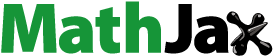
Abstract
Doxorubicin is widely used anticancer drug; however, use of doxorubicin is limited. Under externally applied magnetic field, magnetic agents can help to transport drug directly to tumor. Folate receptor is overexpressed in ovarian carcinomas. In this study, we aimed to develop magnetically responsive and folate receptor-targeted biomimetic drug delivery system for ovarian cancer therapy. Doxorubicin-loaded and glucose/gluconic acid-coated magnetic nanoparticles were synthesized and erythrocyte membrane vesicles were used for coating of nanoparticles. Folate ligand was anchored to surface so as to target receptor. Hydrodynamic size of nanocarrier was found as 91.2 ± 20.8 nm. The results showed that delivery system has controlled drug release profile and biocompatible features. In folate-free medium, folate receptor-targeted nanocarrier showed 10.33-fold lower IC50 values for A2780 cells and 3.93-fold lower for OVCAR3 cells compared to non-targeted nanoparticles and demonstrated more cytotoxicity against ovarian cancer cells. Moreover, magnetically and folate receptor-targeted doxorubicin delivery system was significantly more effective for therapy of xenografted nude mice than free doxorubicin based on tumor shrinkages and biochemical parameters. In conclusion, it can be suggested that folate ligand-attached and biomimetically designed magnetic drug delivery system have advantages and potential for targeted ovarian cancer therapy.
Graphical Abstract
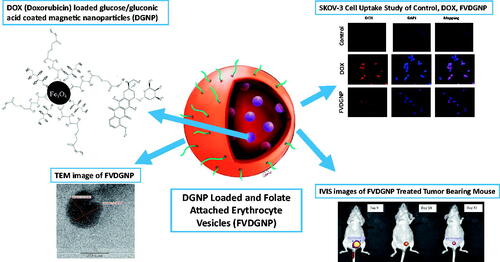
Introduction
Gynecological cancer especially ovarian cancer is major cause of mortality in women following breast cancer. Doxorubicin (DOX) is anti-cancer drug and also known as anthracycline antibiotic [Citation1]. Unfortunately, DOX has many undesirable side effects such as cardiotoxicity, hand–foot syndrome and myelosuppression leading to have very narrow therapeutic index of the medication [Citation2]. In addition to undesirable side effects, drug resistance is important issue. Nano-carrying systems are common in medicine and pharmaceutical technology and nanoparticles can be designed as biodegradable and biocompatible for drug delivery [Citation3]. Targeted drug delivery systems promise developed therapeutic activity by increasing concentration of drugs at target tissue [Citation4]. Magnetic targeting provides controlled drug release at target area with utilizing external magnetic field [Citation5,Citation6]. Receptor-targeted ligand carrying delivery systems can overcome drug resistance mechanism because they can be taken into cell by receptor-mediated endocytosis [Citation7]. Folate receptor (FR) has been observed in various types of cancers. In many studies, over 90% of ovarian carcinomas overexpress FR [Citation4] so FR could be used for targeted therapy. For this reason, in this work we developed magnetically responsive and FR-targeted DOX delivery system.
It is stated that when free drug is encapsulated in erythrocytes as carrier system, release of drug from cells is very rapid. This unwanted rapid release can be prevented by using particulate formulation and encapsulating into cells [Citation8]. In Hu et al. report [Citation9], nanoparticles have coated with erythrocyte membrane-derived vesicles and it is stated that vesicular structure has simulated erythrocyte membrane structure to large extent, has been expressed as biomimetic system and may provide advantage for personalized treatment.
Here, we developed biomimetic RBC vesicle-coated DOX carrying novel magnetic nanoparticular system for ovarian cancer treatment. Novelty of work is preparing of biomimetic, magnetic and glucose-coated pH-cleavable bond containing DOX nanocarriers. Folate ligand was anchored to RBC vesicle surface to target ovarian cancer cells via FR interaction. In first step, DOX-loaded glucose/gluconic acid-coated magnetic nanoparticles (GNP) were prepared and characterized. These magnetic nanoparticles provide targeting of system to preferred area. In the next step, ghost cells were prepared using RBCs and folate ligand anchored onto cell membrane vesicles, then drug-loaded magnetic nanoparticles were encapsulated into vesicles to gain biomimetic feature. In last steps, in vitro, in vivo and ex vivo studies were performed to evaluate treatment efficiency of nanocarrier. All results revealed that folate ligand-attached and biomimetically designed magnetic drug delivery system have potential for targeted ovarian cancer therapy. This system could allow personalized ovarian cancer therapy since, when erytrocytes supplied from ovarian cancer patient, these nanocarrier systems can be prepared from patient’s own erythrocytes.
Material and method
Synthesis of GNP and DOX conjugation
GNP were synthesized using modified reactions described by Lu et al. (2010 and 2013) [Citation10,Citation11]. About 0.05 M d-glucose (Fluka, USA) solution was added into 0.06 M FeCl3 (Sigma Aldrich) solution in jacked reaction vessel at 80 °C under N2 gas. After that, reaction temperature was decreased to 60 °C and NaOH solution was added until the pH was adjusted to 10 and left to react for 30 min. End of time, obtained nanoparticles were washed with water and ethanol by magnetic decantation and were dried overnight. Characterization studies were performed by Raman spectroscopy (Bruker Fra 160/S), X-ray photoelectron spectroscopy (XPS, SPECS EA 300), X-ray diffraction (XRD, Philips X’Pert Pro), scanning electron microscopy (SEM, Philips XL 30S FEG), vibrating sample magnetometer (VSM, LDJ Elextronics 9600), fourier-transform infrared spectrophotometer (FTIR, Perkin Elmer FTIR Spectrum One-B Spectrometer) and thermogravimetric analyzer (TGA, Perkin Elmer Diamond TA/TG).
