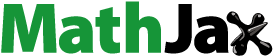
Abstract
The motive of study was to develop biotinylated chitosan (BI-CHI) decorated docetaxel (DTX) loaded nanocochleates (BI-CHI-DTX-NC) to achieve controlled drug release, improve bioavailability, targeted delivery and enhanced anticancer potency with the reduced systemic toxicity of DTX. The development involved the loading of DTX to nanocochleates (DTX-NC) through conversion of dimyristoylphosphatidylglycerol-sodium (DMPG-Na) and cholesterol bearing liposome on addition of calcium ions, followed by encapsulated DTX-NC with BI-CHI (BI-CHI-DTX- NC) and compared with DTX and DTX-NC. The release of DTX indicated strong pH dependence and implies strong hydrogen-bonding between nanocochleates and DTX. Formulated BI-CHI-DTX-NC demonstrated higher in-vitro anticancer activity in biotin over expressed human breast cancer MCF-7 cells. The targeting effect for the BI-CHI-DTX-NC was also demonstrated. The concentration of the drug needed for growth inhibition of 50% of cells in a designed time period (GI50) was 1.8 μg/ml for free DTX while it was decreased by 33.34% for the DTX-NC (1.2 μg/ml). Furthermore, the GI50 value of BI-CHI-DTX-NC was 0.2 μg/ml, i.e. an 88.89% decrease was observed as compared to DTX solution. Moreover, bioavailability of DTX from BI-CHI-DTX-NC was increased by 10-folds with longer circulation time and slower plasma elimination with low tissue distribution as compared to DTX solution. The results indicate that the BI-CHI-DTX- NC has the potential to be applied for targeting anticancer drug delivery.
Graphical Abstract
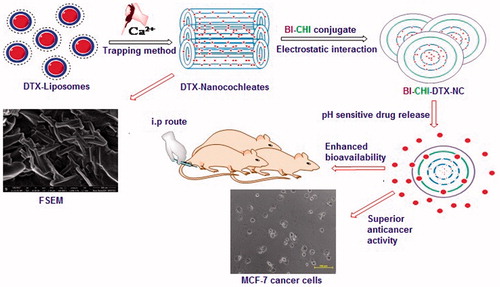
Introduction
Docetaxel (DTX), extensively employed for the antitumor therapy as well as over the advanced stages of metastatic breast cancer, prostate cancer and ovarian cancer cases [Citation1]. The current marketed formulation Taxotere® is two-vial formulation in which one vial contains DTX-Tween 80 and 13% w/v ethanol on the other. There is another single vial formulation with dosage strength of 20 and 80 mg/ml. In these formulations, it is essential to dilute the formulations prior to administration and should be used within 4 h due to instability of Taxotere® under dilution. The dilution is essential in order to limit the concentration of tween 80 under 1%, above which produce haemolysis, acute hypersensitivity and systemic retention [Citation2].
In order to minimise the adverse effects of DTX, efforts have been carried out as liposomes [Citation3,Citation4], protein conjugates [Citation5], nanoparticles [Citation6,Citation7], ligand-grafted nanoparticles [Citation8], micelles [Citation9], SEDDS [Citation10], fullerenes [Citation11], prodrugs [Citation12] and even newer formulations like metal organic frameworks [Citation13]. These formulations were promising at some extent but were observed to show limitations like poor encapsulation, intricate methods of preparation, low physiochemical stability, haemocompatibility issue as well as affinity to reticuloendothelial system uptake [Citation14].
Nanocochleates (NCs) are the liposomal precipitates whereby divalent cation destabilizes the phospholipid to form a roll of stacked sheets ending in a robust cockle-shaped structure [Citation15]. The lipid based NCs system offers greater potential for the development of noble pharmaceuticals due to its perks such as biocompatibility, ease and safety of production, lowered adverse effects and increased efficacy. The use of chitosan (CHI) in place of divalent cation has shown promising results in increased stability even under oral administration keeping the bioavailability in check and bettered [Citation16]. NCs were also used to preserve the antioxidant activity of thyme essential oils and the results were encouraging [Citation17].
