Abstract
Polymerized porcine hemoglobin (pPolyHb), a kind of glutaraldehyde-polymerized haemoglobin-based oxygen carrier, was developed as a potential red blood substitute for clinical applications. Assessment of its absorption, distribution, and metabolism is a major determinant of its safety and efficacy. In this study, a series of pharmacokinetic parameters of pPolyHb were measured and calculated in different animal models, such as the top-load model, the 50% exchange transfusion model and the haemorrhagic shock model. The results showed that the kinetic process of pPolyHb in rats conforms to the laws of linear pharmacokinetics in vivo. The half-life of pPolyHb was superior and more stable under non-pathological conditions, but in clinical situations such as trauma and anaemia, the half-life of pPolyHb may decline. The study of clearance (CL) and the apparent volume of distribution (Vd) of pPolyHb in these three different animal models demonstrated longer residence and a predominant, targeted role of pPolyHb with little accumulation in tissues. Through this study, the superior pharmacokinetic characteristics of pPolyHb have been proved and will aid in the determination of a reasonable dosing regimen and administration interval in clinical situations.
Introduction
A current survey on the treatment of clinical blood diseases showed that allogeneic red blood cell (RBC) transfusion has long been considered an important treatment option for patients suffering from blood loss [Citation1]. However, due to the limited availability of blood, 80% of the world population does not have access to fresh blood products, especially in remote areas and combat zones [Citation2]. Moreover, even though there is enough blood available, there are numerous possible complications related to transfusion. It is noteworthy that donated blood may contain infectious agents such as malaria, HIV, hepatitis and West Nile virus [Citation3]. Further, the emergence of new infectious agents may lead to the spread of disease, increasing the cost of a unit of blood. Moreover, transfusion-related adverse events, both short term and long term, are among the costliest contributors to health care expenditures. In addition, there are new concerns regarding the safety of blood transfusions following extended duration of storage [Citation4,Citation5]. Meanwhile, blood group typing is time consuming and may cause the delay of transfusion. For these reasons, it has been a long-term goal of scientists and engineers to develop an efficacious and safe universal red blood cell (RBC) substitute for use in transfusion medicine [Citation6].
Polymerized porcine haemoglobin (pPolyHb) is a type of RBC substitute, with porcine haemoglobin (Hb) as the precursor material for synthesis, and glutaraldehyde as the crosslinking agent [Citation7]. Porcine Hb shares high homology with human Hb, and is free from contamination with human viruses and prions. Our previous isovolumic exchange transfusion experiments in rats demonstrated that pPolyHb helped maintain hemodynamic stability, enabled a suitable level of oxygen delivery to tissues, and effectively reversed anaerobic metabolism caused by extensive blood volume exchange [Citation8]. However, the pharmacokinetic characteristics of pPolyHb have not been thoroughly investigated.
Pharmacokinetics is the study of the absorption, distribution, metabolism and excretion of drugs or other exogenous substances, and the use of mathematical methods and kinetic principles to clarify the law of dynamic changes [Citation9]. The pharmacokinetic properties of a drug affect every stage of the overall drug development process, from the confirmation of drug targets to phase-III clinical trials. Pharmacokinetics are used for dose determination, dosage form and evaluation of the route of administration [Citation10]. In vivo evaluation of active metabolites and the rational use of drugs provide a basis to ensure the safety and effectiveness of drugs. In this study, we primarily measured and calculated pharmacokinetic parameters, such as maximal plasma concentration (Cmax), area under the plasma concentration time curve (AUC), plasma clearance (CL), apparent volume of distribution (Vd) and half-life (T1/2), which reflect the absorption, distribution and metabolism of pPolyHb. It is well known that clinical conditions can influence the pharmacokinetics of numerous drugs [Citation11]. Consequently, it is possible that the pharmacokinetics of Hemoglobin-based oxygen carriers (HBOCs) could be also altered in situations such as massive hemorrhage caused by injury, accidental blood loss, or a major surgery [Citation3]. To clarify this, we investigated the changes in pPolyHb pharmacokinetics using a 50% exchange transfusion model, a haemorrhagic shock model, except for a top-load model.