For binding of DOX (Chemos) onto nanoparticles via hydrazone bond, nanoparticles were modified by using two steps, which are carboxymethylation described by Tijsen et al. 2001 and hydrazination mentioned by Lu et al. (2008) and Lassen et al. (2010) [Citation12–14]. For carboxymethylation of gluconic acid, 500 mg GNP were dispersed in water:isopropanol mixture 5:95 (v/v). About 10 M NaOH solution was added under N2 gas. Then, 12.75 mg monochloroacetic acid was added to mixture and allowed to react. After completion of reaction, carboxymethylated GNP (CGNP) were washed and nanoparticles were dried. For hydrazination of CGNP, 100 mg sample was dispersed in 10 ml water and 5 µmole hydrazine hydrate, 15 µmole EDC and 16.25 µmole NHS were added into this dispersion. Reaction was left for 4 h and later, hydrazinated GNP (HGNP) were washed. Characterization studies were performed by FTIR, TG and XPS analyses.
To attach DOX onto nanoparticles, 20 mg HGNP were dispersed in acetate buffer [Citation15] (10 mM, pH 6.5). Varying drug concentrations (0.5, 1, 1.5, 2, 3 and 4 mg/mL) were added into HGNP solution and incubated for 48 h and solution was neutralized. After that, DOX-loaded GNP (DGNP) were precipitated by centrifugation. Spectrophotometric determinations were carried out at 486 nm in supernatants. Drug loading amount and yield were calculated according to following EquationEquations (1)(1)
(1) and Equation(2)
(2)
(2) , respectively. All reactions were schematized in . Characterization studies were performed by FTIR, TG and XPS analyses. Moreover morphology of DGNP were investigated with transmission electron microscopy (TEM) (JEOL JEM 2100F, Tokyo, Japan).
(1)
(1)
(2)
(2)
Preparation of FR-targeted magnetic drug delivery system
Folate ligand was attached to ghost cells based on method mentioned by Fang et al. (2013) [Citation16] and DGNP-loaded erythrocyte membrane vesicles was developed using described by Gupta et al. (2014) [Citation17]. First of all, for ghost cell preparation, method mentioned by Hu et al. [Citation9] was used. Whole blood was collected in EDTA-containing tube and centrifuged. Plasma and buffy coat were separated from erythrocytes and cells were washed with 1× physiological buffered solution (PBS). Erythrocytes were incubated in ice-cold buffer with addition of 0.1× PBS for hemolysis. Resulting ghost cells were obtained and washed with PBS. Then, 75 μg/mL 1,2-distearoyl-sn-glycero-3-phosphoethanolamine (folate-PEG-DSPE, 2000 Da, Nanocs Inc., New York, USA) solution was incubated with 1 ml of ghost cell at 4 °C. End of incubation period, ghost cells were centrifuged and kept in sonic bath. Ghost cells were passed through mini-extruder (Avanti Polar Lipids Inc., USA) using 400 and 100 nm pore-sized membranes, respectively. Obtained vesicles were dispersed in 0.1× PBS and 500 μL of erythrocyte vesicles were incubated with 2.5 ml of DGNP at concentration of 0.2 mg/mL (in 1% sucrose solution) for 1 h and at 37 °C. Then, 25% sucrose solution was added to achieve isotonic medium and incubated. DGNP-loaded and folate-attached erythrocyte vesicles (FVDGNP) were purified with centrifugation and encapsulation yield was determined by inductively coupled plasma-mass spectrometry (ICP-MS) (Agilent 7500series, Santa Clara, USA) through Fe analysis. Folate non-attached nanoparticle-loaded vesicles (VDGNP) were also prepared as control group for analyses. FVDGNP was illustrated in . Morphology of FVDGNP was examined (after staining with 1% uranyl acetate solution) with TEM (Carl Zeiss Libra 120 kV, Oberkohen, Germany) and hydrodynamic size was studied with both zeta sizer (Malvern Zeta Sizer NanoSZ, Malvern Instruments Ltd., UK) and nanoparticle tracking analysis (NTA) system (Malvern Nanosight, Malvern Instruments Ltd., UK).
Serum protein binding, hemolysis and macrophage uptake
Fetal bovine serum (FBS, Biowest, USA): nanocarrier mixtures (4 mg Fe/mL) were prepared at 10:90, 20:80, 40:60 and 60:40 (v/v) ratios with 600 µL final volume. The mixtures were incubated for 2 h at 37 °C and centrifugated. Pellets were washed with Mcllvaine’s buffer (pH 7.5) [Citation18,Citation19]. Bradford assay [Citation20] was used for protein determination in supernatants, then protein-binding amount and yield were calculated.
To determine hemolysis potential of nanocarrier, erythrocytes (2% hematocrit level) were mixed with nanoparticles at 0.5, 1, 1.5 and 2 mg/ml GNP, DGNP (in 10% sucrose soluton) and 25, 20, 15, and 10 μg/mL nanoparticle-containing VDGNP and FVDGNP (in PBS solution) at 1:1 (v/v) ratio and incubated at 37 °C for 2 h. Sucrose solution and PBS were used as negative control groups and 1% Triton X-100 as positive control group. End of incubation, erythrocytes and nanocarriers were separated from mixture by centrifugation and hemoglobin was spectrophotometrically determined at upper layer at 540 nm. Hemolysis ratio calculated via absorbance with the formula (EquationEquation (3)(3)
(3) ) below [Citation21,Citation22].
