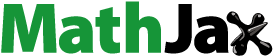
Abstract
Regeneration of peripheral nerve defects remained a remarkable clinical challenge. Engineered nerve conduits represent a promising strategy to improve functional recovery in peripheral nerve injury repair. However, nerve conduits require additional factors such as neurotrophic factors to create a more conducive microenvironment for nerve regeneration. Neurotrophic factors have well-demonstrated abilities to improve neurite outgrowth, making them great candidates for repairing of defected nerves. To this end, we examined the beneficial effects of repairing the transected rat sciatic nerve by loading of nerve growth factor (NGF) in nerve conduits. The PCL/Collagen/NBG conduits were interposed into the 10 mm right sciatic nerve defects. Twenty-four rats were randomly allocated into four groups: 1- nerve autograft group, 2- a nongrafted group with gap 10-mm, 3- conduit group and 4- the conduits loaded with NGF. Motor and sensory functional recovery, the evoked muscle action potential, and motor distal latency showed significant improvement in rats treated with NGF. The histology and immunohistochemistry studies revealed less fibrosis and a high level of expression of CD31 and NF-200 protein at the crush site in the Conduit + NGF group. In conclusion, the PCL/Collagen/NBG conduit loaded with NGF, which exhibited nanometer-scale features, neurotrophic activity, favorable mechanical properties and biocompatibility could improve sciatic nerve regeneration in rats.
Introduction
Peripheral nerve injury is a serious health problem in today’s society that can lead to permanent disability [Citation1]. Peripheral nerve reconstruction remains a medical challenge. Recent clinical treatments, especially for large nerve gaps, involve the utilization of autografts and suturing of the gaps. Although, this method is in most cases ineffective because of the mismatch of sizes between the donor and recipient tissues, donor site morbidity, loss of function at the donor sites and complex surgical procedures [Citation2]. Neural tissue engineering is one of the most promising ways of treating nerve damage [Citation3]. Therefore, To seek alternatives for autografts, the development of artificial nerve guidance conduits (NGCs) is ideal as an alternative [Citation4,Citation5]. For artificial NGCs, remarkable efforts have been made directed by the aim to best mimic components and the structures of autologous nerve. With the progress of fabricating methods during the past decades, structures of nerve channels have been greatly improved to satisfy various kinds of requirements including nanofibrous and porous channel wall with proper permeability and degradability, along with ideal mechanical properties in resisting collapse and stretch forces when applied in vivo [Citation4]. Electrospinning is a prominent strategy to make nanofibrous conduits able to assure tailored porosity, degradation rate, suitable mechanical properties and large surface areas for suitable cell attachment. This unique feature on structure promotes extensive exploration on its use for neural tissue engineering [Citation6].
In ensuring the success of neural tissue engineering, material selection plays a decisive role as specified by Ezra et al. [Citation7]. By tailoring the material degradation rates and mechanical features, it is possible to reduce the inflammatory response, thus improving the guidance and support to preserve axon regeneration [Citation8]. Poly (ε-caprolactone)(PCL) as a synthetic material in combination with a natural polymer of collagen are attractive as neural tissue engineering conduits to obtain a scaffold with favorable cell adhesion, tailored degradation rate, appropriate mechanical and surface properties [Citation9]. On the other hand, bioceramics are usually used combined with different polymers to obtain the best possible biological and mechanical properties. The characteristics of nanobioglasses (NBG) include excellent bioactivity, ability to deliver cells and controllable biodegradability to release ions during the degradation process [Citation10]. While bioactive glasses have been used widely for bone regeneration, little research has been done on the application of bioactive glasses to the regeneration of soft tissues, peripheral nerve regeneration [Citation3]. Also, recent studies indicate that low concentrations of 45S5 bioactive glass induce angiogenesis, which is necessary for multiple applications in tissue regeneration and the healing of soft tissue wounds [Citation11].
The limited success of conduits may be attributed to the lack of efficient microstructure, biologically active molecules for guiding the growth of regenerating nerves and enhancing axonal regeneration. In fact, conduits are not only an empty channel which allows the regenerated nerve to pass through [Citation12,Citation13]. The neurotrophic factors are the first candidate for improving nerve regeneration. Neurotrophic factors belong to several distinct protein families, among which the neurotrophin family consists of nerve growth factor (NGF), brain-derived neurotrophic factor (BDNF) and the neurotrophins NT-3 and NT-4/5 [Citation14]. The recent use of artificial NGCs fabricated with suitable biomaterials and filled with a variety of with neurotrophic factors such as NGF, neurotrophin NT-3 and glial cell line-derived neurotrophic factor(GDNF) has been shown enhanced nerve regeneration across gaps [Citation15]. In fact, neurotrophic activity is essential for regeneration of injured nerve tissues and plays an important role in neuron survival, differentiation and maintenance of synaptic activities [Citation2]. NGFs are as an important member of neurotrophin family that play an important role in the survival, and neurite outgrowth of target neurons, both in vitro and in vivo, but also enhances peripheral nerve regeneration, as shown in many previous types of research [Citation13,Citation16]. In previous research, it has proven that NGF stimulates and regulate neurite outgrowth of sensory neurons by specific receptor tyrosine kinases trkA, trkB or trkC [Citation17]. NGF can be physically absorbed into nerve channels to be used for nerve regeneration. In this study, NGF suspended in fibrin conduits as a support to improve engraftment. Accordingly, the basic aims of this study were to investigate the neurodegenerative properties of poly (ε-caprolactone)/collagen/nanobioglass (PCL/Collagen/NBG) nanofibrous conduits loaded with NGF, on sciatic nerve regeneration across a 10-mm-long gap.