Materials
Animals
A total of 27 male and nine female Sprague-Dawley rats weighing 240 ± 20 g were obtained from the Xi’an Jiaotong University, China. Animals were kept under standardized temperature conditions (22 ± 1 °C) with free access to food and water. All rats were randomly divided into three models: Top-load (n = 18), 50% exchange transfusion (n = 6), and haemorrhagic shock (n = 6). In the Top-load model, 18 rats were randomly divided into three groups which included low dose (2.6 g/kg), medium dose (3.6 g/kg) and high dose (5.52 g/kg). The experiments described in this study were performed in adherence to National Institutes of Health guidelines on the use of experimental animals. The experimental protocol was approved by the Animal Care Committee of Northwest University, Xi’an, China.
Test solutions
pPolyHb (10.5 ± 0.5 g/dL, methaemoglobin 5%, endotoxin 1.0 EU/mL, osmolality 300–330 mOsm, PH 7.4 ± 0.05, average molecular weight of pPolyHb 600 ± 50 kD, 64 kD tetramer 2%) was formulated in a buffer consisting of Na+ 135–155 mmol/L, K+ 3.0–5.0 mmol/L, Ca2+ 1–3 mmol/L, Cl- 140–160 mmol/L and stored at 4 °C until use.
Methods
Surgical preparation
Rats were food-restricted for 12 h. On the day of surgery, rats were anesthetized via intraperitoneal (i.p.) injection with sodium pentobarbital (45 mg/kg, intramuscularly). For rats in the top-load model group, different doses of pPolyHb were injected at a rate of 7 ml/kg/h via the caudal vein. For the 50% exchange transfusion and haemorrhagic shock models, the left femoral artery was cannulated (PE 50 tubing) and connected to an MP150 Data Acquisition System (BIOPAC, Goleta, CA) to record blood pressure, ECG and heart rate. The right femoral artery and the caudal vein were cannulated to induce controlled haemorrhage, and the transfusion of pPolyHb, respectively.
Exchange transfusion protocol
Blood was withdrawn through the right femoral artery, and an equal amount of pPolyHb was simultaneously transfused through the caudal vein. In this model, rats received a 50% blood volume exchange (ET) transfusion with pPolyHb at a rate of 0.5 ml/min. Blood volume (BV) was equal to 7% of body weight (BW). After a 60-min period of observation, all catheters were removed. The surviving rats were sacrificed by barbiturate overdose after the final blood collection.
Haemorrhage and resuscitation protocols
After measuring the base concentration of pPolyHb, rats were rapidly haemorrhaged by withdrawal of 30 ± 5.0% of the blood volume (BV) via the right femoral artery catheter until the mean arterial pressure (MAP) decreased to 35 ± 5 mmHg (within 10 min). After 10 min of stabilization, rats were intermittently haemorrhaged by 30% removal of their blood volume and maintained hypotensive with a MAP of 35 ± 5 mmHg throughout the hemorrhagic shock period [Citation6]. At the end of the 90-min shock period, rats were perfused with 2 g/kg pPolyHb plus twice the lost blood volume of normal saline (NS) at a rate of 0.5 ml/min through the caudal vein over 30 min. After a period of observation of 60 min, all catheters were removed. The surviving rats were sacrificed by barbiturate overdose after the final blood collection.
Pharmacokinetics
Pharmacokinetic (PK) parameters were calculated using the plasma concentration time curve of pPolyHb [Citation12]. In this experiment, blood samples were collected at different time intervals, and the pPolyHb concentration of the blood sample was measured with a blood cell analyser. In the top-load model group, blood samples (0.5 ml) were obtained from the eyes at 0, 1, 2, 4, 8, 12, 18, 24, 30, 48, 72 h after transfusion. In the 50% exchange transfusion and hemorrhagic shock model group, blood samples (0.5 ml) were obtained before exchange transfusion or resuscitation, and 0, 1, 2, 4, 8, 12, 18, 24, 48, 72 h after pPolyHb transfusion. The sample collections were centrifuged immediately at 3000 rpm for 15 min, then the supernatant was obtained for measurement of pPolyHb concentration by blood cell analyser (XFA6100).
