinomas. Its product inhibits the metastasis of breast cancer and melanoma cells, and a decrease in its expression is correlated with a greater metastatic potential in these cancers [Citation16]. However, NME2 overexpression is also associated with a poor prognosis in neuroblastoma and osteosarcoma [Citation17,Citation18]. Moreover, the NME2 gene is not associated with the metastasis of endometrial hepatocellular and thyroid carcinoma [Citation19]. These apparently conflicting results suggest that NME2 may have differential effects depending on the type of cancer, including differences in local invasion or metastasis. However, little is known about the role of NME2 in the modulation of chemosensitivity in cancer cells. For this reason, we characterized the role of NME2 in chemoresistance regulation. In particular, we investigated the role of NME2 in the regulation of chemoresistance in CRC, using cell-based assays. Human 5-FU-resistant HCT-8 CRC cell line (HCT-8/FU) and its progenitor cell line HCT-8 were used to analyse the methylation. The results showed that the methylation of NME2 was lower in HCT-8/FU than in HCT-8 cells and NME2 expression exhibited the inverse pattern. Knockdown of NME2 in HCT-8/FU cells inhibited proliferation and survival and promoted apoptosis. These findings suggest that NME2 mediates chemoresistance to 5-FU in CRC. Thus, we speculate that NME2 might represent a promising therapeutic target for the treatment of drug-resistant cancers.
Results
5-FU toxicity profile of HCT-8 and HCT-8/FU cell lines
To determine the mechanism underlying 5-FU resistance, an in vitro cell model using HCT-8 and HCT-8/FU cells was established. The single cell-derived clones for the HCT-8 and HCT-8/FU cell lines were obtained by a limiting dilution strategy. The 5-FU toxicity profiles of the HCT-8 and HCT-8/FU cell lines were assessed. The 50% inhibitory concentrations of 5-FU in HCT-8 and HCT-8/FU cells were 15.32 and 71.79 μg/ml, respectively, and the resistance index was 4.69 (p < .05). The dose–response curve is shown in .
Figure 1. Inhibitory effects of various concentrations of 5-FU on HCT-8 and HCT-8/FU cells. Cell viability, as assessed by the CCK-8 assay, was determined 24 h after the exposure of HCT-8 and HCT-8/FU cells to increasing concentrations of 5-FU. Results represent the average of triplicate wells and are representative of three independent experiments.
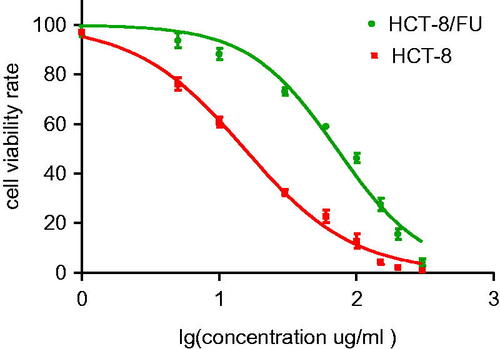
Methylation analysis
To examine differential methylation between HCT-8 and the resistant cell line HCT-8/FU, the Illumina Infinium Human Methylation 850 BeadChip array (Illumina, Inc., San Diego, CA) was used to analyse methylation levels; the array evaluates more than 850,000 cytosine-phosphate-guanine (CpG) sites, with complete coverage of CPG islands in 1st exons, 5' UTRs, TSS1500 and TSS200. The beta value (β) is an indicator of the methylation level at a CpG locus and is defined as the ratio of intensities between methylated and unmethylated alleles. A difference in β of ≥0.5 or ≤0.5 was set as the threshold for statistical significance. A total of 44,833 differentially methylated CPG sites were identified. These sites belonged to 16,580 genes, of which 8885 were hypermethylated and 7695 were hypomethylated in resistant cells. To further explore the differentially methylated gene between HCT-8 and HCT-8/FU, we carried out Gene Ontology (GO) analyses and pathway enrichment analyses. For the GO analyses, all the differentially methylated genes were annotated to three major GO categories: biological process (BP), cell component (CC) and molecular function (MF). GO CC analysis revealed genes significantly enriched in cell, cell part and organelle; for BP, there was significant enrichment in cellular process, metabolic process and single-organism process; For MF, there was enrichment in binding and catalytic activity (Supplementary Figure 1). Moreover, we found the differentially methylated genes that were significantly enriched in the calcium signalling pathway, focal adhesion, cell adhesion molecules pathways using KEGG pathway enrichment analysis (Supplementary Figure 2). We then compared these enriched genes with potential cancer happen and progress genes, NME2 was mainly involved in three pathways of metabolism, synthesis and interconversion of nucleotide di- and triphosphates and metabolism of nucleotides, according to previous reports, NME2 was of particular interest, perhaps is related to the chemoresistance to 5-FU in CRC.