Materials and methods
Materials
PCL (Mw 80,000 g/mol), phosphate-buffered saline (PBS), fetal bovine serum (FBS), trypsin–EDTA, 3–(4, 5-dimethylthiazol-2-yl)-2, 5-diphenyl tetrazolium bromide (MTT), 40, 6-diamidino-2-phenylindole (DAPI) and collagenase I were purchased from Sigma-Aldrich. Dulbecco’s Modified Eagle’s Medium (DMEM) was obtained from Invitrogen (Carlsbad, CA). Also, glacial acetic acid was prepared from Merck. Collagen I samples were extracted from rat tails for electrospinning technique and preparation of porous nanofibrous conduits. Nerve growth factor (NGF) was purchased from Millipore, Singapore.
Production and characterizations of the nanofibrous conduits
Nanofibrous tubular nerve conduits were fabricated by electrospinning with a polymer blend of PCL, collagen type I and 45S5 NBG as described previously [Citation3]. Briefly, the nerve conduits were electrospun using a polymer blend of PCL (10% weight per volume, wt/vol), collagen type I derived from rat tail (5% weight per volume, wt/vol) and NBG (2% weight per volume, wt/vol) in acetic acid to obtain a PCL (70)/Collagen (20)/NBG (Citation10) weight percent composition. The electrospinning set-up included a syringe pump, a high-voltage supply, and a rotating mandrel with 2 mm diameter. A positive voltage (between 15 and 18 kV) was applied to the system using a power supply. The PCL/collagen/NBG blend solution was delivered through a 22-gauge blunt tip syringe needle at a constant flow rate of 1 ml/h using a syringe pump. The distance between the syringe tip and the mandrel was 8 cm, and the rotation rate of the mandrel was approximately 1000 rpm. Nerve conduits had an external diameter of 1.8–2 mm. The internal diameter was found to 1.6 mm and 12 mm length. After the spinning of fibers, the PCL/Collagen/NBG nerve conduits stabilized by cross-linked in the vapor of a 2.5% glutaraldehyde solution at room temperature for 6 h. Qualitative evaluation of fiber morphology of the electrospun conduits was performed by field emission scanning electron microscopy (SEM-Philips XL30). The nanocomposite samples were coated with gold using an (EMITECH K450X, England) Sputter before examination under the SEM that operated at the acceleration voltage of 15 kV. On selected SEM images, the fiber diameter distribution and cross-sectional pore size were determined by using ImageJ software (Image J, National Institutes of Health, Bethesda, MD).
Isolation and culture of human endometrial stem cells
Human endometrial stem cells (hEnSCs) were isolated as we described previously in our papers [Citation18]. Briefly, the biopsy tissue was digested in Collagenase type I (1 mg/ml, Sigma-Aldrich) for 60 min at 37 °C. The resultant cell suspension was filtered through 70- and 40-μm cell strainers and centrifuged to separate the supernatant from the pellet. The isolated cells were suspended in Dulbecco’s modified Eagle’s medium (DMEM)/F12 medium containing 10% FBS and 1% antibiotic penicillin/streptomycin (P/S; Sigma-Aldrich) and then incubated at the 37 °C incubator in 5% CO2 in the air. When the cells had reached 80% confluence, they were trypsinized and halved for characterization by flow cytometry. HEnSCs at passage 3 were used for the experiments.
Cell adhesion and morphology analysis on the nanofibrous conduits after cell seeding
Prior to the cell seeding, nanofibrous conduits were sterilized by exposure to ultraviolet (UV) radiation for 1 h and incubated with DMEM/F12 containing 10% FBS for 24 h. Then, 100 μL of cell suspension was further seeded onto nanofibrous conduits, placed in a 24-well plate at a density of 5 × 104 cells/well and incubated at 37 °C in 5% CO2 for 2 h. In order to the better attachment of hEnSCs to the surfaces of the conduits, 500 μL DMEM/F12 containing 10% FBS was added to each well and incubated for 72 h. The cellular morphology of seeded cells onto conduits was evaluated by SEM at the third day of the culture period. The samples were fixed in 2.5% glutaraldehyde for 1 h. Afterward, they rinsed three times in PBS, and dehydrated in graded alcohols concentrations; 30, 50, 70, 80, 90 and 100% at 37 °C for 10 min per concentration. Ultimately, Images were examined using an SEM (Philips XL-30, Netherlands) at an accelerating voltage of 15 keV.
Cell viability and proliferation study
To quantify the number of viable cells next to conduits, hEnSCs were seeded on nanofibrous conduits, and metabolic activity of the hEnSCs was measured with using 3–(4,5-dimethyl thiazolyl-2-yl)-2,5-diphenyltetrazolium bromide (MTT) assay on day 1, 3, 5 and 7 as described previously [Citation3]. On the other hand, using phenylindole (DAPI) staining, the cell attachment was evaluated. HEnSCs were seeded onto the nanofibrous conduits and cultured in DMEM/F12 for 1, 3, 5 and 7 days. After fixation and DAPI staining, the images of stained cells were captured using a confocal fluorescent microscope (Nikon, Melville, NY) and cell growth on the conduits evaluated.
NGF loading on nanofibrous conduits
To prepare fibrin gel, NGF with a concentration of 100 ng/ml were mixed with 3 mg/ml bovine fibrinogen (Sigma) and dissolved in M199 Media (Sigma).The fibrinogen- the NGF solution was converted to a gel by adding 15 µL thrombin (50 U/ml in sodium buffer 1 M, Sigma), then the mixture injected into nanofibrous conduits. The nanofibrous conduits were put in an incubator at 37 °C for 1 h to form a three-dimensional network structure.