The pPolyHb plasma concentration time curves were drawn according to the measured concentrations. Non-compartmental methods employed by the DASver2.0 were used to calculate pharmacokinetic parameters. Maximal plasma concentration (Cmax) represents the greatest plasma concentration reached after dosing in each rat. The area under the plasma concentration time curve (AUC0-∞) was estimated using the linear trapezoidal rule to the last measurable concentration (AUC0-t). Extrapolation to infinity (AUCt-∞) was accomplished by dividing Ct by the negative value of the terminal slope (k) of the log-linear plasma concentration–time curve. Thus, AUC0-∞ is equal to the sum of AUC0-t and AUCt-∞. Additional parameters were calculated as follows: the plasma clearance (CL) was calculated as the dose divided by AUC0-∞, the mean residence time (MRT) was calculated as k−1, the apparent volume of distribution (Vd) was calculated as the product of CL and MRT, and half-life (T1/2) was calculated as ln (2) divided by k [Citation13].
Statistical analysis
All values in the text and figures are presented as mean ± SD. The differences between the three rat models or different doses of the top-load model were assessed by one-way ANOVA followed by unpaired Student’s t-test. Statistical significance was defined as p < .05 to reject a null hypothesis. Data calculation was done using GraphPad Prism 7.0 software, and this software was also used for mapping. The statistical moment method in the DAS 2.0 pharmacokinetic software was used to calculate the major pharmacokinetic parameters as following: Maximum concentration (Cmax), time at maximum concentration (Tmax), area under the curve (AUC), mean residence time (MRT) and elimination half-life (T1/2).
Results
Top-load model
As shown in , pPolyHb concentration reached its peak briefly, and then gradually declined until 48 h after transfusion, when the pPolyHb concentration could not be measured. illustrates that the increase in Cmax was directly proportional to the increase in dose (R2 = 0.972). The value of Cmax for each dose was 51.3 ± 1.58 g/L (low dose), 63 ± 2.96 g/L (medium dose), and 75.25 ± 3.02 g/L (high dose).
Figure 1. Plasma concentration–time curve in the top-load, 50% exchange transfusion and haemorrhagic shock models. (A) plasma concentration–time curve in the top-load model; (B) plasma concentration–time curve in the 50% exchange transfusion model; (C) plasma concentration–time curve in the haemorrhage shock model. Blood samples were drawn from the eyes at 0, 1, 2, 4, 8, 12, 18, 24, 30, 48, 72 h after pPolyHb transfusion. The blood samples were centrifuged, and the supernatant was obtained for measurement of pPolyHb concentration by blood cell analyser (XFA6100).
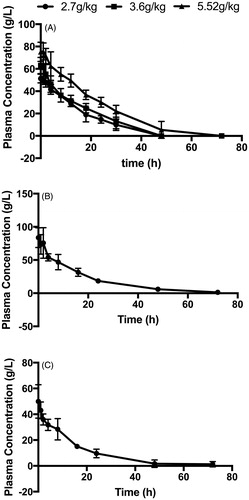
Figure 2. Maximal plasma concentration (Cmax) in the top-load, 50% exchange transfusion, and haemorrhagic shock models. The value of Cmax was the pPolyHb concentration after transfusion of pPolyHb or resuscitation with pPolyHb, since pPolyHb was administered intravenously. (A) Cmax of different doses in the top-load model. Cmax was directly proportional to increases in dose (R2 = 0.976); (B) Cmax in three different models. *p < .05 in comparison to the 3.6 g/kg group in the top-load model, &&&p < .0005 in comparison to 2.7 g/kg group in the top-load model. ##p < .005 in comparison to 5.52 g/kg group in the top-load model, $$p < .005 in comparison to the 50% exchange transfusion model.