Validation of NME2 methylation in HCT cells
To experimentally confirm the results of the methylation chip analysis, we performed pyrosequencing to evaluate the NME2 methylation level in HCT-8/FU cells and parental HCT-8 cells. The same trend was observed in the methylation chip and pyrosequencing. The methylation levels of NME2 were lower in HCT-8/FU cells than that in the HCT-8 cells ().
Elevated NME2 expression in HCT cells
To investigate the functional importance of NME2 methylation for transcriptional activity, we performed a quantitative real-time PCR (qRT-PCR) and Western Blotting analysis using HCT-8/FU cells and parental HCT-8 cells. As expected, the expression of NME2 was much higher in HCT-8/FU cells than in HCT-8 cells, as shown in . These results are consistent with the notion that hypomethylation leads to greater expression of NME2 in CRC cells.
Figure 3. NME2 expression level was elevated in HCT-8/FU cells. (A) qRT-PCR analysis was performed for NME2, which was differentially expressed between HCT-8 and HCT-8/FU cells. The expression level of NME2 was normalized to the level in HCT-8 cells. (B) Western Blotting analysis was performed for NME2, Western Blotting results were consistent with qRT-PCR, showed that the NME2 protein level was also significantly upregulated in HCT-8/FU cells compared with the HCT-8 cells. Statistical analyses were carried out using t-test, *p < .05.
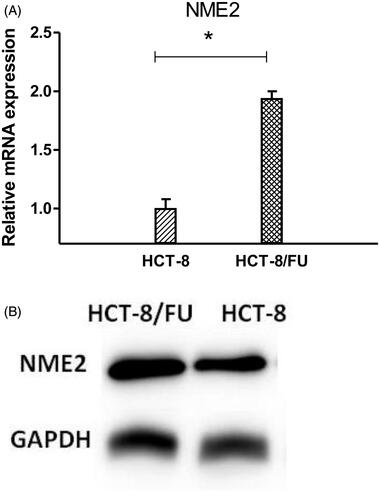
NME2 silencing weakened the resistance of CRC cells to 5-FU treatment
To confirm that NME2 confers resistance to 5-FU, specific SiRNAs targeting human NME2 (SiRNA1, 2) and non-silencing control SiRNA, i.e. a scramble control (SiCtrl), were used to infect CRC cells, which exhibit high endogenous NME2 levels. qRT-PCR and Western Blotting analyses revealed that NME2 mRNA and protein expression levels were effectively suppressed in SiRNA-treated HCT-8/FU cells compared with that in the scramble control-transfected cells. The knockdown ratio was approximately 64% in HCT-8/FU cells (). Cell survival assays (CCK-8) were performed using HCT-8/FU cells following treatment with 5-FU. As expected, the silencing of NME2 in HCT-8/FU cells resulted in a statistically significant increase in sensitivity to 5-FU. The 50% inhibitory concentrations of 5-FU in HCT-8/FU and the silencing of NME2 in HCT-8/FU cells were 52.6 and 35.00 μg/ml, respectively (p < .05); an increase was not observed in the SiCtrl-silenced cells (), suggesting that NME2 is involved in the resistance of CRC cells to 5-FU. To further enhance the efficiency NME2 interference, we additionally used siRNA2 but observed a similar knockdown ratio and similar growth inhibition.
Figure 4. Effect of NME2 knockdown on cell survival. HCT-8/FU cells transfected with SiRNA targeting NME2 and untransfected cells (C) were cultured for 24 h. (A) Relative levels of NME2 mRNA were analysed by qRT-PCR in HCT-8/FU cells after different siRNA treatments. (B) Protein level of NME2 was analysed by Western Blotting in HCT-8/FU cells after different SiRNA treatments. (C) Inhibitory effects of various concentrations of 5-FU on HCT-8/FU cells by SiR1 treatments. (D–G) NME2 was silenced in HCT-116 and LOVO cells. (D,F) Relative levels of NME2 mRNA by qRT-PCR in HCT-116 and LOVO cells after different SiRNA treatments; (E,G) Inhibitory effects of various concentrations of 5-FU on HCT-116 and LOVO cells by SiR1 treatments. *p < .05, **p < .01, ns represents no statistic difference.