(3)
(3)
RAW 264.7 cells (purchased from ATCC, Wesel, Germany) were cultured at 10% FBS, 1% penicillin–streptomycin, l-glutamine-containing DMEM and incubated at 37 °C under 5% CO2 environment. About 1 ml cells (2.04 × 104 cell/mL) were mixed with 100 µL of nanocarrier (25 µg nanoparticle/mL) (DGNP, FVDGNP, VDGNP) and incubated for 5 h. Afterwards, 25 µL of this mixture were taken and investigated for morphological changes with cell counter and imaging device (Arthur, NanoEntek Inc., USA).
Drug release
Release characteristics of DOX from DGNP was determined in 10 mM pH 7.4 phosphate and pH 5.5 acetate buffer. Nanoparticles were taken in dialysis membrane (molecular weight: 2000 Da) and placed at 37 °C. The dialysis medium was changed for certain periods and replaced with fresh buffer. Free DOX formulation was also used for release profile. The released drug amounts were calculated as follows (EquationEquation (4)(4)
(4) ) and release profiles were evaluated [Citation23,Citation24].
(4)
(4)
Cell culture studies
A2780 (bought from ECAC) and OVCAR3 cells (supplied from ATCC) were cultured at 10% FBS, 1% penicillin–streptomycin, 2 mM l-glutamine-containing RPMI-1640 medium and SKOV3 cells (purchased from ATCC) were cultured at 10% FBS, 1% penicillin–streptomycin, 2 mM l-glutamine-containing DMEM and incubated at 37 °C under 5% CO2 environment. Cytotoxicity analysis was performed with 3-4,5-dimethyl thiazol-2-yl-2,5-diphenyltetrazolium bromide (MTT, Amresco Inc., USA). For all cell lines, 10 × 103 cells per well were seeded in 96-well plate and incubated for 24 h. After cell attaching, 100 µL of drug group at 5, 10, 25, 100, 500, 1000, 1500, and 2000 ng DOX/mL concentrations were added onto cells and incubated for 24, 48 and 72 h. FVDGNP was also analyzed using folate-free RPMI-1640 to research folate-binding capacity. After incubation, media were discarded and medium: MTT solution (10:1) (v:v) mixture was added to each well. Cells were incubated for 4 h and formed formazan crystals were dissolved in dimethyl sulfoxide. Absorbance was recorded at microplate reader (Polarstar Omega, BMG Labtech, Germany) at 540 nm and IC50 values for 72 h were calculated using GraphPad Prism5 software (USA). The data statistically analyzed by analysis of variance (ANOVA) using SPSS software version 24.0 (USA) and statistical significance was set at p < .05.
To analyze uptake of drug delivery system by ovarian carcinoma cells, 100 µL of cells (at 5 × 104 cells/mL density) were inoculated on microscope slides placed in six-well plate and incubated overnight. Media were discarded and PBS, DOX and FVDGNP at 2 µg DOX/mL concentration were added. After 2 h, cells were washed with PBS and for fixation 200 μL of 2% formaldehyde solution was added. After washing, cells were treated with 4,6-diamino-2-phenylinediol (DAPI) for nuclear staining. Both DAPI fluorescence and DOX fluorescence were examined by fluorescent microscopy (Olympus BX51, Tokyo, Japan).
Biodistribution and cancer treatment potential
All animal experiments were approved by the Animal Ethics Committee of Ege University. For biodistribution studies, 4–6-weeks-old CD-1 female mice and for evaluation of cancer treatment experiments CD-1 female nude mice were used. During studies, mice were kept under controlled conditions and were fed with food and water ad libitum.
For biodistribution studies, 100 µL of drug group at 30 µg DOX/mL concentration was injected to mice from tail vein (n = 3) and magnet (0.1 Tesla) was fixed to abdominal region of each mice for application of nanocariers except from free DOX. After 60 and 180 min of post-injection, mice were sedated via inhalation of isofluorane and visualized fluorescently (λex 465 nm/λem 600 nm) in dorsal decubitus at in vivo imaging system (IVIS Spectrum, Perkin Elmer, Caliper Life Sciences, USA) by removing magnet. Then (180 min of post-injection), mice were sacrificed and blood was collected into lithium/heparin tubes; lung, liver, kidney, spleen, large intestine, ovary and muscle tissues were gathered. For ex vivo analyses, DOX in organs/tissues were visualized with IVIS and organs/tissues homogenized for HPLC analysis [Citation25]. Organs/tissues were washed with water and PBS. About 100 mg sample were homogenized in 0.1 M sodium dodecyl sulfate solution and Tris buffer (pH =8.8, 1 M). Then, methanol and chloroform were added and centrifuged. DOX-containing chloroform layer was collected and dried. Obtained pellet was dissolved in mobile phase: trifluoroacetic acid (0.05 M) and acetonitrile mixture (60/40, v/v). HPLC conditions were as follows: Shimadzu Prominence HPLC System, C18 Inertsil ODS 3–3 column (15 cm × 4.00 mm), 20 µL injection volume, 1 ml/min flow rate, 30 °C, fluorescent detector: λex 480 nm, λem 558 nm. Biodistribution (%) data were calculated based on the percentage of total amount of DOX in related tissue/organ over total amount of DOX in all tissue/organs.