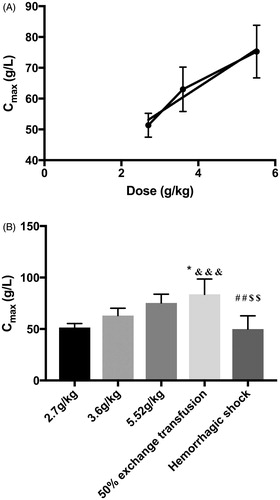
shows the area under the curve (AUC) of different doses in the top-load model. The AUC of the three groups was 987.74 ± 142.84 g/L.h, 1,301.772 ± 258.55 g/L.h, and 1,889.777 ± 309.7 g/L.h, in the low-, medium- and high-dose groups, respectively. The increase of AUC was also directly proportional to the increase in dose (R2 = 0.999).
Figure 3. Area under the plasma concentration–time curve (AUC) in the top-load, 50% exchange transfusion, and haemorrhagic shock models. (A) AUC of different dose groups in the top-load model. AUC was directly proportional to increases in dose (R2 = 0.999). (B) AUC in three different models. ***p < .0005 in comparison to the 50% exchange transfusion model. #p < .05 in comparison to the 5.52 g/kg group in the top-load model. $$p<.005 in comparison to the 2.7 g/kg group in the top-load model. &&p < .005 in comparison to the haemorrhagic shock model. &&&&p < .00005 in comparison to the haemorrhagic shock model.
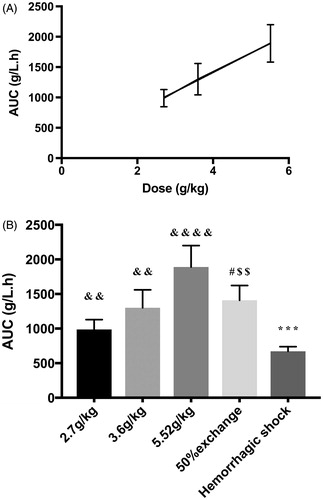
The half-life (T1/2) of pPolyHb in the top-load model is shown in . The values were 12.28 ± 3.55 h (low dose), 16.15 ± 2.264 h (medium dose) and 17.1 ± 3.058 h (high dose). There were no significant differences for T1/2 between these three dose groups.
Figure 4. Half-life (T1/2), apparent volume of distribution (Vd), and clearance (CL), in the top-load, 50% exchange transfusion and haemorrhagic shock models. Based on the plasma concentration–time curve, the non-compartmental method was employed using DASver2.0, which was used to calculate the value of T1/2, Vd, and CL in the three models. (A) Half-life (T1/2) in the three models. *p < .05 in comparison to the 3.6 g/kg group in the top-load model, **p < .005 in comparison to the 3.6 g/kg group in the top-load model. #p < .05 in comparison to the 5.52 g/kg group in the top-load model. ##p < .005 in comparison to the 5.52 g/kg group in the top-load model. (B) Apparent volume of distribution (Vd) in the three models. **p < .005 in comparison to the haemorrhagic shock model. #p < .05 in comparison to the 3.6 g/kg group in the top-load model. $$p < .005 in comparison to the 5.52 g/kg group in the top-load model. &&p < .005 in comparison to the 2.7 g/kg group in the top-load model (C) Clearance (CL) in the three models. **p < .005 in comparison to the 50% exchange transfusion model.
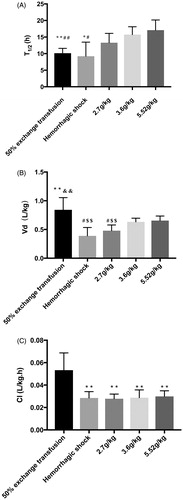
The apparent volume of distribution (Vd (L/kg)) in the top-load model is illustrated in . The value of Vd was 0.479 ± 0.996 L/kg (low dose), 0.632 ± 0.065 L/kg (medium dose), and 0.656 ± 0.789 L/kg (high dose). The low value of Vd indicated a limited distribution from the central compartment to the peripheral compartment of these three dose groups.
shows the plasma clearance (CL (L/(kg·h)) of different dose groups in the top-load model, which was 0.028 ± 0.004 L/kg·h (low dose), 0.0288 ± 0.007 L/kg·h (medium dose), and 0.030 ± 0.005 L/kg·h (high dose). There were no significant differences between these three dose groups.