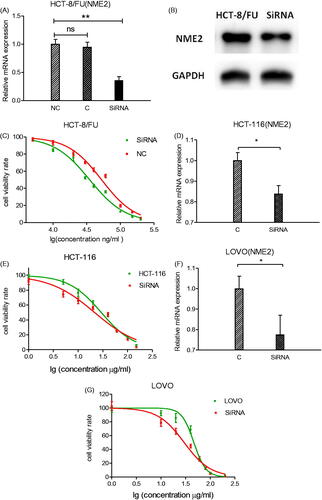
To examine whether NME2 plays a role in chemoresistance in other CRC cells, two other CRC cell lines (HCT-116 and LOVO) were chosen and NME2 was silenced by targeted SiRNA. As shown in , NME2 expressions were remarkably suppressed in target SiRNA group of HCT-116 and LOVO cells. CCK-8 assay showed that the 50% inhibitory concentrations of 5-FU were significantly decreased when silencing of NME2 in HCT-116 (21.52 vs. 29.39 μg/ml) and LOVO (29.55 vs. 46.99 μg/ml), respectively (). Similar increase in sensitivity to 5-FU was observed in the HCT-116 and LOVO with HCT-8 cells. All these data indicate that in CRC cells, NME2 may involve in the resistance of CRC cells to 5-FU.
Overexpression of NME2 enhanced the resistance of HCT-8 to 5-FU treatment
To further conform NME2 mediated drug resistance in CRC cells, a NME2 overexpression stable HCT-8 cell line was established by using lentivirus system, with GFP overexpression stable cells serving as the control. Next, qRT-PCR and Western Blotting was used to analysed the expression of NME2 in HCT-8 cell line, as shown in , NME2 expression was significantly higher in the groups infected with lentivirus-NME2 than that in lentivirus-GFP. Furthermore, CCK8 assay was performed in NME2 overexpression HCT-8 cells, as shown in . Overexpresion of NME2 significantly increased cell resistance to 5-FU (the 50% inhibitory concentrations of 5-FU were 53.18 and 27.54 μg/ml, respectively, p < .05, ). Together, NME2 upregulation significantly enhanced the resistance of HCT-8 to 5-FU treatment.
Figure 5. Effect of NME2 overexpression on cell survival. HCT-8 cell line with NME2 overexpression and with stable GFP overexpression served as a control. (A) Relative levels of NME2 mRNA were analysed by qRT-PCR in HCT-8 cells after different treatments. (B) Protein level of NME2 was analysed by Western Blotting in HCT-8 cells after different treatments. (C) Inhibitory effects of various concentrations of 5-FU on HCT-8 cells by different treatments. **p < .01.
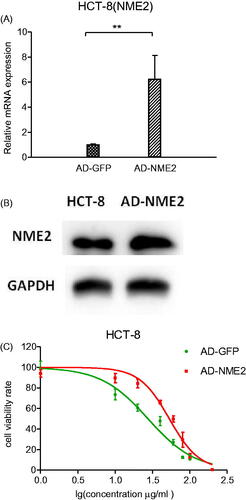
Effect of NME2 on apoptosis
The effect of NME2 on apoptosis was examined by flow cytometry. The percentages of spontaneous and 5-FU-induced apoptotic cells were significantly higher in NME2 knockdown cells than in negative control cells (), on the contrary, the percentages of spontaneous and 5-FU-induced apoptotic cells were significantly lower in NME2 overexpression cells than that in control cells (). These data further confirmed that NME2 protects against apoptosis in CRC.
Figure 6. Effects of NME2 on cell apoptosis based on a flow cytometry analysis. (A) Knockdown of NME2 in HCT-8/FU cells increased the apoptosis rate. Various concentrations of 5-FU resulted in differences in the cell apoptosis rate. (a–c) HCT-8/FU cells were treated with non-silencing control siRNA and various concentrations of 5-FU. (d–f) HCT-8/FU cells were treated with a specific siRNA targeting human NME2 (siRNA1) and various concentrations of 5-FU. Differences in the cell apoptosis rate among treatments, as detected by flow cytometry (FCM). Total apoptosis was defined as early apoptosis plus late apoptosis. (C) Overexpression of NME2 in HCT-8 cells decreased the apoptosis rate. (B,D) The apoptosis rate is presented mean ± SEM of three independent experiments. *p < .05, **p < .01 (Student’s t-test).