For ovarian cancer treatment studies, xenograft tumor model was generated in nude mice with SKOV3 cells transfected with luciferase gene (supplied from Cell Biolabs Inc., USA). About 6 × 106 SKOV3-Luc cells were injected intraperitoneally. In order to monitor tumor formation and tumor volume dedection, either luciferin (Biovision Inc., USA; 12 mg/mL in PBS) was injected intraperitoneally following mice were visualized with IVIS 5 min later or 2DG-750 probe (XenoLight RediJect, Perkin Elmer, USA) (λex 745 nm/λem 820 nm) was administrated via tail vein following mice were visualized 3 h later. When tumor volume was reached to minimum 50 mm3, drug groups were injected via tail vein at 2–3 day interval (n = 3) [Citation26,Citation27]. FDVGNP and DOX were administrated at 200 µL of 0.5 mg DOX/kg dose and also PBS was injected to control groups. Tumor volume shrinkage of mice were observed by IVIS and at the end of treatment, cardiac blood was collected and biochemical analyses were performed with VetScan VS2 analyzer.
Results and discussion
Synthesis of GNP and DOX conjugation
GNP were synthesized both controlled partial reduction of Fe3+ using α-d-glucose as the reducing agent and by co-precipitation method in alkaline medium. According to Raman spectrum (), signals at 213, 274, 381, 488, 589 and 1270 cm−1 verified magnetite structure [Citation28]. XRD analysis confirmed magnetite phase and hkl indices as follows: 220 at 30.223°, 311 at 35.5432°, 400 at 43.203° and 440 at 62.658° as mentioned in a study [Citation29]. According to FTIR spectrum of GNP (), peaks at 3318, 2900, 1600 and 1350 cm−1 belong hydrogen bonded O–H streching, C–H stretching, C = O streching and C–H bending of glucose/gluconic acid chain, respectively. XPS spectrum was seen in and showed that GNP had C/Fe ratio as 2.2 and O/Fe ratio as 5.2. GNP hysteresis curve had paramagnetic properties under room temperature and 1.5 T magnetic field (). Based on thermogravimetric measurements (), GNP had 19% organic layer and 9% bound water of its weight and nanoparticles were decomposed at 325 °C except from magnetite structure which was stabile still at 600 °C [Citation30]. Size of GNP was found to be ∼55–70 nm and spherical-like-shaped nanoparticles were obtained (). Hydrodynamic size was determined as 112 nm since magnetic nanoparticles tend to attract each other due to magnetic properties and form aggregates [Citation31,Citation32].
Figure 2. Characterization of GNP: (A) Raman spectroscopy, (B) XPS, (C) VSM, (D) TG and (E) SEM analyses.
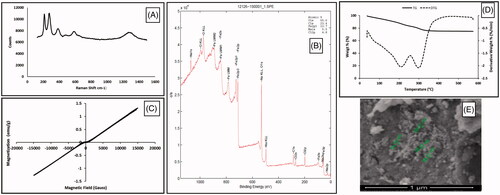
Hydrazone linkage is type of bond that is readily cleavable in acidic environment, and this bond was chosen for nanoparticle-DOX linkage for our study. Carboxymethylated and hydrazinated GNP structure were investigated with XPS, FTIR and TG analyses. XPS data confirmed newly added carboxymethyl group (). The C/Fe ratio was increased to 13.3 and O/Fe ratio increased to 16.1 according to those ratios of GNP. C content of nanoparticles increased six times and O content increased three times. FTIR spectra () showed that signals at 1085 cm−1 could also belong to C–O streching of newly added and free carboxylic acid group of CGNP. The peak at 1100 cm−1 could belong to C–N streching of aliphatic amine group of hydrazine structure in HGNP which was different from CGNP spectrum. Modifications were verified with these data. However, organic layer percentage was still same in CGNP and HGNP within GNP because of slightly differencies in weight (Supplementary Figure S1).
In , while loaded drug amount was rising, loaded drug efficiency decreased with increasing initial DOX concentrations. When 3 and 4 mg/mL initial drug concentrations were used, loaded drug amounts were determined as 280 and 285 µg per mg nanoparticle respectively; however, drug loading yields were found as 47.2 and 37.9% respectively. For this reason, optimum initial DOX concentration was chosen as 3 mg/mL. According to FTIR spectra of DGNP () and DOX (Supplementary Figure S2), signal at 3785 cm−1 belongs to N–H streching and also signals at 1280 and 1210 cm−1 belong to C–N streching which were found both DOX and DGNP structure. Moreover, peak at 1680 cm−1 refer to carbonyl group and can be also H-C = N streching [Citation33]. In addition peak at 1400 cm−1 may be regarded to C = C streching in aromatic ring. These results verified the presence of DOX in DGNP. Moreover, DGNP maintained the paramagnetic properties (). According to TG analysis (), there was no considerable decomposition until 210 °C since first decomposition step of DOX was at 235 °C and it was calculated that 24% of DGNP decomposed at 600 °C. Morphology of DGNP was determined as rod-like shape seen in and hydrodynamic size was measured as 140 nm (bigger than GNP).
Preparation of folate receptor-targeted magnetic drug delivery system
Folate-PEG-DSPE was anchored on vesicles to target ovarian cancer cells. Besides magnetic targeting, addressing of nanocarrier with FR interaction could bring about dual targeted and efficient cancer treatment. It was stated in literature that pores may be formed on cell surface at size of 10–500 nm by controlling osmolarity [Citation17]. In this work, we loaded DGNP into vesicles by opening pores with hypotonic solution leading nanoparticle’s entry following closing of pores with hypertonic solution resulting isotonic medium and nanoparticle entrapment. Mixture ratio of ghost cells and nanoparticles were used in accordance with the previous work [Citation9] to fully coat of nanoparticles. DGNP loading yield was determined as 98% of total weight. The amount of anchored Folate-PEG-DSPE was 38.70 μg per mL ghost cell. It was dedected by UV spectroscopy (at 284 nm). Obtained FVDGNP was seen as 90–130 nm with nearly spherical shape (). While hydrodynamic size of empty vesicles were 120 ± 24.58 nm, FVDGNP had size of 165 ± 25.39 nm showed larger structure. Hydrodynamic size of FVDGNP was also studied with NTA system and it was found that size was in the range of 82–168 nm (Supplementary Figure S3). It can be said that when nanoparticle load into vesicles, the size increases.