In vivo studies
Sciatic nerve defect
A total of 24 adult male Wistar rats were used in this study to evaluate the nerve regeneration. The rats were randomly divided into 4 groups (n = 6 rats). (1) Nerve autograft group (Positive Control) that reversed autografts were interposed into the 10-mm nerve gap, (2) nerve defect group with 10 mm gap without treatment (Negative Control), (3) nerve conduits group alone (Conduit) and (4) nerve conduits group with NGF injected into their lumen (Conduit + NGF). The rats were deeply anesthetized with a combination of ketamine (60 mg/kg) and xylazine (10 mg/kg) given by intraperitoneal injection throughout the surgical procedure. Surgery was conducted on the rat’s right sciatic nerve, as the experimental side. Under aseptic conditions, the sciatic nerve was exposed via a posterior, right thigh muscle-splitting incision. An operating microscope, the right sciatic nerve was severed into proximal and distal segments at the center of the right thigh. Both proximal and distal stumps were anchored with 7–0 nylon to a depth of 1 mm into the conduits, leaving a 10 mm gap between the stumps and the skin which was closed with 6–0 silk. Then, a 12 mm long guidance conduit was used to bridge the gap, adjoin the proximal and distal nerve stumps with both ends entering the conduit by 1 mm. In the nerve autograft group, a 10 mm nerve segment was resected, then reversed 180° and sutured back into the nerve. In the negative control group, there was no conduit to bridge the created defect. All rats were housed in a temperature and humidity controlled room, with 12 h light/dark cycles and easy access to standard food and water. All rats were sacrificed at 12-week postsurgery. Assessment of sciatic nerve regeneration was performed by sciatic function index, hot plate test, neural electrophysiological examination as well as histological and immunohistochemical evaluation.
Electromyographic studies
Electromyography (EMG) was performed on all rats prior to sacrifice at 12-week postoperatively. The electrophysiological response was characterized by calculating the evoked muscle action potential (EMAP) and the motor distal latency (DL) using an electromyographic recorder system (Negarandishegan, Tehran, Iran). The animals were anesthetized by intraperitoneal injection of ketamine and xylazine again and the sciatic nerves were exposed. Adipose and fibrous tissues next to sciatic nerve trunk were removed. The sciatic nerve proximal to the site of the cut was stimulated with an electric stimulus (3–5 mA) using needle electrodes. In order to remove any possible interference, a ground electrode was placed in surrounding muscle tissues. The gastrocnemius response was recorded from the needle and cap electrodes located in the gastrocnemius muscle (filtering frequency of 10 Hz to 10 kHz, a sensitivity of 2 mV/division and sweep speed of 1 ms/division). The distance between the place of stimulation and the muscle was 2 cm which was kept constant for the frequent experiments.
Walking-foot-print analysis
Four, 8 and 12 weeks post-operatively, the rats’ footprints were recorded for the analysis of the sciatic functional index (SFI) as described previously [Citation19]. The rats’ hind feet were dipped in the ink and they were placed inside a wooden walking corridor (100 × 20 × 15 cm) lined with a white millimeter paper and ended with a darkened goal box. SFI was calculated using the following equation where E and N are the experimental and normal foot, respectively; PL is the distance from the heel to the top of the third toe, TS is the distance between the first and the fifth toe and IT is the distance from the second to the fourth toe. This process has repeated a minimum of 3 times until obvious footmarks were obtained. Results in a value around zero imply the normal function while −100 represented the total impairment.
Hot plate latency test
The hot plate latency (HPL) is usually used for evaluating the sensory function and thermal pain sensitivity. Four, 8 and 12 weeks postsurgery, the rats were evaluated for hot plate latency test by placing their damaged limbs in the center of an open-ended cylindrical space on a hot plate (56 °C) and recording the time(in seconds) until they jumped or licked their paws. Along with seeing the response, the rats were removed from the hot plate. The time elapsed from the onset of hot plate contact to a withdrawal of hind limb was measured by a timer and recorded as withdrawal reflex latency (WRL). The cut off time for their reaction was set at 12 s. The affected right limbs were tested 3 times, with an interval of 10 min between consecutive tests to avoid sensitization and the 2 latencies were averaged to get the final result.
Histological and immunohistochemical examination
Twelve weeks after implantation, the rats were sacrificed with an overdose of ketamine (200 mg/kg), then the distal-section of the sciatic nerves was removed and immediately fixed in a 10% buffered formalin, dehydrated in a graded series of ethanol solutions and finally embedded in paraffin, cut into 2–4 µm thickness and stained with hematoxylin–eosin (H and E) and Luxol fast blue (LFB) for light microscopy examination (Nikon, 50i, Japan). In stained sections, regenerated nerve tissues in the conduits were examined. Sections were evaluated according to the following criteria: nerve growth from proximal and distal, inflammation (parenchyma infiltration of inflammatory cells), vascularity and rate of vacuolation. Demyelination was examined by LFB which stains the myelin blue.
Distal segments of the regenerated nerve conduits were analyzed with immunohistochemical methods 12-week postsurgery. Double immunochemistry labeling was performed to visualize axons using NF-200 and the degree of angiogenesis using CD31 antibody according to a standard immunohistochemical protocol. Briefly, after permeation and blocking of non-specific binding, sections were incubated with two primary antibodies: mouse anti-NF200 (1:100) and mouse anti CD31 (1:100; Abcam, Cambridge, MA). Afterwards, Sections were washed in PBS and incubated at room temperature for 2 h with secondary antibody: goat anti-mouse IgG secondary antibody (1:200). Then, DAB followed by the counterstaining of haematoxylin was added for coloration. Finally, the immunostained samples were mounted onto glass slides and viewed under an optical microscope.
Statistical analysis
The data were expressed as Mean ± SD (standard deviation). The data at the various time points were statistically analyzed by SPSS 11.0 software (SPSS, Chicago, IL) using one-way analysis of variance (ANOVA) and the Tukey post hoc test. The significance level was set at p < .05.