Discussion
In this study, we examined the genes associated with acquired resistance to 5-FU to enable the development of targeted therapies for CRC. We screened for differences between the HCT-8/FU and HCT-8 cell lines, using a methylation chip analysis. A total of 45,833 differentially methylated regions were identified, of which 25,248 were hypermethylated and 20,585 were hypomethylated. These differentially methylated regions were in 16,580 genes, of which 8885 were hypermethylated and 7695 were hypomethylated. The function of the differentially methylated genes was annotated based on references GO and pathway analyses. Based on these results, NME2 was identified as being involved in a potential resistance mechanism. Finally, we performed qRT-PCR Western Blotting and pyrosequencing to validate the NME2 mRNA expression, protein expression and methylation chip analysis results, respectively. 5-FU is a major chemotherapy drug used for CRC, it is an analogue of the pyrimidine uracil and represents a rationally designed chemotherapy agent based on the molecular biology of tumours [Citation12]. However, drug resistance has become a key challenge in cancer treatment. Resistance has been linked to numerous mechanisms, including altered drug metabolism, bypass of oncogenic signalling pathways, suppression of apoptotic cascades and an enhanced DNA repair capacity [Citation20,Citation21]. Recently, next-generation sequencing, SNP arrays, transcriptome analyses and other technologies have been used to identify the mechanisms underlying drug resistance. Remarkably, new evidence has revealed marked differences in both gene-specific and genome-wide epigenetic profiles between drug-resistant cells and sensitive cells. Nonetheless, the role of epigenetic modifications in CRC as well as the clinical relevance of parameters related to methylation are unclear. Few studies have addressed the role of epigenetics in hepatocarcinogenesis [Citation22] and there is even less information regarding the roles of DNA methylation and drug resistance in CRC.
Using a DNA methylation chip, we described the landscape of aberrantly methylated promoters of potential genes involved in CRC and investigated the relationship between NME2 methylation and acquired resistance to 5-FU in CRC. NME2 was initially identified as a metastasis suppressor gene, but its ability to block local invasion and remote metastasis appear to be cell type-specific. For example a study using tissue chip technology and immunohistochemistry demonstrated that NME2 expression is associated with the level of differentiation in gastric cancer cells as well as metastasis to the lymph nodes and reduced rates of proliferation, migration and invasion through the collagen matrix [Citation23]. Recently, NME2 was found to be highly expressed in patients with CRC. The expression patterns of 24 5-FU pathway genes, including NME2, in colon cancer were characterized using paired non-tumour and tumour samples from 52 patients with CRC and these results suggested that NME2 has a crucial role in the 5-FU pathway [Citation24]. For this reason, we further analysed NME2 methylation and expression profiles in relation to chemoresistance. For the first time, we demonstrated that NME2 plays a crucial role in 5-FU-resistant CRC cells. We observed that NME2 expression was higher in 5-FU-resistant HCT-8/FU CRC cells than in 5-FU-sensitive HCT-8 CRC cells. In addition, our results showed that the knockdown of NME2 increases sensitivity to 5-FU and apoptosis, overexpression of NME2 decreases sensitivity to 5-FU and apoptosis. These results suggest that NME2 plays critical roles in many aspects of cancer biology, including cancer cell proliferation, survival, apoptosis, drug resistance and metastasis. However, the factors that regulate these diverse NME2 functions in CRC cells are unknown. It is possible that NME2 activity depends on up- and downstream molecules that interact with NME2 in a given cell type. Many molecules interact with or regulate NME2, such as epidermal growth factor receptor, erbB-2 and sex steroid receptor in ovarian carcinomas [Citation25]. The relationship between NME2 and genes in the myc family appears to be complex. myc is an oncogene involved in diverse cellular processes via the transcriptional regulation of a large number of loci involved in gene expression, cell division, apoptosis, cell adhesion, stem cell self-renewal, differentiation, metabolism and drug resistance [Citation26–28]. NME2 is a transcriptional regulator of the oncogene myc [Citation29] and interacts with G-quadruplex DNA in the nuclease hypersensitive element of the c-myc promoter to induce c-myc expression [Citation30], which is responsible for the transcriptional activation of the proto-oncogene c-myc, when NME2 has a high levels of gene expression would results in up-regulation of myc transcription [Citation31]. These results imply that NME2 may be associated with resistance to 5-FU in CRC via the regulation of myc expression.