Serum protein binding, hemolysis and macrophage uptake
At 60% serum, 40% DGNP and FVDGNP (maximum serum protein-binding ratio) showed approximate protein binding of 11.03 and 45.32%, respectively (). FVDGNP was found to be significantly different when compared to DGNP since it was thought that vesicular structure led to more protein binding. It is quite high when compared with other study [Citation19]. Moreover serum protein binding affinity of GNP and DGNP (Supplementary Table S1) were slightly different from each other.
Table 1. Serum protein-binding yield of nanocarriers with varying ratios of serum:nanocarrier suspension.
As known [Citation34], increase in nanoparticle concentration may cause erythrocyte hemolysis. Moreover, variability in surface charge of nanoparticle could result in hemolysis (anionic nanoparticles leads to less hemolysis than cationic nanoparticles). Based on results, FVDGNP did not bring about any hemolysis since FVDGNP have anionic surface charge (−14.1 ± 0.6 mV). Furthermore, DGNP also did not lead to hemolysis even at concentration of 25 µg/mL.
Physicochemical properties influence nanoparticle uptake and clearance by macrophages. Generally, macrophage uptake of larger particles are more efficiently than smaller particles of same properties [Citation34]. About 1% macrophage uptake (Supplementary Figure S4) of FVDGNP and DGNP was dedected even at 5 h, thus it can be clearly said that our nanocarrier could not be recognized by RES.
Drug release
Drug release profile of DOX and FVDGNP were examined at both acidic pH value and physiological pH (). Whereas more than 50% of free DOX was released in both pH media in first 3 h, carrier-bound DOX was released at pH 5.5 and 7.4 at 0.28 and 0.11%, respectively. The amounts of drug released in 1 and 3 h were 2.64 and 0.61 μg DOX/mg NP at pH 5.5 and 7.4 and 8.2 and 3.81 μg DOX/mg NP in 24 h, respectively. Drug release data confirmed acid-labile hydrazone bond of drug. Also in other works [Citation15,Citation35] release of hydrazone-bound DOX in acidic medium appears to be higher than in physiological pH. According to results, side effects of drug could be minimized by using nanocarrier system due to lower drug release; however, more DOX release can be seen in target region.
Cell culture studies
A2780, OVCAR3 and SKOV3 cells were chosen for cytotoxicity studies and GNP were not found as cytotoxic. shows each cell death caused from drug group treatments for 24, 48 and 72 h. Graphics demonstrated that cell survival was decreased with increasing time suggested that drug carriers allowed controlled drug release. IC50 values belonged to FVDGNP and other groups were seen from . IC50 values of VDGNP and FVDGNP are lower than (p < .05) DGNP. For SKOV3 cell line, IC50 values of VDGNP and FVDGNP are 1.25- and 2.45-fold, for A2780 cell line 1.25- and 1.60-fold and for OVCAR3 cell line 1.76- and 2.31-fold lower in comparison with DGNP, respectively. FVDGNP in folate-free medium containing cell lines were found as more effective (p < .05) than free DOX. This case might be explained by drug sensitivity of cells as well as uptake of drug carrier into cell. While folate-free nanoparticles are uptaken into cancer cells through non-specific binding/penetration process, folate carrying nanostructures enters cells via receptor-mediated endocytosis following interaction of folate ligand with FR [Citation36]. In study reported in 2012, it was found that liposome coated magnetic nano-crystal and DOX-containing nanospheres entered more effectively into cells in comparison with non-coated magnetic nanospheres [Citation37]. In addition in many studies, it was showed that folate bearing nanocarriers were highly uptaken into FR expressing cells and demonstrated more activity according to control groups [Citation38–41]. In our work, with OVCAR3 and A2780 cells using folate-free medium, FVDGNP showed 10.33-fold lower IC50 values for A2780 cells and 3.93-fold lower for OVCAR3 cells in comparison with DGNP. It was known that FR expression level is lower in OVCAR3 cells and higher in SKOV3 and A2780 cells and this situation could explain the difference of IC50 values between cell lines [Citation42,Citation43]. Moreover IC50 values of FVDGNP were decreased (p < .05) with using folate-free medium showed interaction of folate ligand with its receptor.
Figure 6. Death (%) of cells treated with drug groups: for SKOV3 cells at (A) 24, (B) 48, (C) 72 h, for A2780 cells at (D) 24, (E) 48, (F) 72 h and for OVCAR3 cells at (G) 24, (H) 48, (I) 72 h.
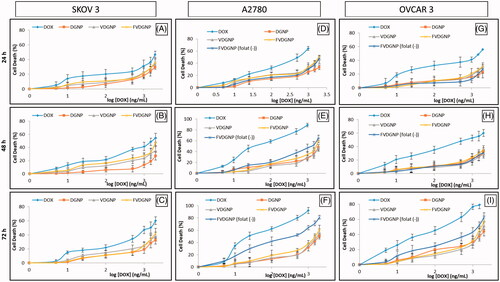
Table 2. IC50 values (µg/mL) of DOX and DOX-loaded nano vehicles for SKOV3, A2780 and OVCAR3 cell lines at 72 h.
As seen in images of cells visualized by fluorescent microscope, it can be suggested that both DOX and FVDGNP gave red signals in nuclei region (). However, DOX signal for FVDGNP was lower than free DOX which is in consistent with cytotoxicity studies. Similar results were obtained by Li et al. in 2013 [Citation43]. The reason of this may be due to slower entrance of FVDGNP into cell via receptor-mediated endocytosis mechanism compared to faster diffusion of free DOX.