The exploration of active targeting techniques using ligands is getting popular as well as efficient for quite of a time now. The selectivity of ligands to cancerous cells averts the toxicity problem to healthy tissues and ensures maximum efficacy of the therapy. Biotin (BI) has been used as a targeting moiety over drug-loaded carriers like liposomes [Citation18], dendrimers [Citation19] and nanoparticles [Citation20,Citation21] designed to increase cellular uptake in tumour cells. BI receptors are over expressed in the majority of cancer cells like breast cancer, prostate cancer, pancreatic cancer and ovarian cancer [Citation19]. BI is water soluble vitamin (vitamin H) having essential roles involving cell growth, signal transduction and many other cellular functions. BI is internalized into the cells through binding to the sodium-dependent multivitamin transporter (SMVT) present over cancer cell surfaces [Citation22,Citation23]. As cancer cells are under rapid proliferation, the transporters are highly expressed which desires more BI for rapid cell divisions. The use of BI as targeting moiety and NCs as carrier can ensures the selectivity and deliverability as well as stability of the formulation.
CHI is a natural biodegradable polymer which increases the biocompatibility of the matrix for the controlled release of therapeutics [Citation24]. The CHI is favourable for being used as a polymer in pharmaceutical formulation due to its proximal amino group available for ligand conjugation, positive charge on the surface attracted to negatively charged nanoparticles electrostatically as well as the tendency to degrade under lysosomal enzymes at acidic pH of tumor microenvironment makes it suitable for the formulation. Some of the works involving chitosan in nanoparticles [Citation25–27] signify the efficiency of the polymer being used in nanomedicine and the future it beholds over scientific advancement. CHI can be covalently conjugated with the acid group of BI [Citation28] and the conjugated BI-CHI has two amino groups under resonance in BI end and hydroxyl groups in CHI end thereby localizing charges on the terminal end which facilitate strong electrostatic interaction between biomolecules and NCs which acts as novel drug formulation.
In the present study, we developed biotinylated chitosan (BI-CHI)-decorated docetaxel (DTX)-loaded nanocochleates (BI-CHI-DTX-NC) to achieve controlled drug release and active targeted delivery with enhanced anticancer potency and reduced systemic toxicity of DTX. The development involved the loading of DTX to nanocochleates (DTX-NC) through conversion of dimyristoylphosphatidylglycerol-sodium (DMPG-Na) and cholesterol bearing liposome into the NCs structure on addition of calcium ions, negatively charged DMPG-Na selected as the phospholipid components because it has been reported to yield NCs. Cholesterol to form complexes with drug as well as to stabilize the phospholipid membranes, followed by encapsulation of DTX-NC with BI-CHI conjugation (BI-CHI-DTX-NC) that shows controlled release with targeted delivery of DTX. The developed BI-CHI-DTX-NC was compared with DTX-NC and DTX in terms of in-vitro and in-vivo evaluations.
Methods
Materials
Docetaxel anhydrous and Paclitaxel (Internal Standard) were purchased from Sigma-Aldrich Chemical Private Limited, Bangalore, India. Chitosan (low molecular weight 179.17 kDa), Biotin and dialysis bag (MW cut off 12000) were obtained from Sigma-Aldrich Chemical Private Limited, India. DMPG-Na was gifted from Lipoid GmbH Ludwigshafen, Germany. Cholesterol was obtained from Research-Lab Fine Chem Industries Ltd, Mumbai, India. Ethanol, chloroform, EDTA (ethylenediaminetetraacetic acid) and calcium chloride were of analytical grade. Anhydrous dimethylsulfoxide (DMSO) and N,N′-dicyclohexyl carbodiimide were purchased from Loba-Chem Pvt. Ltd. Methanol (HPLC grade) and acetic acid were purchased from Merck Chemicals, Mumbai, India. ELISA plates of SRB assay were read on the Sunrise model of Tecan, Austria. All other solvents used for the study were of analytical grade.