Results
Evaluation of microstructures of nanofibrous conduits
displays the SEM micrographs of electrospun conduits obtained from the collagen/PCL/NBG blends. It is obvious that the electrospun nanofibrous conduits are composed of fibers without beads with average diameters of 353.5 nm and a pore size of 1.1 µm. The mandrel design and the volume of polymer solution could control the diameter and thickness of the conduits. The fabricated conduits had an inner diameter of 1.6 mm with the external diameter between 1.8–2 mm and a length 12 mm. On the other hand, at 3 days of culture, Human EnSCs proliferated on the conduits (). Nanofibrous conduits were found to support adhesion and cell proliferation due to mimic the extracellular matrix structure (ECM).
Cytotoxicity and proliferation assay
The cultured cell nuclei were stained with 4′, 6′-diamidino-2-phenylindole (DAPI) to evaluate cell viability. For this purpose, hEnSCs were seeded onto the nanofibrous conduits and cultured in DMEM/F12 for 1, 3, 5 and 7 days. Results of DAPI staining showed an increase the attachment and proliferation of live cells on the conduits over time and it was in agreement with SEM observation (). MTT assay was used to measure the viability of hEnSCs cultured on the nanofibrous conduits and tissue culture polystyrene (TCP) at 1, 3, 5 and 7 days as 3D and 2D cultures, respectively (). Until day 3, the cells cultured on TCP showed higher viability than cells cultured on the PCL/collagen/NBG conduits. However, in days 5 and 7, the viability of cells cultured on PCL/collagen/NBG conduit significantly enhanced relative to cells cultured on the 2 D group. These results showed that PCL/collagen/NBG nanofibrous conduits were suitable substrates than TCP in relation to proliferation and attachment of hEnSCs. This was reaffirmed by DAPI staining. Collagen and NBG particles enhance the hydrophilicity of conduits and prepare suitable condition for cellular adhesion and proliferation.
Animal model
The sciatic nerve is the most common nerve to evaluate the regeneration of peripheral nerves in vivo tests. Because it has enough space and length to implant a graft and manipulate a surgery. In this experiment, we used adult Wistar rats to test the efficacy of electrospun collagen/PCL/NBG conduits in bridging a 10 mm sciatic nerve defect gap. Three months post-operatively, the rats of the all of the groups (except the negative control group) were understood to have recovered from foot ulcers on the right side. No serious chronic inflammatory reaction or adverse tissue reactions were seen. Also, it was found that the nerve conduits did not adhere to the surrounding tissues and were easily removed for histological testing. In a negative control group, no regenerated nerves between proximal and distal nerve stumps were observed ().
Figure 3. Position of the sciatic nerve in the posterior compartment of the thigh of the rat (A), Surgical implantation of a collagen/PCL/NBG nerve conduit bridging a 10 mm sciatic nerve defect in rats (B), view of the transplanted conduit (C) and the view of the regenerated nerve 3 months post-surgery (D).
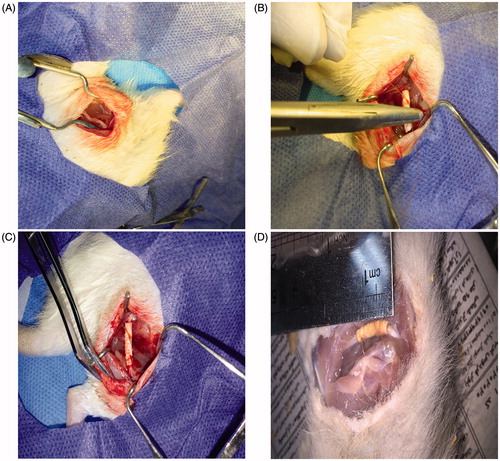
Electromyographic studies
To determine whether the functional reinnervation happened via the nanofibrous fabricated conduits or not, the electrophysiological analysis was accomplished. In an electrophysological study, at 12-week postimplantation, there were no significant differences between autograft with conduit and Conduit + NGF group in amplitude and latency evaluations (p > .05). Also, a result of amplitude measurement existed significance between Negative Control group with autograft, Conduit alone and Conduit + NGF group (***p < .001). The same statistical results were found in the analyses of latency (***p < .001 and **p < .01). In Negative Control group, very low signals were detected and no muscle contractions were found. Results demonstrated conduit alone and Conduit + NGF reached a similar level of muscle reinnervation as autograft, but still was in a pre-mature stage to be improved towards normal nerve functions. Comparison of electrophysiological data (EMAP and DL) was shown in .
Figure 4. Evoked muscle action potential (EMAP) and latency (DL) of autograft, Negative Control group, Conduit and Conduit + NGF group after 12 weeks implantation in rats compared with each other. There were no significant differences between autograft group with Conduit and Conduit + NGF group (p > .05). Significance was seen between Negative Control group with autograft, Conduit alone and Conduit + NGF group. ap < .05 compare with Negative Control group; ap < .01 compare with Negative Control group; ap < .001 compare with Negative Control group.
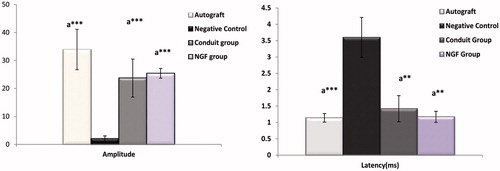
Walking-foot-print analysis
shows the average SFI values and the injured foot walking pattern of all study groups at 4, 8 and 12-week post surgery. The walking-track analysis showed better functional recovery results in the autograft group. The autograft, as the gold standard of nerve bridging, showed improved SFI from −59.44 ± 8.45 at the end of the 4th week to −57.51 ± 4.46 and −54.75 ± 3.5 at the end of 8th and 12th weeks, respectively. There was a significant difference between the Conduit and Conduit + NGF groups with the autograft group at the end of the first month. But 1 month later, the SFI value of both Conduit and Conduit + NGF group increased, so that after the third month, there was no significant difference between the Conduit and Conduit + NGF groups compared with autograft group. Results indicated both groups (Conduit and Conduit + NGF) reached a similar level of motor recovery as the autograft. After the twelfth week, the order of SFI value from high to low was: autograft group (−54.75 ± 3.5), Conduit + NGF group (−63.38 ± 5.04), Conduit group (−64.01 ± 5.79), and Negative Control group (−87.25 ± 2.27). There was obvious a difference between the Conduit and Conduit + NGF groups with Negative Control group (**p < .01 and ***p < .001) in times of 4w, 8w and 12w, indicating the Negative Control group had very little improvement and little motor recovery compared with nerve conduits alone and conduit loaded with NGF.