Among the regulated genes, the methylation of CBR1, UGT2A1 and RFC3 were all downregulated (β difference >0.3), indicating that these genes might involve in 5-FU chemoresistance to in CRC. Carbonyl reductase 1 (CBR1), a member of the short-chain dehydrogenase/reductase superfamily, catalyses the reduction of the ketone, aldehyde and C=C bond of ONE into 4-hydroxy-2-nonenal (HNE), 4-oxo-2-nonenol and 4-oxononanal, respectively [Citation32]. This gene has been reported to mediate DOX reduction and suggested to develop the chemoresistance in cancer cells [Citation33]. UGT2A1 belongs to UDP-glycosyltransferase family, which catalyse biotransformation reactions in which lipophilic substrates are conjugated with glucuronic acid to increase water solubility and enhance excretion. They are of major importance in the conjugation and subsequent elimination of potentially toxic xenobiotics and endogenous compounds, UGT2A1 exhibited highest expression in the lung, colon and liver and other tissues [Citation34], hence, it may play an important role in drug resistance. RFC3 mainly functioned in DNA replication, nucleotide excision repair and mismatch repair. It may affect the efficacy of drugs similar to paclitaxel’s and it also reported to over-expressed in many kinds of cancers. When RFC3 gene was silenced, ovarian tumour cells proliferation were greatly suppressed [Citation35].
In conclusion, we demonstrated, for the first time that the knockdown of NME2 increases sensitivity to 5-FU and apoptosis in vitro, indicating that NME2 is a target molecule involved in 5-FU induced chemoresistance. Thus, the specific inhibition of NME2 expression in future therapeutic regimens could optimize 5-FU-based chemotherapy in CRC.
Materials and methods
Cell lines and culture
Human CRC cell lines HCT-8 and HCT-8/FU were obtained from Bogu Biotech (Shanghai, China). HCT-8 and HCT-8/FU cells were cultured in RPMI 1640 (Gibco, Carlsbad, CA) and DMEM (Gibco, Carlsbad, CA), respectively, supplemented with 10% (v/v) heat-inactivated foetal bovine serum (FBS), 100 U/ml penicillin and 100 μg/ml streptomycin (Life Technologies, Grand Island, NY). Cells were grown in 5% CO2 at 37 °C.
Cytotoxicity assay
HCT-8 and HCT-8/FU cells were seeded in a 96-well plate at a density of 5 × 104 cells per well. After incubation for 24 h, the medium was replaced with fresh medium containing 5-FU at concentrations of 1, 5, 10, 30, 60, 100, 150, 200 and 300 μg/ml in the presence of 10% FBS for an additional 24 h of incubation. Cell viability was examined using the CCK-8 assay. The absorbance of each well was measured at 450 nm on a microplate reader. The proliferation rate was defined as the percentage of surviving cells in each group compared with that in the untreated group.
DNA methylation chip analysis
Approximately 500 ng of gDNA for the manual assay or 1000 ng of gDNA for the automated assay per sample was used as input material for bisulphite conversion. DNA was treated with sodium bisulphite using an Illumina-specified Bisulfite Conversion Kit (Illumina, San Diego, CA). After treatment, unmethylated cytosines are converted to uracil, while methylated cytosines remain unchanged. The BCD samples were denatured and neutralized for amplification. The denatured DNA was isothermally amplified in an overnight step. The whole-genome amplification uniformly increased the amount of DNA by several thousand-fold, without substantial amplification bias. The amplified product was fragmented by a controlled enzymatic process that does not require gel electrophoresis. The process uses end-point fragmentation to avoid over-fragmentation of the sample. After isopropanol precipitation, the fragmented DNA was collected by centrifugation at 4 °C. The precipitated DNA was resuspended in hybridization buffer. The fragmented, resuspended DNA samples were added to BeadChips. The BeadChips were incubated in the Illumina Hybridization Oven (Illumina, San Diego, CA) to hybridize the samples to the BeadChips. Twelve samples were applied to each BeadChip, which were separated using an IntelliHyb seal (Illumina, San Diego, CA). The prepared BeadChip was incubated overnight in the Illumina Hybridization Oven. The amplified and fragmented DNA samples annealed to locus-specific 50-mers (covalently linked to one of over 500,000 bead types) during hybridization. Two bead types correspond to each CpG locus for Infinium I assays: one bead type corresponds to the methylated state (C) and another bead type to the unmethylated (T) state of the CpG site. One bead type corresponds to each CpG locus for Infinium II assays. Unhybridized and non-specifically hybridized DNA was washed, and the chip was prepared for staining and extension in capillary flow-through chambers. Single-base extension of the oligos on the BeadChip, using the captured DNA as a template, incorporates detectable labels on the BeadChip and was used to determine the methylation level of the query CpG sites. The Illumina HiScan or iScan System was used to scan the BeadChip using a laser to excite the fluorophore of the single-base extension product on the beads. A scanner was used to record high-resolution images of the light emitted from the fluorophores.