Synthesis of BI-CHI conjugates
The BI-CHI conjugate was synthesized according to a method by Balan et al with some modifications [Citation28]. BI (50 mg) solution (2 ml DMSO) was treated with DCC (67.3 gm) and NHS (31.3 gm) for 30 min at 4 °C in order to activate BI. The BI-activated solution was added dropwise in to 25 ml solution of CHI (1% in 0.1 M acetic acid) under slowly stirring at room temperature and kept for 24 h under stirring condition. The BI-CHI conjugates was precipitated by adding acetone, purified by dialysis for 72 h in distilled water, lyophilized and stored at 4 °C.
Characterization of BI-CHI conjugates
Fourier transform infrared spectroscopy (FTIR) of BI, CHI and BI-CHI were obtained by using Brucker Vertex-70/80 FTIR (Tokyo, Japan). 1 H NMR spectrum was measured on a Bruker ARX-400 spectrometer operating at 400 MHZ at 25 °C. BI was dissolved in deuterated DMSO and BI-CHI was dissolved in deuterated water (containing 1% deuterated trifluoroacetic acid) for acquisition of NMR spectra. UV-VIS spectra were acquired using spectrophotometer (V630, JASCO, Japan) as a qualitative proof of BI conjugation with CHI. The degree of BI conjugation was determined by UV–VIS spectrophotometer. BI solutions of known concentration were prepared in DMSO and their respective UV absorbance was recorded to plot its calibration curve. To determine the concentration of BI in conjugate, sample (10 mg) was dissolved in DMSO, absorbance was recorded at 205 nm and BI concentration was calculated. Degree of conjugation of BI on a weight basis was calculated by using the following equation: Conjugation of BI (wt%) = Weight of BI in conjugate/Total weight of conjugate ×100. Powder X-ray diffraction (PXRD) was conducted using X-ray diffractometer (PW 1729; Philips, Almelo, Netherlands). Thermal properties were studied using differential scanning calorimetry (DSC 821e; Mettler-Toledo, Greifensee, Switzerland).
Preparation of DTX-loaded nanocochleates (DTX-NC)
Preparation of DTX-loaded liposomes
DTX-loaded liposomes were prepared by ethanol injection method [Citation29] with some modifications. DMPG-Na (24 mg) and cholesterol (10 mg) were dissolved in 5 ml mixture of chloroform and ethanol (3:2v/v) and warmed gently up to the phase transition temperature of DMPG-Na. DTX (6 mg) was dissolved in 0.5 ml of ethanol and added to the lipid cholesterol solution. The solution was rapidly injected into 10 ml of demineralised water (pH 7.0) under stirring at 800 rpm until complete evaporation of ethanol and chloroform. Final volume was adjusted with demineralised water and the obtained DTX-loaded liposomes were filtered through 0.45 μm membrane filter.
Preparation of DTX-loaded nanocochleates (DTX-NC)
DTX-loaded nanocochleates (DTX-NC) were prepared by trapping method [Citation30]. CaCl2 (20 μl) was added dropwise in to DTX-loaded liposomal formulation under vortex. The liposomal phase immediately turned turbid because of NCs formation. Precipitated DTX-NCs were stored at 2– 8 °C.
Preparation of BI-CHI decorated DTX-NC (BI-CHI-DTX-NC)
BI-CHI lyophilized powder (10 mg) was dissolved in DMSO (2 ml) and sonicated for 15 min to dissolve BI-CHI. The resulting solution was added into DTX-NC suspension under stirring at room temperature for 6 h. After stirring, the BI-CHI-DTX-NC carrier were collected as suspension and lyophilized. The schematic illustration of the fabrication of BI-CHI-DTX-NC is presented in .
Evaluation of DTX-NC and BI-CHI-DTX-NC
The particle size of DTX-NC and BI-CHI-DTX-NC was determined by Dynamic Light Scattering technique (Brookhaven Instruments Corp. 90 plus particle sizing software Ver.5.34). The zeta potential was measured with laser Doppler electrophoretic mobility measurement using HORIBA Nanoparticle analyzer (HORIBA SZ for Windows, Version 1.9).