Biodistribution and cancer treatment potential
To evaluate extent to which drug form can deliver DOX into target region whereas miniziming its accumulation in other organs, biodistribution of DOX in whole body was examined both IVIS Spectrum and ex vivo analyses. There was no significant difference between images obtained at 1 and 3 h, therefore in vivo images taken at 3 h () and ex vivo images were demonstrated in . It was seen that under magnetic field signal of drug in abdominal region using magnetic forms was higher than that of free DOX owing to magnetic response of nanocarriers. Drug distributed to all organs using free form. These results were obtained through HPLC assay ().
Figure 8. IVIS Spectrum images of biodistribution of (A1) DOX, (A2) DGNP, (A3) VDGNP and (A4) FVDGNP in mice at 3 h. Representative ex vivo images of liver, lung, spleen, kidney, ovary, large intestine and muscle (top to down) 3 h after injection of (B1) DOX, (B2) DGNP, (B3) VDGNP and (B4) FVDGNP.
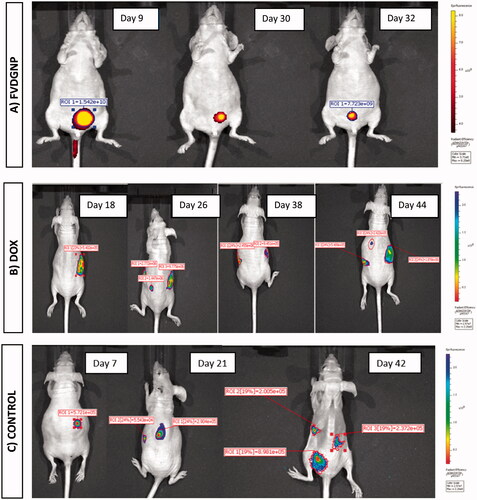
Thanks to biomimetic property of vesicular forms, distribution of VDGNP and FVDGNP in liver and lung were reduced. Similarly, in report, whereas free DOX mostly accumulated in liver, lung and spleen, DOX carrying magnetic nanoparticles accumulated relatively lower in liver and lung [Citation44]. Distribution of FVDGNP in ovary was further increased in comparison with VDGNP as a result of FR interaction hence, it could be said that drug will be highly accumulated in ovary region by using FVDGNP as nanocarrier. Likewise, in a study described by Wang, folate ligand bearing nanoparticles allowed accumulation of greater amount of drug in tumor containing FR positive cells with reduced access to non-tumor tissues [Citation45].
After biodistribution studies, we further examined antitumor efficiency of FVDGNP and compared with DOX in SKOV3-luc cell-xenografted nude mouse model. Tumor inhibition/growth profile of each group including untreated group were monitored and displayed in and tumor volumes and survival of animals can be seen from . FVDGNP treatment group, one of initial tumor volume was dedected as 853.4 mm3 in ninth day and tumor growth inhibition after inoculation (7 dose injection) was found as 93% (). Other initial tumor volumes were determined as 113.5/392.6 mm3 and 45.6 mm3. These tumors were completely treated after 16–18 days (3 dose injection) of inoculation, this may be caused from initial smaller volume of tumor. However, DOX treatment group, two of mice had metastasis despite 3 dose injection of DOX and those mice died after 6–7 times of administration. Another mouse had also metastasis in spite of three times of DOX injection and only slight treatment was monitored. Furthermore one of tumor volume of that animal reached to 1078.3 mm3 at 44 days post-inoculation (). According to results, FVDGNP showed much better antitumor activity than DOX. Any treatment was observed in PBS administrated group and mice were lost weight (). Similarly, in another work, injection FR-targeted DOX-loaded micelles suppressed 86% of tumor growth inhibition of KB-cell xenografted nude mice compared to control group suggesting better targeting ability to tumors [Citation46]. Briefly, compared to DOX group, FVDGNP group showed significant tumor treatment due to either magnetic or/and FR targeting.
Figure 9. IVIS Spectrum tumor images of mice intravenously treated with (A) FVDGNP (visualized with 2DG-750 probe), (B) DOX and (C) PBS (both visualized with luciferin solution).
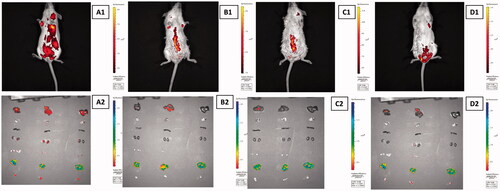
Table 3. Data indicates the survival and imaging period of nude mice in all treatment groups.
Safety of FVDGNP was also investigated with biochemical analyses by comparing the effects of PBS and free drug (). Healthy mice parameters were used for reference values. As known, decreasing of albumin (ALB) and increasing of alanine aminotransferase, aspartate aminotransferase and globulin levels mean liver damage and inorganic phosphorus (PHOS), creatinine (CRE) and ion levels elevations indicate kidney injury. When PBS- and DOX-treated group were compared with reference biochemistry results, remarkable liver damage can be seen from those analyses. However, compared to DOX group, in FVDGNP group ALB level was increased and AST and GLOB levels were decreased remarkably. In addition PHOS, CRE and ion levels of FVDGNP receiving mice were stayed more closely to reference values contrary to that of DOX-administrated mice. Hence, it can be revealed that changes in liver and kidney function of FVDGNP-treated group was lower than DOX-treated mice. Moreover, cardiotoxicity is determined especially with increase in creatine kinase (CK) enzyme levels. There was significant raise in CK value of DOX-receiving mice according to reference level (278 U/L) and increased to 792 U/L. In another work [Citation47], it was stated that CK levels in DOX-administrated rat group were considerably increased as a result of myofibrillar degeneration in heart. However, CK level was found as 383 U/L in FVDGNP-treated mice and showed that FVDGNP leads lower myocardial damage. In Meng et al.’s study [Citation48], similar biochemical data were described with using DOX carrying nanoparticle system against free form of DOX when investigated in terms of kidney and liver functions.