Hot plate latency test
The results of the hotplate test are shown in . Four weeks after surgical transection, most rats respond in the hotplate test by licking their hind paws and the WRL dropped to 12 s. This delay matched the time required to see the bridging of the regenerating nerve axons across a gap of 10 mm, with an approximate mean rate of regeneration of 0.2 mm per day [Citation20]. At the end of the fourth week, significant differences were found between group's autograft, Conduit and Conduit + NGF compared with Negative Control group (***p < .001). Also, NGF treated rats had significantly better responsiveness than the autograft group (*p < .05). Afterward, the WRL steadily improved during the 12-week recovery time. Twelve weeks after injury, the withdrawal response displayed better sensory recovery results in group's Conduit + NGF, autograft and conduit respectively and also compared with group Negative Control (***p < .001, *p < .05). However, no statistically significant differences were found between group's Conduit and Conduit + NGF with together and compared with autograft group (p > .05).
Histological and immunohistochemical examination
illustrates H&E staining of along the longitudinal directions and cross-sections cut from the regenerated nerves in different groups at 3 months after surgery. In the negative control group, no remarkable axon regeneration has been observed after 12 weeks implantation (not shown). In the treated group with NGF, the vacuolation was considerably reduced compared with autograft and Conduit groups. The dark spots on the axon sheets usually represent the Schwann cells. These cells with elongated nuclei ensheath axons are visible in all parts of the peripheral nervous system. Among the three experimental groups, in autograft group, the largest number of new capillaries had regenerated. Although, the formation of blood vessels was observed with a lower ratio in the group Conduit alone and Conduit + NGF group, it shows that conduit-forming materials (NBG) cause angiogenesis. Also, a large number of regeneration nerve fibers and improved myelin sheath in Conduit + NGF group similar to autograft group. LFB staining that stains the myelin blue, confirms findings of H&E staining. In this staining, higher demyelination was observed in a group treated with NGF similar to autograft group ().
Figure 7. Hematoxylin/eosin staining of the regenerated nerves in different groups after 12 weeks of Surgery. Autograft group (A, B, C), conduit (D, E, F) and Conduit + NGF groups (J, H, I). Longitudinal directions: A, D, G (×10) and cross-sections: B, E, and H (×20). Long arrows: vacuole degeneration; short arrows: blood vessels).
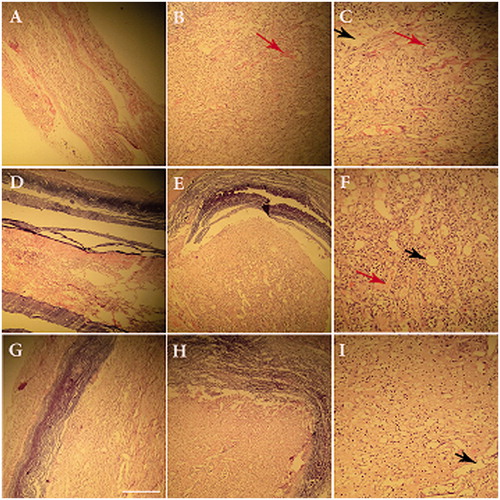
Figure 8. Luxol fast blue staining of the three groups; autograft (A), conduit group (B) and Conduit + NGF group (C) at the end of 12th week postsurgery (×20). (Arrows: vacuole degeneration).
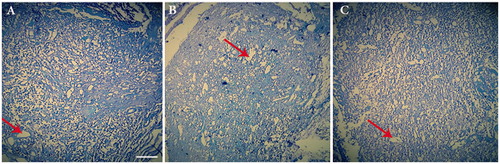
Analysis of axonal neurofilament was performed to measure the amount of regeneration in conduits groups compared with the autograft. Three months after nerve grafting, immunohistochemistry with anti-NF200 showed that a number of myelinated nerve fibers appeared in conduit + NGF group than in conduits group. Actually, a conduit + NGF group displayed NF200-positive axons that were as well as a greater number of positive-stained area (Brown color) in autograft group. Also, weak-positive staining for NF200 in conduit group was seen compared with conduit + NGF group (). By using CD31 staining, the rate of angiogenesis was evaluated in the studied groups. Following immunohistochemistry for CD31, conduit group showed the density of CD31- positive microvessels (more intense brown color) similar to and partly more than the densities in the autograft group 12-week after nerve grafting. In fact, Loaded NGF in the conduit did not have a profound effect on the rate of angiogenesis. These findings suggest that a robust angiogenic response may be achievable exclusively through a biomaterials-based approach ().