The encapsulation efficiency was determined by pellets separation technique [Citation31]. 1 ml of NCs was aliquoted into polypropylene centrifuge tube. The tube was centrifuged at 20000 rpm for 15 min at 4 °C, the supernatant and pellets were separated. 100 μl of pH 9.5 EDTA was added to the NCs pellet to allow the opening of the NCs into liposomes and the release of DTX. One milliliter of ethanol was added followed by vortexing the solution for 2 min. The resulting solution was clear which was suitably diluted with the demineralized water of pH 7 and the absorbance was determined at 231 nm. The free DTX concentration in supernatant was also measured and encapsulation efficiency was calculated using following equation:
(1)
(1)
The shape and surface morphology of the NCs were observed under Field Emission Scanning Electron Microscopy using Nova NanoSEM 450 (Bruker) under FESEM software Espirit 1.9. Also, the NCs were visualized using field transmission electron microscope (TEM; Philips CM-200, Eindhoven, Netherlands). The Fourier transform infrared spectroscopy (FT-IR) spectra’s were recorded using an FTIR spectrometery (FTIR-8400; Shimadzu Corporation, Kyoto, Japan). The powder XRD pattern was determined using a Bruker AXS Advance D-8 scanner.
In-vitro drug release study
The in-vitro release of DTX from DTX-NC and BI-CHI-DTX-NC was carried out in phosphate buffer saline (PBS, pH 7.4 and pH 5.3) using dialysis bag diffusion technique. Formulation equivalent to 1 mg of DTX was introduced into a dialysis bag (cellulose membrane, molecular weight cut off 12, 0000 Da), hermetically sealed and immersed into 100 ml of release medium. The entire system was kept at 37 ± 0.5 °C with continuous magnetic stirring at 100 rpm/min. At selected time intervals, sample was removed and the amount of DTX was quantified using UV-Vis absorption spectrophotometer.
Pharmacokinetic study in rats
Experimental design and sample collection
Healthy female rats weighing around 200 grams and 8 weeks old were procured from National Institute of Biosciences, Pune, India. Animals were acclimatized for one week prior to the initiation of treatment. During this acclimatization period, the health status of the animals was monitored daily. The rats were fasted yet provided free access of water overnight before the day of the experiment. The protocol was reviewed and approved by the Institutional Animal Ethics Committee of Poona College of Pharmacy (Pune, India). Rats were randomly divided into three groups (n = 4 in each group). First group received DTX at the dose of 5 mg/kg (suspended in 30% PEG 400) by intraperitoneal (i.p.) route. Second group received DTX-NC at the dose of 5 mg/kg by i.p. route. Third group received BI-CHI-DTX-NC at the dose of 5 mg/kg by i.p. Blood (200 µl) was sampled by capillary tube puncture from the retro orbital sinus veins in EDTA-coated tubes (BD Vacutainer (R) K2 EDTA) at the predetermined time intervals (0.5, 1, 2, 4, 6, 12, 24, 48 and 72 h) after administration. Samples were centrifuged at 10000 rpm for 15 min and the plasma obtained was stored at –20 °C for later analysis.
Bioanalysis of DTX
The plasma DTX concentrations were determined by HPLC analysis according to the method reported by Chun Chu et al [Citation32]. After re-equilibration to room temperature, plasma samples (200 μl) were transferred to 2-ml Eppendorf centrifuge tube, to which 20 μl of internal standard (Paclitaxel 100 ng/ml in methanol) was added and homogenized by vortex mixing for 30 s twice to ensure uniform distribution. 2 ml of ethyl acetate was added as extraction solvent to the mixture. The mixture was sonicated for 3 min and vortexed for 5 min followed by centrifugation at 10,000 rpm for 10 min at ambient temperature. The supernatant 200 μL was transferred into a 5-ml glass tube and evaporated to dryness for 45 min at room temperature. The residue was reconstituted in 200 μL of methanol and 10 μL aliquot was used for HPLC analysis.