Total RNA extraction
RNA was extracted from cells using TRIzol reagent (Takara Bio, Inc., Otsu, Japan), followed by the addition of 400 μl of chloroform. The mixture was then centrifuged at 12,000×g for 15 min at 4 °C, and the upper layer was transferred to a clean Eppendorf tube (Thermo Fisher Scientific Co., Waltham, MA) and supplemented with an equal volume of isopropanol. The mixture was centrifuged at 12,000×g for an additional 10 min at 4 °C. After washing the precipitate with 70% precooled ethanol, total RNA was dissolved in RNase-free water. RNA purity was checked using the NanoPhotometer spectrophotometer (Implen, Westlake Village, CA). The RNA concentration was measured using the Qubit RNA Assay Kit on a Qubit version 2.0 fluorometer (Life Technologies, Carlsbad, CA), and integrity was assessed using the RNA Nano 6000 Assay Kit and the Agilent Bioanalyzer 2100 System (Agilent Technologies, Santa Clara, CA).
Quantitative reverse transcription (qRT)-PCR analysis
Samples were reverse-transcribed at 42 °C for 5 min, followed by 10 s at 95 °C and PCR cycling conditions consisted of 5 s at 95 °C and 34 s of annealing at 60 °C. Approximately, 2 μg of mRNA sample was amplified by PCR using a One Step SYBR PrimeScript™ RT-PCR Kit II according to the manufacturer’s instructions (TaKaRa BioTechnology, Kusatsu, Japan). SYBR Green-based qPCR was then performed using the ABI ViiA 7 Real-Time PCR System (Applied Biosystems, Foster City, CA). GAPDH was used as the endogenous control and all reactions were performed in triplicate. Relative gene expression was calculated using the comparative cycle threshold (2−ΔΔCT) method.
Western Blot analysis
Total protein from cells was extracted in 1*SDS buffer and the protein concentration was calculated with a Pierce BCA protein assay kit (Pierce, Rockford, IL). Equivalent quantities of proteins were separated by 15% SDS–PAGE gels and transferred to PVDF membranes (Millipore, Bedford, MA). Following blocking with 5% non-fat milk at room temperature for 2 h, the membranes were incubated with primary antibodies (NME2 and GAPDH, Santa Cruz, CA) at 4 °C overnight, and washed three times in TBST followed by incubation with the secondary corresponding goat anti-mouse horseradish peroxidase (HRP)-conjugated antibody for 2 h at room temperature. The protein levels were normalized to those of GAPDH.
Pyrosequencing validation
Methylation results were validated using a pyrosequencing assay for a subset of samples previously analysed by the Illumina Infinium Human Methylation850 array (Illumina, San Diego, CA). A minimum of 500 ng of DNA was converted using the EZ DNA Methylation-Gold (Zymo Research Corporation, Irvine, CA) bisulphite conversion kit, following the manufacturer’s instructions. Specific sets of primers for PCR amplification and sequencing were designed using PyroMark Assay Design version 1.0.11 (Qiagen, Hilden, Germany).
Pyrosequencing primer sequences (5'-GTTGAGTAAAGTTTTAGGTT-3′) and PCR primer sequences (forward 5′-GGTAGGGTAGAGGTAGGATTAGATG-3′; reverse 5′-CTACTTAAACCCTAATTAAACCTCRAC-3′) were designed, when possible, to hybridize with CpG-free sites to ensure methylation-independent amplification. PCR was performed under standard conditions with biotinylated primers and the PyroMark Vacuum Prep Tool (Biotage AB, Uppsala, Sweden) was used to prepare single-stranded PCR products, according to the manufacturer’s instructions. A total of 50 μl volume was amplified with 2 μl bisulphite-converted DNA, 1 × Pyromark PCR master Mix 11.5 μl, 35.5 μl RNase-free water, 1 ul forward primer and 1 μl 5′biotinylated-reverse primer. Thermal cycling conditions included an initial denaturation at 95 °C for 3 min, followed by 35 cycles of denaturation (25 s at 94 °C), annealing (25 s at 60 °C) and extension (25 s at 72 °C), and then final extension at 72 °C for 5 min. PCR products were observed on 2% agarose gels before pyrosequencing. Reactions were performed using the PyroMark Q96 System (Qiagen, Hilden, Germany) with appropriate reagents and protocols.