Table 4. Blood biochemistry profiles of healthy and PBS, DOX and FVDGNP-treated mice groups.
Conclusions
In this study, GNP were synthesized and DOX covalently attached to nanoparticle via hydrazone bond. Folate-PEG-DSPE was attached to erythrocyte ghost cells and extruded, afterwards, DGNP were encapsulated into folate-anchored cell membrane vesicles. According to characterization and in vitro studies, FVDGNP had biomimetic property, controlled drug release ability, cytotoxicity and FR interaction on ovarian cancer cells. In addition, nanocarrier could be targeted magnetically and also could be accumulated in ovary. Compared to DOX, FVDGNP showed significant tumor treatment and decreased side effects due to either magnetic or/and FR targeting. From all these results, FVDGNP has great potential for ovarian cancer treatment.
SUPPLEMENTARY.docx
Download MS Word (232.4 KB)Acknowledgements
The authors would like to acknowledge to Ege University Drug Development and Pharmacokinetics Research-Application Center (ARGEFAR) for the laboratorial support for this research.
Disclosure statement
No potential conflict of interest was reported by the authors.
Additional information
Funding
References
- Sheng Q, Liu J. The therapeutic potential of targeting the EGFR family in epithelial ovarian cancer. Br J Cancer. 2011;104:1241–1245.
- Jung K, Rezska R. Mitochondria as subcellular targets for clinically useful anthracyclines. Adv Drug Deliv Rev. 2001;49:87–105.
- Chomoucka J, Drbohlavova J, Huskab D, et al. Magnetic nanoparticles and targeted drug delivering. Pharmacol Res. 2010;62:144–149.
- Sudimack J, Lee RJ. Targeted drug delivery via the folate receptor. Adv Drug Deliv Rev. 2000;41:147–162.
- McBain SC, Yiliu HHP, Dobson J. Magnetic nonoparticles for gene and drug delivery. Int J Nanomed. 2008;3:169–180.
- Wilczewska A, Niemirowicz K, Markiewicz KH, et al. Nanoparticles as drug delivery systems. Pharmacol Rep. 2012;64:1020–1037.
- Cho K, Wang X, Nie S, et al. Therapeutic nanoparticles for drug delivery in cancer. Clin Cancer Res. 2008;14:1310–1316.
- Hamidi M, Rafiei P, Azadi A, et al. Encapsulation of valproate-loaded hydrogel nanoparticles in intact human erythrocytes: a novel nano-cell composite for drug delivery. J Pharm Sci. 2011;100:1702–1711.
- Hu CMJ, Zhang L, Aryal S, et al. Erythrocyte membrane-camouflaged polymeric nanoparticles as a biomimetic delivery platform. PNAS. 2011;108:10980–10985.
- Lu W, Shen Y, Xie A, et al. Green synthesis and characterization of superparamagnetic Fe3O4 nanoparticles. J Magnet Magnet Mater. 2010;322:1828–1833.
- Lu W, Shen Y, Xie A, et al. Preparation and drug-loading properties of Fe3O4/poly(styrene-co-acrylic acid) magnetic polymer nanocomposites. J Magnet Magnet Mater. 2013;345:142–146.
- Tijsen CJ, Kolk HJ, Stamhuis EJ, et al. An experimental study on the carboxymethylation of granularpotatostarch in non-aqueous media. Carbohydr Polym. 2001;45:219–226.
- Lu DX, Wen XT, Liang J, et al. Novel pH-sensitive drug delivery system based on natural polysaccharide for doxorubicin release. Chin J Polym Sci. 2008;26:369–374.
- Joullié MM, Lassen KM. 2010. Evolution of amide bond formation. USA: Arkat, Inc.
- Pourjavadi A, Hosseini S, Alizadeh M, et al. Magnetic pH-responsive nanocarrier with long spacer length and high colloidal stability for controlled delivery of doxorubicin. Colloids Surf B Biointerfaces. 2014;116:49–54.
- Fang RH, Hu CMJ, Chen KNH, et al. Lipid-insertion enables targeting functionalization of erythrocyte membrane-cloaked nanoparticles. Nanoscale. 2013;5:8884–8888.
- Gupta N, Patel B, Ahsan F. Nano-engineered erythrocyte ghosts as inhalational carriers for delivery of fasudil: preparation and characterization. Pharm Res. 2014;31:1553–1565.
- Cole AJ, David AE, Wang J, et al. Polyethyleneglycol modified, cross-linked starch coated iron oxide nanoparticles for enhanced magnetic tumor targeting. Biomaterials. 2011;32:2183–2193.
- Semete B, Booysen L, Kalombo L, et al. Effects of protein binding on the biodistribution of PEGylated PLGA nanoparticles post oral administration. Int J Pharm. 2012;424:115–120.
- Bradford MM. A rapid and sensitive method for the quantitation of microgram quantities of protein utilizing the principle of protein-dye binding. Anal Biochem. 1976;72:248–254.
- Mayer A, Vadon M, Rinner B, et al. The role of nanoparticle size in hemocompatibility. Toxicology. 2009;258:139–147.
- Yallapu MM, Chauhan N, Othman SF, et al. Implications of protein corona on physico-chemical and biological properties of magnetic nanoparticles. Biomaterials. 2015;46:1–12.