Exchange transfusion model
The concentration–time curve of the exchange transfusion model is shown in . As can be seen in , Cmax (83.695 ± 6.655 g/L) in the exchange transfusion model was instantly achieved; this was the highest Cmax of the three models. There were significant differences in Cmax between the exchange transfusion model and two doses of the top-load model (2.7 g/kg and 3.6 g/kg).
As shown in , the area under the concentration–time curve (AUC) of the exchange transfusion model was 1410 ± 95.59 g/L.h. There were significant differences in AUC between the exchange transfusion model and the 5.52 g/kg and 2.7 g/kg doses in the top-load model. There were no significant differences in AUC between the exchange transfusion model and the 3.6 g/kg dose in the top-load model.
shows that the half-life (T1/2) in the exchange transfusion model was 10.14 ± 0.651 h. There were significant differences for T1/2 between the exchange transfusion model and the 3.6 g/kg and 5.52 g/kg doses in the top-load model.
As shown in , Vd (L/kg) of the exchange transfusion model was 0.842 ± 0.951 L/kg, which was also the highest of the three models. There were significant differences for Vd between the exchange transfusion model and the haemorrhagic shock model and 2.7 g/kg group in the top-load model. No significant differences for Vd were seen between the exchange transfusion model and the 3.6 g/kg and 5.52 g/kg doses in the top-load model.
Plasma clearance (CL (L/(kg·h)) in the exchange transfusion model was 0.053 ± 0.007 L/(kg·h), the highest one of the three models. Therefore, the retention of pPolyHb in plasma under this condition was the shortest. There were significant differences between the CL in the exchange transfusion model and different dose groups (2.7, 3.6, 5.52 g/kg) in the top-load model ().
Haemorrhagic shock model
The concentration–time curve of the haemorrhagic shock model is shown in . Results showed that the concentration of pPolyHb gradually declined until 48 h after transfusion, when the pPolyHb concentration could not be measured. Cmax was 49.927 ± 5.787 g/L, significantly lower than in the exchange transfusion model. There were no significant differences in Cmax between the haemorrhagic shock model and the 2.7 g/kg and 3.6 g/kg groups in the top-load model ().
As shown in , the AUC of the haemorrhagic shock model was 672.867 ± 67.511 g/L.h, the lowest of the three models. Significant differences in AUC were noted between the haemorrhagic shock model and the other models.
The half-life (T1/2) of the haemorrhagic shock model was 9.213 ± 4.26 h, which was significantly lower (0.86-fold) than the T1/2 of pPolyHb in the 5.52 g/kg dose of the top-load model (17.1 ± 3.058 h) and the lowest T1/2 of the three models. There were no significant differences in T1/2 between the haemorrhagic shock model and the 50% exchange transfusion model or the 2.7 g/kg dose in the top-load model ().
As shown in , the Vd (L/kg) of the haemorrhagic shock model was 0.388 ± 0.146 L/kg, the lowest of the three models. This was similar to the 2.7 g/kg group in the top-load model. Significant differences in Vd were seen between the haemorrhagic shock model and the 50% exchange transfusion model.
CL (L/(kg·h)) in the haemorrhagic shock model was 0.030 ± 0.003 L/kg·h, as indicated in . There were no significant differences between the CL of the haemorrhagic shock model and different doses in the top-load model.
Discussion
In this study, three animal models were established (the top-load model, the 50% exchange transfusion model, and the 60% haemorrhagic shock model) to mimic different clinical situations and identify the most suitable clinical indications for pPolyHb. A series of pharmacokinetic parameters that reflected the absorption, distribution and metabolism of pPolyHb in rats were established by detecting the concentration of pPolyHb at different time intervals. These pharmacokinetic parameters included the maximum concentration (Cmax), the area under the plasma concentration-time curve (AUC), the elimination half-life (T1/2), the clearance (CL) and the apparent volume of distribution (Vd). Among them, Cmax and AUC mainly reflected the absorption characteristics of pPolyHb, while the distribution and metabolism characteristics of pPolyHb were represented by three values of CL, Vd and T1/2.