Cell transfection
Cells were seeded in a 96-well plate at a density of 5 × 104 cells per well. Transfections were performed using cells obtained at 70% confluence with Lipofectamine Plus reagent according to the manufacturer’s instructions (Invitrogen, Carlsbad, CA). siRNA targeting NME2 and control siRNA were synthesized and purchased from GenePharma, Shanghai, China (NME2 siRNA1: Sense
5′-GAAGACAUUGCUGAACAAATT-3′, Antisense
5′-UUUGUUCAGCAAUGUCUUCTT-3′; NME2 siRNA2: Sense
5′-GACCAAUCCAGCAGAUUCATT-3′, Antisense
5′-UUUCAGGUCAAUGUAGUGCTT; Negative control siRNA: Sense
5′-UUCUCCGAACGUGUCACGUTT-3′, Antisense
5′-ACGUGACACGUUCGGAGAATT-3′).
siRNA transfection was performed using Lipofectamine RNAi Max Transfection Reagent (Invitrogen, Carlsbad, CA) according to the manufacturer’s instructions, The knockdown efficiency was assessed by qRT-PCR using mRNAs and Western Blotting using proteins collected 24 and 48 h post-transfection; and HCT-8 were infected into GV492 vector (Genechem, Shanghai, China) . Then, the lentiviral packaging plasmid was infected into HCT-8 cells with enhance infection solution according to the manufacturer’s protocol. The infected efficiency was samely assessed by qRT-PCR and Western Blotting at 48 or 72 h after infection.
Cell apoptosis analysis
FITC Annexin V/PI apoptosis detection was performed using the FITC Annexin V/PI Apoptosis Detection Kit (KeyGEN, Nanjing, China). Apoptosis (expressed as a percentage) was determined by flow cytometry according to the manufacturer’s instructions.
Statistical analysis
The methylation levels of CpGs were presented as the beta. The differences in the HCT-8 and the HCT-8/FU cells were provided as delta beta. Statistical analysis was performed by applying the SPSS statistical package version 16.0 (SPSS Inc., Chicago, IL).Paired t-tests were used to compare the differences in expression between the HCT-8 and HCT-8/FU cells. A p value < .05 was considered to be statistically significant. The results were presented using GraphPad Prism software (GraphPad Software, Inc., La Jolla, CA).
Shaojia_et_al._Supplementary_Material.pdf
Download PDF (377.4 KB)Disclosure statement
The authors declare that there are no conflicts of interest.
Additional information
Funding
References
- Siegel R, Ma J, Zou Z, et al. Cancer statistics, 2014. CA Cancer J Clin. 2014;64:9–29.
- Rad R, Cadiñanos J, Rad L, et al. A genetic progression model of braf-induced intestinal tumorigenesis reveals targets for therapeutic intervention. Cancer Cell. 2013;24:15–29.
- Sankaranarayanan R, Ramadas K, Qiao Y. Managing the changing burden of cancer in Asia. BMC Med. 2014;12:3.
- Velde CJHVD, Boelens PG, Borras JM, et al. EURECCA colorectal: multidisciplinary management: European consensus conference colon & rectum . Eur J Cancer. 2014;50:1.e1–1e.34.
- Portela A, Esteller M. Epigenetic modifications and human disease. Nat Biotechnol. 2010;28:1057–1068.
- Hu Q, Baeg GH. Role of epigenome in tumorigenesis and drug resistance. Food Chem Toxicol. 2017;109:663–668.
- Heyn H, Esteller M. DNA methylation profiling in the clinic: applications and challenges. Nat Rev Genet. 2012;13:679.
- Yong WM, Lee S, Park BW, et al. S-1 combined with docetaxel following doxorubicin plus cyclophosphamide as neoadjuvant therapy in breast cancer: phase II trial. BMC Cancer. 2013;13:1–7.
- Von MG, Rezai M, Fasching PA, et al. Survival after adding capecitabine and trastuzumab to neoadjuvant anthracycline-taxane-based chemotherapy for primary breast cancer (GBG 40–GeparQuattro). Ann Oncol. 2014;25:81.
- Iwata H, Sato N, Masuda N, et al. Docetaxel followed by fluorouracil/epirubicin/cyclophosphamide as neoadjuvant chemotherapy for patients with primary breast cancer. Jpn J Clin Oncol. 2011;41:867–875.
- Francini G, Petrioli R, Lorenzini L, et al. Folinic acid and 5-fluorouracil as adjuvant chemotherapy in colon cancer. Gastroenterology. 1994;106:899.
- Gao J, Qiu Y, Liu S, et al. Knockdown of EpCAM enhances the chemosensitivity of breast cancer cells to 5-fluorouracil by downregulating the antiapoptotic factor Bcl-2. PLoS One. 2014;9:e102590.