Discussion
Peripheral nerves have some capability for regeneration and repair after lesions; wide researches have been done to understand and develop strategies to improve this regeneration process [Citation21]. Until several years ago, autologous nerve graft has been used frequently in the bridging of peripheral nerve defects in a clinic. However, the limited availability and donor-site morbidity such as (scar, neuroma pain) of autografts and disproportion of grafted nerve tissue remain as unavoidable disadvantages [Citation22]. In recent times, enormous effort has been developed the engineered nerve guidance conduits (NGCs) as a powerful strategy in the field of peripheral nerve regeneration [Citation3]. NGCs can act as a bridge, providing directional guidance and opportunities to control the microenvironment as well as biological support to nerve regeneration. Electrospinning technique is the perfect choice to produce nanofibrous NGCs able to assure high porosity and large surface areas for cell attachment and nutrient transportation [Citation6]. Interest towards employing electrospinning for conduits fabrication is mainly due to the mechanical, biological and kinetic properties of the conduit being easily manipulated by changing composition of the polymer and processing parameters [Citation23]. Considering the benefits of collagen and PCL in combination with each other like good biocompatibility, biodegradability, desired cell adhesion and suitable mechanical properties, this compound has been widely used in tissue engineering [Citation24]. On the other hand, recent reports demonstrate that bioactive glasses have proangiogenic potential over a limited range, and this mass of material could be loaded onto a three-dimensional biomaterial for localized and sustained delivery [Citation25]. Bioactive glasses widely utilized in many fields for its favorable bioactivity, biodegradability, proper mechanical properties, ability to deliver cells and a profound effect on the growth of the peripheral axons [Citation10,Citation26]. In our previous work, we showed that PCL/collagen/NBG conduits made by electrospinning are as a desirable and new nerve conduit material to enhance nerve regeneration [Citation3].
In order to design an ideal nerve conduit, in addition to the conduit material, incorporation of neurotrophic factors and cells supporting axon renewal should also be considered [Citation15]. Several studies indicated that, after a nerve injury, cell adhesion molecules, neurotrophic factors and stem cell transplantation played very basic roles in nerve regeneration and functional recovery [Citation21,Citation27]. NGF is one type of cell growth factors, which play a very important role in neuronal development, as well as the survival of sympathetic, sensory neurons and the cell bodies during the regeneration of the axons [Citation28].
Some studies have used composite NGF nerve conduits as an NGF carrier to the lumen of a different neural tube, resulting in increased peripheral nerve regeneration across a defect [Citation12,Citation29,Citation30]. However in practice, NGF simply solved in organic solvents and it is rapidly diffuse in extracellular fluids. As a result, it is difficult to keep it in injury site. On the other hand, periodic injection of NGF is an expensive and impractical for clinical application and excessive doses may also evoke unfavorable side effects [Citation31]. One of the effective strategies to solve these problems is to incorporate NGF into polymeric materials and then implant directly into the target tissue site [Citation32]. Since ECM is known as a reservoir for the neurotrophic factors, components of ECM such as collagen are appropriate targets for the transfer of exogenous neurotrophic factors [Citation30]. Wenji Sun et al. showed that collagen membranes loaded with the collagen binding NGF maintained a higher concentration and stronger bioactivity of NGF on the collagen scaffold and enhanced the nerve growth into this scaffold in vivo study [Citation30]. Also, it has been shown that fibrin sealant could easily be used in conjunction with sutures to gradually releases the neurotrophic substances to the damaged nerve and to enhance recovery of nerve function [Citation33]. Neurotrophins, by binding to their specific receptors, activate important intracellular signaling. NGF has a high affinity for binding to tyrosine kinase receptors (TrkA) [Citation34]. Atrophy and decreased TrkA expression after axotomy can be reversed by the addition of exogenous NGF, which also upregulates neurofilament expression and improves growth of dorsal root ganglion axons in the peripheral nerve system [Citation35,Citation36]. In this study, to enhance the effect of the NGF, we used collagen in the construction of conduits and fibrin gel for NGF loading. Yet, there are no reports utilizing NGF inside PCL/collagen/NBG nanofibrous conduits for Sciatic nerve tissue engineering. The useful effects of NGF viewed in the present study are in line with several previous studies.
Improvement of sensory and motor recovery as well as motor nerve conduction velocity and evoked muscle action potential have been observed after administration of NGF [Citation33]. In the electrophysological study, measurements of EMAP and latency both showed an increase as a function of the trial period, which suggested that the transected nerve had undergone enough regeneration. From the results of walking tract analysis, the autograft group showed a higher SFI score than the three experimental groups in the first month after surgery, but After 3 months, SFI result exhibited Conduit + NGF and conduit group achieved significant functional recovery as autografts.
To assess the sensory recovery, behavioral test such as hot plate test is used [Citation37]. In autograft, Conduit + NGF and conduit groups, one Month after surgical transection, the withdrawal reflex latency dropped to 12 s and decreased until the third month. NGF treated rats had significantly better responsiveness than the autograft group but only the end of the fourth week and no at the end of the eighth and twelfth weekends. This improved recovery of a heat response in the NGF group as observed after 4 weeks could be attributable to enhanced regeneration of the accurate population of sensory axons. In fact, administration of NGF promoted nerve regeneration within conduits at an early stage, the promoting effect would not last after 1 month [Citation32]. Alternatively, cotreatment with NGF could possibly induce a greater heat sensitivity in the regenerated axons [Citation20]. Furthermore, previous studies have shown that NGF is a strong stimulator of degranulation of mast cells, releasing 5-hydroxytryptamine and histamine, which are involved in hypersensitivity reactions. It is likely that circulating NGF induces a release of bioactive mediators from mast cells located adjacent to the peripheral cutaneous sensory nerve endings, thereby facilitating nervous activity, e.g. by increasing substance P levels [Citation38].
Twelve weeks post-implantation, successful regeneration of nerves across the gap happened in all conduits. The structure of fabricated conduits was stable to support regeneration for many months until the nerve is repaired. Macroscopic observations showed neither deformation nor swelling or breakage of the conduits (). Moreover, neither conduit adhesions nor inflammatory responses were observed, indicating ideal biocompatibility with the guidance conduits. Also, the MTT assay and DAPI staining showed a good cell survival rate, and there was not any evidence of conduit toxicity.