Since pPolyHb was infused intravenously, Cmax was instantly achieved in all three models. In the top-load model, the peak concentration increased proportionally with increases in dose. Cmax in the 50% exchange transfusion model was the highest of the groups, and there were no significant differences in Cmax between the 50% exchange transfusion model and the 5.52 g/kg group in the top-load model.
AUC was defined as the area under the plasma concentration–time curve, which indicates the amount of drug (pPolyHb) to which the animal was exposed. Therefore, AUC represented the degree and amount of pPolyHb absorption. By calculating the area under the plasma concentration time curve, it was shown that AUC exhibited the same pattern as Cmax in the top-load model. The value of AUC increased proportionally with increases in dose. In the three animal models, the highest AUC was observed in the top-load model at a dose of 5.52 g/kg, indicating that rats had the highest absorption of pPolyHb in this condition; AUC was the lowest for the 60% haemorrhagic shock model. Because the value of pPolyHb in AUC and Cmax was proportional to the dose administered, this study showed that the process of elimination or absorption of pPolyHb in rats followed first-order kinetics and that the kinetic process of pPolyHb in rats conformed to linear pharmacokinetics. This conclusion will inform future research.
The elimination half-life (T1/2) indicates the time required to halve the end-phase plasma concentration. This parameter visually reflects the rate of drug elimination in animals. There were no significant differences in elimination half-life of pPolyHb at various doses in the top-load model, which was consistent with the linear pharmacokinetic profile. However, the elimination half-life of pPolyHb in the 50% exchange transfusion model and the 60% haemorrhagic shock model were lower than in the top-load model. Because the infusion of pPolyHb and haemorrhage in the 50% exchange transfusion model were carried out simultaneously, the partial loss of pPolyHb may be due to the process of blood loss. This may have resulted in an elimination half-life derived from the final concentration of pPolyHb in vivo that was less than the half-life of pPolyHb in the top-load model. In the 60% haemorrhagic shock model, rats were in a shock state, which may explain the reduced half-life of pPolyHb.
Clearance (CL) refers to the amount of plasma cleared by the organ within a unit of time, and indicates the amount of drug-containing plasma which is cleared per unit time in an animal. In the top-load model, the clearance did not change significantly with increasing doses. This is also consistent with the characteristics of linear pharmacokinetics. In the 50% exchange transfusion model, drug clearance was the highest, and it was significantly higher than the other two models. This indicated that the shortest residence time of pPolyHb in rats was in the 50% exchange transfusion model. This may be due to the partial loss of pPolyHb from the rat during the blood loss process. In the 60% haemorrhagic shock model, the clearance was the same as that in the top-load model, and there were no significant differences between groups.
The steady-state apparent volume of distribution (Vd) is the proportional constant of the in vivo dose and the plasma concentration when the drug reaches homoeostasis in vivo. Vd can reflect the wide range of drug distribution in the body, the higher the value, the more widely the drug is distributed. The value of Vd is mainly used to estimate the amount of blood and systemic drugs. The higher the concentration of the free drug in the blood, the higher the drug level of the target, thus determining the adverse effect of the drug. Therefore, predicting and analysing Vd is a valuable tool for evaluating drug efficacy. The body fluid of rats is approximately 0.5 ∼ 0.8 L/kg. If the apparent volume of distribution is higher than the body fluid, drug accumulation in tissues may exist. In this study, the values of Vd were less than 0.5 L/kg in the top-load model at a dose of 2.7 g/kg and in the 60% haemorrhagic shock model, and the value of Vd was the smallest in the 60% haemorrhagic shock model. These results suggest that the distribution and metabolism of pPolyHb in both circumstances was predominantly in body fluids, and that pPolyHb may have the highest targeting in the 60% haemorrhagic shock model. The Vd value was the largest in the 50% exchange transfusion model, and there were no significant differences between the 3.6 g/kg and 5.52 g/kg groups in the top-load and the 50% exchange transfusion model. However, the values of Vd fluctuated around 0.8 L/kg, demonstrating that pPolyHb was mainly distributed in body fluids in rats, with little accumulation in tissues or organs.