- Ak G, Yılmaz H, Hamarat SS. Preparation of magnetically responsive albumin nanospheres and in vitro drug release studies. Artif Cells Nanomed Biotechnol. 2014;42:18–26.
- Ak G, Sanlier SH. Synthesis of folate receptor targeted and doxorubicin coupled chemotherapeutic nanoconjugate and research into its medical applications. Prep Biochem Biotech. 2012;42:551–563.
- Alhareth K, Vauthier C, Gueutin C, et al. HPLC quantification of doxorubicin in plasma and tissues of rats treated with doxorubicin loaded poly(alkylcyanoacrylate) nanoparticles. J Chromatogr B. 2012;887–888:128–132.
- Kim JO, Kabanov AV, Tatiana K. Polymer micelles with cross-linked polyanion core for delivery of a cationic drug doxorubicin. J Control Release. 2009;138:197–204.
- Wang Z, He Q, Zhao W, et al. Tumor-homing, pH- and ultrasound-responsive polypeptide-doxorubicin nanoconjugates overcome doxorubicin resistance in cancer therapy. J Control Rel. 2017;264:66–75.
- Silva SW, Melo TFO, Soler MAG, et al. Stability of citrate-coated magnetite and cobalt–ferrite nanoparticles under laser irradiation: a raman spectroscopy investigation. IEEE Trans Magnet. 2003;39:2645–2647.
- Jiang JS, Gan ZF, Yang Y. A novel magnetic fluid based on starch-coated magnetite nanoparticles functionalized with homing peptide. J Nanopart Res. 2009;11:1321–1330.
- Zhang L, He R, Gu H. Oleic acid coating on the monodisperse magnetite nanoparticles. Appl Surf Sci. 2005;253:2611–2617.
- Easo SL, Mohanan PV. Dextran stabilized iron oxide nanoparticles: synthesis, characterization and in vitro studies. Carbohydr Polym. 2013;92:726–732.
- Nicolas J, Mura S, Brambilla D. Design, functionalization strategies and biomedical applications of targeted biodegradable/biocompatible polymer-based nanocarriers for drug delivery. Chem Soc Rev. 2013;42:1147–1235.
- Zhang K, Chen Z, Wei J, et al. A study on one-step laser nanopatterning onto copper–hydrazone-complex thin films and its mechanism. Phys Chem Chem Phys. 2017;19:13272–13280.
- Dobrovolskaia MA, Aggarwal P, Hall JB, et al. Preclinical studies to understand nanoparticle ınteraction with the immune system and its potential effects on nanoparticle biodistribution. Mol Pharm. 2008;5:487–495.
- Kievit FM, Wang FY, Fang C, et al. Doxorubicin loaded iron oxide nanoparticles overcome multidrug resistance in cancer in vitro. J Control Release. 2011;152:76–83.
- Domcke S, Sinha R, Levine DA, et al. Evaluating cell lines as tumour models by comparison of genomic profiles. Nat Comms. 2013;4:1–10.
- Wang H, Wang S, Liao S, et al. Folate-targeting magnetic core–shell nanocarriers for selective drug release and imaging. Int J Pharm. 2012;430:342–349.
- Wang L, Neoh KG, Kang ET, et al. Multifunctional polyglycerol-grafted Fe3O4@SiO2 nanoparticles for targeting ovarian cancer cells. Biomaterials. 2011;32:2166–2173.
- Nukolova VN, Obero SH, Cohe SM, et al. Folate decorated nanogels for targeted therapy of ovarian cancer. Biomaterials. 2011;32:5417–5426.
- Yang T, Li B, Qi S, et al. Co-delivery of doxorubicin and mi siRNA by folate receptor targeted liposomes exhibits enhanced anti-tumor effects in vitro and in vivo. Theranostics. 2014;4:1096–1111.
- Chiani M, Norouzian D, Shokrgozar MA, et al. Folic acid conjugated nanoliposomes as promisingcarriers for targeted delivery of bleomycin. Artif Cells Nanomed Biotechnol. 2017 [Jun 23]; [7 p.]. DOI:https://doi.org/10.1080/21691401.2017.1337029
- Corona G, Giannini F, Fabris M, et al. Role of folate receptor and reduced folate carrier in the transport of 5-methyltetrahydrofolic acid in human ovarian carcinoma cells. Int J Cancer. 1998;75:125–133.
- Li F, Zhang H, Gu C, et al. Self-assembled nanoparticles from folate-decorated maleilated pullulan–doxorubicin conjugate for improved drug delivery to cancer cells. Polym Int. 2013;62:165–171.
- Elbialy NS, Fathy MM, Khalil W. Doxorubicin loaded magnetic gold nanoparticles for in vivo targeted drug delivery. Int J Pharm. 2015;490:190–199.
- Wang F, Wang Y, Ma Q, et al. Development and characterization of folic acid-conjugated chitosan nanoparticles for targeted and controlled delivery of gemcitabinein lung cancer therapeutics. Artif Cells Nanomed Biotechnol. 2016;45:1530–1538.
- Zhang L, Lu J, Jin Y, et al. Folate-conjugated beta-cyclodextrin-based polymeric micelles with enhanced doxorubicin antitumor efficacy. Colloids Surf B Biointerfaces. 2014;122:260–269.
- Meng H, Xue M, Xia T, et al. Use of size and a copolymer design feature to improve the biodistribution and the enhanced permeability and retention effect of doxorubicin-loaded mesoporous silica nanoparticles in a murine xenograft tumor model. ACS Nano. 2011;5:4131–4144.
- Sahu BD, Kumar JM, Kuncha M, et al. Baicalein alleviates doxorubicin induced cardiotoxicity via suppression of myocardial oxidative stress and apoptosis in mice. Life Science. 2016;144:8–18.