- Boissan M, Dabernat S, Peuchant E, et al. The mammalian Nm23/NDPK family: from metastasis control to cilia movement. Mol Cell Biochem. 2009;329:51–62.
- Stahl JA, Leone A, Rosengard AM, et al. Identification of a second human nm23 gene, nm23-H2. Cancer Res. 1991;51:445.
- Veenstra M. The N-myc and c-myc downstream pathways include the chromosome 17q genes nm23-H1 and nm23-H2. Oncogene. 2002;21:2097.
- Flørenes VA, Aamdal S, Myklebost O, et al. Levels of nm23 messenger RNA in metastatic malignant melanomas: inverse correlation to disease progression. Cancer Res. 1992;52:6088–6091.
- Oda Y, Naka T, Takeshita M, et al. Comparison of histological changes and changes in nm23 and c-MET expression between primary and metastatic sites in osteosarcoma: a clinicopathologic and immunohistochemical study. Hum Pathol. 2000;31:709–716.
- Leone A, Seeger RC, Hong CM, et al. Evidence for nm23 RNA overexpression, DNA amplification and mutation in aggressive childhood neuroblastomas. Oncogene. 1993;8:855–865.
- Iizuka N, Oka M, Noma T, et al. NM23-H1 and NM23-H2 messenger RNA abundance in human hepatocellular carcinoma. Cancer Res. 1995;55:652–657.
- Dannenberg JH, Berns A. Drugging drug resistance. Cell. 2010;141:18–20.
- Turner NC, Reisfilho JS. Genetic heterogeneity and cancer drug resistance. Lancet Oncol. 2012;13:e178.
- Song MA, Tiirikainen M, Kwee S, et al. Elucidating the landscape of aberrant DNA methylation in hepatocellular carcinoma. PLoS One. 2013;8:e55761.
- Liu Y, Yang A, Wei L, et al. NME2 reduces proliferation, migration and invasion of gastric cancer cells to limit metastasis. PLoS One. 2015;10:e0115968.
- Kidd EA, Yu J, Li X, et al. Variance in the expression of 5-fluorouracil pathway genes in colorectal cancer. Clin Cancer Res. 2005;11:2612–2619.
- Mandai M, Konishi I, Koshiyama M, et al. Expression of metastasis-related nm23-H1 and nm23-H2 genes in ovarian carcinomas: correlation with clinicopathology, EGFR, c-erbB-2, and c-erbB-3 genes, and sex steroid receptor expression. Cancer Res. 1994;54:1825.
- Nie Z, Hu G, Wei G, et al. c-Myc is a universal amplifier of expressed genes in lymphocytes and embryonic stem cells. Cell. 2012;151:68–79.
- Soucek L, Evan GI. The ups and downs of Myc biology. Curr Opin Genet Dev. 2010;20:91–95.
- Fernandez PC, Frank SR, Wang L, et al. Genomic targets of the human c-Myc protein. Genes Dev. 2003;17:1115–1129.
- Berberich SJ, Postel EH. PuF/NM23-H2/NDPK-B transactivates a human c-myc promoter-CAT gene via a functional nuclease hypersensitive element. Oncogene. 1995;10:2343–2347.
- Thakur RK, Kumar P, Halder K, et al. Metastases suppressor NM23-H2 interaction with G-quadruplex DNA within c-MYC promoter nuclease hypersensitive element induces c-MYC expression. Nucleic Acids Res. 2009;37:172.
- Bouvard C, Lim SM, Ludka J, et al. Small molecule selectively suppresses MYC transcription in cancer cells. Proc Natl Acad Sci USA. 2017;114:3497–3502.
- Doorn JA, Maser E, Blum A, et al. Human carbonyl reductase catalyzes reduction of 4-oxonon-2-enal. Biochemistry. 2004;43:13106–13114.
- Matsunaga T, Kezuka C, Morikawa Y, et al. Up-regulation of carbonyl reductase 1 renders development of doxorubicin resistance in human gastrointestinal cancers. Biol Pharm Bull. 2015;38:1309–1319.
- Bushey RT, Chen G, Blevins-Primeau AS, et al. Characterization of UDP-glucuronosyltransferase 2A1 (UGT2A1) variants and their potential role in tobacco carcinogenesis. Pharmacogenet Genom. 2011;21:55–65.
- Shen H, Xu J, Zhao S, et al. ShRNA-mediated silencing of the RFC3 gene suppress ovarian tumor cells proliferation. Int J Clin Exp Pathol. 2015;8:8968–8975.