Conclusions
The results showed that absorption and elimination of pPolyHb was consistent with the laws of linear pharmacokinetics in vivo. The extent and the amount of the absorption of pPolyHb increased in proportion to increases in dose. The half-life of pPolyHb in rats was longer and did not significantly change under non-pathological conditions. However, in the case of blood replacement and haemorrhagic shock, the half-life of pPolyHb may decline to a certain degree, which may be due to the pathological conditions. These results will aid in the determination of a reasonable administration interval under clinical situations such as trauma and massive blood loss. The clearance of pPolyHb was low in all three animal models, demonstrating longer residence of pPolyHb in rats, which is a potentially useful drug attribute. The apparent volume of distribution of pPolyHb in rats was less than 0.8 L/kg, in a majority of all three models. Of note, the haemorrhagic shock model had a minimum Vd, demonstrating a targeted role for pPolyHb in rats with little accumulation in tissues. These results provide guidance for the future administration of pPolyHb in clinical trials.
Acknowledgement
We would like to thank LetPub (www.letpub.com) for providing linguistic assistance during the preparation of this manuscript.
Disclosure statement
No potential conflict of interest was reported by the authors.
Additional information
Funding
References
- Hess JR. Red cell changes during storage. Transfus Apher Sci. 2010;43:51–59.
- Kim HW, Greenburg AG. Toward 21st century blood component replacement therapeutics: artificial oxygen carriers, platelet substitutes, recombinant clotting factors, and others. Artif Cells Blood Subst Immunobiol Biotechnol. 2006;34:537–550.
- Taguchi K, Maruyama T, Otagiri M. Pharmacokinetic properties of hemoglobin vesicles as a substitute for red blood cells. Drug Metab Rev. 2011;43:362–373.
- Tinmouth A, et al. Clinical consequences of red cell storage in the critically ill. Transfusion. 2006;46:2014–2027.
- Lelubre C, Vincent JL. Relationship between red cell storage duration and outcomes in adults receiving red cell transfusions: a systematic review. Crit Care. 2013;17:R66.
- Chang TMS. Blood replacement with nanobiotechnologically engineered hemoglobin and hemoglobin nanocapsules. Wires Nanomed Nanobiotechnol. 2010;2:418–430.
- Liu H, Yan C, et al. Study of the effects of polymerized porcine hemoglobin (pPolyHb) in an acute anemia rat model. Artif Cells Nanomed Biotechnol. 2016;45:694–700.
- Zhu H, Dang X, et al. Pharmacodynamic study of polymerized porcine hemoglobin (pPolyHb) in a rat model of exchange transfusion. Artif Cells Blood Subst Biotechnol. 2011;39:119–126.
- Emanuela S, Pierantonio M, et al. Low dose anthracycline and risk of heart failurein a pharmacokinetic model of human myocardium exposure: Analog specificity and role of secondary alcohol metabolites. JPET Fast Forward. 2017;39:217–223.
- Edlund H, et al. Pharmacokinetics and pharmacokinetic–pharmacodynamic relationships of monoclonal antibodies in children. Clin Pharmacokinet. 2014;54:35–80.
- Turck D, et al. Pharmacokinetics of meloxicam in patients with end-stage renal failure on haemodialysis: a comparison with healthy volunteers. Eur J Clin Pharmacol. 1996;51:309–313.
- Morgan JL, et al. Pharmacokinetics of amlodipine besylate at delivery and during lactation. Preg Hypertens. 2018;11:77–80.
- Buehler PW, et al. Synthesis, biophysical properties and pharmacokinetics of ultrahigh molecular weight tense and relaxed state polymerized bovine hemoglobins. Biomaterials. 2010;31:3723–3735.