As well, a large number of new capillaries were observed in groups containing conduits. This could be attributable to the presence of nano bioglass particles in the structure of the conduits and their proangiogenic potential.This is in good agreement with previous studies which reported low concentrations of Bioglass 45S5 (0.02–0.2%w/v) have increased secretion of bFGF and VEGF, as well as the capacity to induce the formation of endothelial cell tubules and to stimulate neovascularization [Citation39]. Also, Leu et al. reported that BG has a biphasic nature in that it possesses proangiogenic potential over a limited range of concentrations and greater osteogenic potential at higher concentrations [Citation25]. Therefore, their data confirmed our result in this regards. Furthermore, Silicate-based Bioglass may also be an angiogenic stimulator via up-regulating expression of receptors of angiogenic growth factors KDR (VEGF receptor), FGFR1 (bFGF receptor) and ACVRL1 (TGFb receptor), and increased their downstream products, such as eNOS gene expression and NO synthesis. Also, bioceramics with higher Si concentrations had an obvious anti-apoptosis effect on endothelial cells [Citation40]. So, this study confirmed that a biomaterial-based strategy can be effective to induce angiogenesis.
Histological assessment showed that the numerous bundles of regenerated sciatic nerve fibers were found in the distal sections of the regenerated tissues in group Conduit + NGF group were similar to autograft group, but significantly higher than those of group's conduit alone and negative control (). It could be due to the integration of NGF in the structure of conduits. Also, less vacuole formation was observed in a group treated with NGF. In H&E staining, greater vacuole formation was scored as negative and less vacuolization was scored as positive. It should be pointed out that, in the nerve defect group, no remarkable axon regeneration has been observed. Moreover, dark spots around the axon sheets are Schwann cells. These cells with elongated nuclei ensheath axons are scattered in all parts of a peripheral nervous system. Histological examination with H&E staining showed medium repair in the group of rats that received conduits alone, whereas notable nerve regeneration and apparent reconnection of the transected nerve was seen in rats that received NGF + conduit implant which was consistent with the images of LFB staining. In an immunocytochemical analysis, we could observe stronger NF-200 positive expression in NGF treated group than that of group conduit and it was similar to autograft group. Also, Assessment of angiogenesis rate by CD31 immunoreactivity, displayed blood vessels formation in the implanted group with conduits.
Conclusion
We have demonstrated that PCL/Collagen/NBG nerve conduits loaded with NGF and fabricated by electrospinning technology have the potential to promote sciatic nerve regeneration. The functional assessments and morphometric measurements revealed that the single dose of NGF administration could enhance the recovery rate of the sciatic nerve damaged. A thorough study of the effects of NGF control release on the axons growth and sciatic nerve regeneration will be followed in the future and will compare the findings of these two methods of NGF administration.
Acknowledgements
We thank our colleagues from Laboratory Animal Sciences Center of Baqiyatallah University of Medical Scinces, who provided animals and animal model making that greatly assisted the research.
Disclosure statement
The authors report no conflicts of interest. The authors alone are responsible for the content and writing of this article.
Additional information
Funding
References
- Kou Y, Peng J, Wu Z, et al. Small gap sleeve bridging can improve the accuracy of peripheral nerve selective regeneration. Artif Cells Nanomed Biotechnol. 2013;41:402–407.
- Lee JY, Bashur CA, Milroy CA, et al. Nerve growth factor-immobilized electrically conducting fibrous scaffolds for potential use in neural engineering applications. IEEE Trans Nanobiosci. 2012;11:15–21.
- Mohamadi F, Ebrahimi‐Barough S, Reza Nourani M, et al. Electrospun nerve guide scaffold of poly (ε‐caprolactone)/collagen/nanobioglass: an in vitro study in peripheral nerve tissue engineering. J Biomed Mater Res A. 2017;105:1960–1972.
- Yu W, Zhao W, Zhu C, et al. Sciatic nerve regeneration in rats by a promising electrospun collagen/poly (ε-caprolactone) nerve conduit with tailored degradation rate. BMC Neurosci. 2011;12:1.
- Ebrahimi M, Ai J, Biazar E, et al. In vivo assessment of a nanofibrous silk tube as nerve guide for sciatic nerve regeneration. Artif Cells Nanomed Biotechnol. 2018;16:1–8.
- Cirillo V, Clements BA, Guarino V, et al. A comparison of the performance of mono-and bi-component electrospun conduits in a rat sciatic model. Biomaterials. 2014;35:8970–8982.
- Ezra M, Bushman J, Shreiber D, et al. Enhanced femoral nerve regeneration after tubulization with a tyrosine-derived polycarbonate terpolymer: effects of protein adsorption and independence of conduit porosity. Tissue Eng A. 2013;20:518–528.
- Cao H, Liu T, Chew SY. The application of nanofibrous scaffolds in neural tissue engineering. Adv Drug Deliv Rev. 2009;61:1055–1064.
- Mohamadi F, Ebrahimi‐Barough S, Nourani MR, et al. Electrospun Nerve Guide Scaffold of Poly (ε‐caprolactone)/collagen/nano‐bioglass: an in‐vitro study in peripheral nerve tissue engineering. J Biomed Mater Res A. 2017;105:1960–1972.
- Koudehi MF, Fooladi AAI, Mansoori K, et al. Preparation and evaluation of novel nano-bioglass/gelatin conduit for peripheral nerve regeneration. J Mater Sci Mater Med. 2014;25:363–373.
- Gorustovich AA, Roether JA, Boccaccini AR. Effect of bioactive glasses on angiogenesis: a review of in vitro and in vivo evidences. Tissue Eng Part B Rev. 2009;16:199–207.
- Liu JJ, Wang CY, Wang JG, et al. Peripheral nerve regeneration using composite poly(lactic acid-caprolactone)/nerve growth factor conduits prepared by coaxial electrospinning . J Biomed Mater Res A. 2011;96:13–20.
- Zeng W, Rong M, Hu X, et al. Incorporation of chitosan microspheres into collagen-chitosan scaffolds for the controlled release of nerve growth factor. PLoS One. 2014;9:e101300.