The HPLC system specifications were as follows: pump, PU-2080 plus (JASCO, Japan); injector; column, Peerless C18, 250 × 4.6 mm, 5 μm (Chromatopak analytical instrumentation India); detector, UV/visible (UV- 2075 plus; JASCO). Data acquisition and analysis were carried out using Borwin/HSS 2000 software (LG 1580–04; JASCO). The mobile phase was a mixture of acetonitrile and water 60:40. The column temperature and flow rate were 35 °C and 0.8 ml/min; wavelength was 231 nm. The pharmacokinetic parameters were analysed by a non-compartmental model with the aid of the programme WINNON-LIN 4 (Pharsight Corp., Mountain View, CA, USA. All values were expressed as their mean ± SD Difference were considered to be significant at a level of p < 0.05.
Tissue bio-distribution study
To investigate the bio-distribution of DTX and BI-CHI-DTX-NC, 6 female Wistar rats (200 g) were randomly divided into two groups (three in each). Formulations were administered by intraperitoneal route at dose 10 mg/kg. Rats were sacrificed by cervical dislocation after 4 h after administration of dose. Liver, lungs, heart and spleen were excised and weighed. The tissues were homogenized with 4 ml methanol, centrifuged and supernatant was injected into HPLC system to determine DTX concentration [Citation17].
In vitro anticancer study
In vitro anticancer activity of free DTX, DTX-NC, BI-CHI-DTX-NC and blank DTX-NC nanocochleates (BNC) were evaluated against human breast cancer MCF-7 cells using in vitro sulforhodamine B assay (SRB assay) [Citation33]. The cell lines were grown in RPMI 1640 medium containing 10% fetal bovine serum and 2 mM L-glutamine. For present screening experiment, cells were inoculated into 96 well microtitre plates in 100 µL at plating densities as shown in the study details above, depending on the doubling time of individual cell lines. After cell inoculation, the microtiter plates were incubated at 37 °C, 5% CO2, 95% air and 100% relative humidity for 24 h prior to addition of experimental drugs. Experimental drugs were initially solubilized in dimethyl sulphoxide at 100 mg/ml and diluted to 1 mg/ml using water and stored frozen prior to use. At the time of drug addition, an aliquot of frozen concentrate (1 mg/ml) was thawed and diluted to 100 μg/ml, 200 μg/ml, 400 μg/ml and 800 μg/ml with complete medium containing test article. Aliquots of 10 µl of these different drug dilutions were added to the appropriate microtitre wells already containing 90 µl of medium, resulting in the required final drug concentrations i.e0.1 μg/ml, 2 μg/ml, 4 μg/ml, 8 μg/ml. After compound addition, plates were incubated at standard conditions for 48 h and assay was terminated by the addition of cold TCA. Cells were fixed in situ by the gentle addition of 50 µl of cold 30% (w/v) TCA (final concentration, 10 TCA) and incubated for 60 min at 4 °C. The supernatant was discarded; the plates were washed five times with tap water and air dried. Sulphorhodamine B (SRB) solution (50 µl) at 0.4% (w/v) in 1% acetic acid was added to each of the wells and plates were incubated for 20 min at room temperature. After staining, unbound dye was recovered and the residual dye was removed by washing five times with 1% acetic acid. The plates were air-dried. Bound stain was subsequently eluted with 10 mM trizma base and the absorbance was read on an plate reader at a wavelength of 540 nm with 690 nm reference wavelength.
Percent growth was calculated on a plate-by-plate basis for test wells relative to control wells. Percent growth was expressed as the ratio of average absorbance of the test well to the average absorbance of the control wells ×100. Using the six absorbance measurements [time zero (Tz), control growth (C) and test growth in the presence of drug at the four concentration levels (Ti)], the percentage growth was calculated at each of the drug concentration levels. Percentage growth inhibition was calculated as:
Results and discussion
Synthesis of BI-CHI conjugate
Since BI receptors are over-expressed on cancer cells, we have intended to decorated NC with BI-CHI conjugate. This involved the synthesis of BI-CHI conjugate followed by the encapsulation of DTX loaded nanocochlates (DTX-NC) with BI-CHI conjugate to obtain BI-CHI decorated DTX loaded nanocochlates (BI-CHI-DTX-NC) carrier. BI-CHI conjugation was prepared by ionic gelation technique [Citation30]. The chemical cross linking between BI and CHI through amide linkage stabilize the polymer and the terminal ends localise positive charge for interaction with NCs. The BI is initially activated by using DCC and NHS whereby the carboxylic end of BI is protected by reacting with NHS to form amine reactive intermediate. The intermediate further reacts with CHI to form the conjugate with formation of strong and stable amide bond () [Citation34].
Characterization of BI-CHI conjugates
Fourier transforms infrared spectroscopy
The FTIR spectrum of BI, CHI and BI-CHI conjugates were shown in . In BI, the peaks appeared in between 3600 and 3000 cm−1 were of hydroxyl (–OH) stretching and N–H stretching vibrations. The C = O stretching vibration of carboxyl group appeared at 1680 cm−1, while the peak at 1657 cm−1 assigned to the C = O bond stretching vibration of –CO–NH group. The characteristic absorption peak of phenyl ring appeared at 1482 cm−1 [Citation35]. The FTIR spectrum of CHI revealed the existence of stretching vibration of N–H (3456 cm−1), and C–H stretch vibration (2884 cm−1 and 2846 cm−1) functional groups. Peaks at ∼1664 cm−1, 1592 cm−1 and 1327 cm−1 were characteristic of amide I, II and III, respectively. The representative peaks due to –CH3 symmetrical deformation appeared at 1316 cm−1, while peaks at 1201 cm−1 and 1071 cm−1 were indicative of C–O stretching vibrations from (C–O–C) [Citation36]. The FTIR spectrum of BI-CHI conjugate showed the characteristic absorption bands of both BI and CHI. The peak due to the deformation vibration N–H amide-II of the amine group (1592 cm−1) shifted to a higher frequency at 1657 cm−1 confirmed the existence of chemical interactions between BI and CHI. Moreover, the peaks due to amide-III (1327 cm−1) and C–O stretching vibrations (1087 cm−1) of CHI were shifted to 1320 and 1067 cm−1, respectively, suggesting the possibility of a strong interaction between BI and CHI.
H1 NMR analysis
The appearance of characteristic peaks in the 1 H NMR spectrum of BI-CHI conjugation confirm that BI is successfully incorporated with CHI. As shown in the 1 H NMR spectrum of BI (). The signals at 11.9, 6.5, 4.3, 4.2, 3.1, 2.8, 2.5, 2.2 and 1.5 ppm were corresponding to the BI protons coded as a, d, e, g, h, b, i, f and c respectively. The coupling of BI with CHI leads to the overlapping of some sympathetic vibration peaks due to the influence of solvent and the interaction between BI and CHI. The characteristics peaks of BI at 6.5 and 4.2 ppm can still be observed. The conversion of triplet signal of BI at 6.56 is converted to singlet in case of BI-CHI which suggested that the terminal amine group of BI was hidden whereas the formation of amide in between CHI and BI showed the singlet signal at 6.6 ppm. Most of the hydrogen peaks of BI below 4 ppm remain invisible in the BI-CHI which suggested that the presence of excess amount of CHI shades over the presence of BI. The characteristic peaks of CHI at 3.32 (H-2’/H-5’/H-6’), 1.92 (H-4’, CHI) were prominently observed in BI-CHI.
Ultraviolet spectroscopy analysis
Preliminary determination were acquired using UV-VIS spectra in which CHI doses not showed any absorbance peaks from 200–400 nm whereas BI showed the characteristic peak at 205.2 nm. The UV–VIS spectrum of the BI-CHI showed blue shift of absorbance to 203.1 nm due to the interaction (data not shown). The peak of BI at 205.2 nm shifted to 202.1 nm after interaction with CHI which is generally believed due to the ground-state electron donor–acceptor interaction between the BI and CHI. The degree of substitution of BI onto CHI was ∼5.9% as determined from UV.