- Fine EG, Decosterd I, Papaloïzos M, et al. GDNF and NGF released by synthetic guidance channels support sciatic nerve regeneration across a long gap. Eur J Neurosci. 2002;15:589–601.
- Chen P-R, Chen M-H, Sun J-S, et al. Biocompatibility of NGF-grafted GTG membranes for peripheral nerve repair using cultured Schwann cells. Biomaterials. 2004;25:5667–5673.
- Tian L, Prabhakaran MP, Hu J, et al. Coaxial electrospun poly (lactic acid)/silk fibroin nanofibers incorporated with nerve growth factor support the differentiation of neuronal stem cells. RSC Adv. 2015;5:49838–49848.
- Quarta S, Baeumer BE, Scherbakov N, et al. Peripheral nerve regeneration and NGF-dependent neurite outgrowth of adult sensory neurons converge on STAT3 phosphorylation downstream of neuropoietic cytokine receptor gp130. J Neurosci. 2014;34:13222–13233.
- Mohamadi F, Ebrahimi-Barough S, Nourani MR, et al. Enhanced sciatic nerve regeneration by human endometrial stem cells in an electrospun poly (ε-caprolactone)/collagen/NBG nerve conduit in rat. Artif Cells Nanomed Biotechnol. 2017 [cited 2017 Nov 8]; [13 p.]. DOI:10.1080/21691401.2017.1391823
- Varejão AS, Meek MF, Ferreira AJ, et al. Functional evaluation of peripheral nerve regeneration in the rat: walking track analysis. J Neurosci Methods. 2001;108:1–9.
- Varejão AS, Cabrita AM, Geuna S, et al. Functional assessment of sciatic nerve recovery: biodegradable poly (DLLA‐ϵ‐CL) nerve guide filled with fresh skeletal muscle. Microsurgery. 2003;23:346–353.
- Cui L, Jiang J, Wei L, et al. Transplantation of embryonic stem cells improves nerve repair and functional recovery after severe sciatic nerve axotomy in rats. Stem Cells. 2008;26:1356–1365.
- Biazar E, Heidari Keshel S. Development of chitosan-crosslinked nanofibrous PHBV guide for repair of nerve defects. Artif Cells Nanomed Biotechnol. 2014;42:385–391.
- Ghasemi-Mobarakeh L, Prabhakaran MP, Morshed M, et al. Electrospun poly (ɛ-caprolactone)/gelatin nanofibrous scaffolds for nerve tissue engineering. Biomaterials. 2008;29:4532–4539.
- Cipitria A, Skelton A, Dargaville T, et al. Design, fabrication and characterization of PCL electrospun scaffolds—a review. J Mater Chem. 2011;21:9419–9453.
- Leu A, Leach JK. Proangiogenic potential of a collagen/bioactive glass substrate. Pharm Res. 2008;25:1222–1229.
- Bunting S, Di Silvio L, Deb S, et al. Bioresorbable glass fibres facilitate peripheral nerve regeneration. J Hand Surg. 2005;30:242–247.
- Yan Q, Yin Y, Li B. Use new PLGL-RGD-NGF nerve conduits for promoting peripheral nerve regeneration. Biomed Eng Online. 2012;11:36.
- Seniuk NA. Neurotrophic factors: role in peripheral neuron survival and axonal repair. J Reconstr Microsurg. 1992;8:399–404.
- Rich KM, Alexander TD, Pryor JC, et al. Nerve growth factor enhances regeneration through silicone chambers. Exp Neurol. 1989;105:162–170.
- Sun W, Lin H, Chen B, et al. Promotion of peripheral nerve growth by collagen scaffolds loaded with collagen-targeting human nerve growth factor-beta. J Biomed Mater Res A. 2007;83:1054–1061.
- Jönhagen ME, Nordberg A, Amberla K, et al. Intracerebroventricular infusion of nerve growth factor in three patients with Alzheimer’s disease. Dement Geriatr Cogn Disord. 1998;9:246–257.
- Xu X, Yee W-C, Hwang PY, et al. Peripheral nerve regeneration with sustained release of poly (phosphoester) microencapsulated nerve growth factor within nerve guide conduits. Biomaterials. 2003;24:2405–2412.
- Jubran M, Widenfalk J. Repair of peripheral nerve transections with fibrin sealant containing neurotrophic factors. Exp Neurol. 2003;181:204–212.
- Belkas JS, Shoichet MS, Midha R. Axonal guidance channels in peripheral nerve regeneration. Oper Tech Orthopaed. 2004;14:190–198.
- Verge V, Merlio J, Grondin J, et al. Colocalization of NGF binding sites, trk mRNA, and low-affinity NGF receptor mRNA in primary sensory neurons: responses to injury and infusion of NGF. J Neurosci. 1992;12:4011–4022.
- Verge V, Tetzlaff W, Bisby M, et al. Influence of nerve growth factor on neurofilament gene expression in mature primary sensory neurons. J Neurosci. 1990;10:2018–2025.
- Wong K-H, Kanagasabapathy G, Bakar R, et al. Restoration of sensory dysfunction following peripheral nerve injury by the polysaccharide from culinary and medicinal mushroom, Hericium erinaceus (Bull.: Fr.) Pers. through its neuroregenerative action. Food Sci Technol (Campinas). 2015;35:712–721.
- Della Seta D, de Acetis L, Aloe L, et al. NGF effects on hot plate behaviors in mice. Pharmacol Biochem Behav. 1994;49:701–705.
- Day RM. Bioactive glass stimulates the secretion of angiogenic growth factors and angiogenesis in vitro. Tissue Eng. 2005;11:768–777.
- Zhai W, Lu H, Wu C, et al. Stimulatory effects of the ionic products from Ca-Mg-Si bioceramics on both osteogenesis and angiogenesis in vitro. Acta Biomater. 2013;9:8004–8014.