Powder X-ray diffraction spectroscopy
The powder XRD analysis showed that the conjugates are more likely to form amorphous rather than replicating the crystalline structure (). BI showed intense peaks at 2ϴ 17.6, 18.8, 20.7, 21.3, 22.8, 34.5 and 42 indicating the crystal nature of BI. Only a hump peak was observed at 2Φ 19.8 for CHI indicating the amorphous nature of CHI. BI-CHI conjugate showed low intensity peaks of BI at 22.2°, 34.5° and 42° suggesting that the partly loss of crystalline character.
Differential scanning calorimetry
DSC thermograms of CHI and BI exhibited sharp endothermic peaks at 86 °C and 232 °C respectively, which suggests the crystalline nature. However, the both BI and CHI peaks were disappeared in the BI-CHI conjugate with appearance of new peak at 219.7 °C indicating partly crystalline nature ().
Preparation DTX-loaded nanocochleates
DTX loaded liposomes
In the present study, we selected DMPG-Na as a lipid for liposomes formation (). An anionic saturated phospholipid with two 14 C atoms chains is the major component of membranes of gram-positive bacteria [Citation37]. DMPG can interact with positively charged polymers through strong electrostatic interaction [Citation38]. The Ca2+ ion can destabilize the head of the phospholipids resulting in rolling of the lipids to create water free interior and thus forming a nanocochlear shaped structure. DTX, a lipophilic bioactive, solubilise into the lipid bilayer [Citation39]. The DTX-loaded liposomes showed particle size, encapsulation efficiency and zeta potential of 172 ± 5 nm, 76.19 ± 3.62% and −57.3 ± 3.3 mV, respectively. The negative zeta potential attributed to the anionic nature of the employed lipids.
DTX-loaded nanocochleates (DTX-NC)
The addition of calcium ions to DTX-liposomes induces fusion of lipid membrane and the formation of planar sheets, which eventually coil around an initial point of folding to form ribbon shape nanocochleates () [Citation15]. Divalent cations Ca2+, Mg2+, Ba2+ and Zn2+ can be used for preparing nanocochleates [Citation40]. It has been reported that Ca2+ forms a more tightly packed, highly ordered and less hydrated structure than does Mg2+ with phospholipids. Also it is required in much lower concentration than Mg2+ [Citation41]. Additionally, it is well documented that Ca2+ plays a vital role in natural membrane fusion phenomena while other cations listed above are ineffective in most such systems. Hence, it is most compatible with the body. Thus, calcium is the most suitable divalent cation for preparing nanocochleates and hence used in the present work. DTX-NC showed particle size of 185 ± 4 nm with encapsulation efficiency of 79.75 ± 3.82% and zeta potential of −51.4 ± 2.6 mV. The increased in particle size due to free drug encapsulation. The decrease in the zeta potential may be due to interaction between calcium ion and lipid resulting partial neutralization leading to less availability of anionic lipid on the surface.
Preparation of BI-CHI-decorated DTX-NC (BI-CHI-DTX-NC)
BI-CHI has amino groups which facilitate strong electrostatic interaction with negative charged DOX-NC (). BI-CHI-DTX-NC showed the particle size, encapsulation efficiency and zeta potential of 379 ± 4 nm (), 78.12 ± 2.72% and −51.4 ± 2.4 mV (). The increased in particle size and zeta potential as compared to DTX-NC which may be due to the decoration of BI-CHI conjugate onto the DTX-NC structure and availability of BI on the surface of DTX-NC. Surface morphology revealed cylindrical and cochlear structure with a bit of aggregation (). The rod shaped structures appears to show their tendency to remain in cluster.
Evaluation of DTX-NC and BI-CHI-DTX-NC
Fourier transform-infrared spectroscopy
The FTIR spectra of DTX, blank nanocochleates (BNC), DTX-NC and BI-CHI-DTX-NC are shown in . The DTX showed intense peaks at 3606 cm−1, 3479 cm−1, 3338 cm−1 and 3264 cm−1 (–NH and –OH stretch), 2988 cm−1 and 2898 cm−1 (–CH stretch), 2712 cm−1 (carboxylic group OH stretch), 1817 cm−1, 1744 cm−1, 1708 cm−1 (C = O ketone stretch), 1524 cm−1 and 1368 cm−1 (benzene ring twin peaks) and 1248 cm−1 (C–O stretch). The BNC showed characteristic peaks of lipid and cholesterol which were observed at 3448 cm−1, 3253 cm−1 (-NH and –OH stretch), 1734 cm−1 (C = O), 1465 cm−1(CH2–CH3 stretch), 1255 cm−1(C–O stretch) and 1159.97 cm−1 (P = O phosphine oxide stretch). DTX peaks were appeared in DTX-NC at 3399 cm−1, 3335 cm−1 (-NH and –OH stretch), 2948 cm−1, 2948 cm−1 (–CH stretch), 2704 cm−1 (carboxylic acid OH stretch), 1737 cm−1, 1666 cm−1 (C = O ketone), 1496 cm−1, 1421 cm−1 (benzene ring peaks) and 1281 cm−1 (C–O stretch). These observations confirm the loading of DTX to NC. Also, BI-CHI-DTX-NC showed characteristic peaks of DTX at 3273 cm−1 (–NH stretch), 1739 cm−1 (ketone stretch) and BI-CHI conjugate peaks at 1626 cm−1 (–C = O amide I), 1576 cm−1 (N–H amide II), 1378 cm−1 (C–H of CHI), 1256 cm−1 (C–O–H of CHI) and 1086 cm−1 (C–O–C of CHI) indicating decoration of DTX-NC by BI-CHI.
Powder X-ray diffraction spectroscopy
BI-CHI-DTX-NC form amorphous rather than crystal structure as observed from powder X-ray diffraction pattern. DTX showed intense peaks at 2Φ 19.7°, 23.4°, 29.5°, 31.8°, 34.3°, 42°and 44° indicating the crystals nature. DTX-NC showed the less intense peaks at 2Φ 19.7°, 23.4°, 31.8° and 44° indicating partial crystalline nature whereas BI-CHI-DTX-NC did not shown any intense peaks indicating amorphous nature of the product ().
In-vitro drug release study
The DTX release from DTX-NC and BI-CH-DTX-NC were investigated by the diffusion bag technique. The DTX release was studied in phosphate buffer saline solution pH 7.4 (mimicking the environment of the circulation) and 5.3 (mimicking the environment in the tumor and in the intracellular organelles) at temperature of 37°C. At pH 7.4, DTX released at a slower and controlled manner from DTX-NC and BI-CHI-DTX-NC, to the extent of ∼72% and 57% respectively after 72 h. The amount of drug release from the BI-CHI-DTX-NC is lower by ∼15% than DTX-NC ().
Figure 10. (a) Cumulative DTX release from DTX-NC and BI-CHI-DTX-NC at 37 °C in phosphate buffer solution (pH 5.3 and 7.4). The data points are average of at least three experiments. Error bars represent the range over which the value was observed. (b) The mean plasma concentration-time profiles of DTX after i.p administration of DTX solution and BI-CHI-DTX-NC in rat.
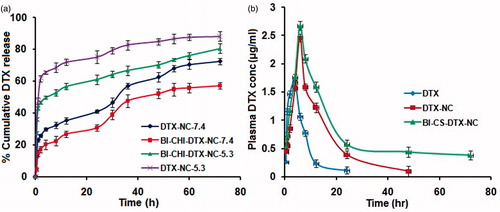
It is ascribed to the hydrogen-bonding interaction between DTX and NC which is more prominent in the neutral condition, resulting drug release in a controlled manner. On the other hand, the release pattern under acidic media indicates that the amount of DTX released was much higher than at neutral conditions. It was found that around ∼88% of the total bound DTX was released from the DTX-NC after 72 h at pH 5.3. Under acidic conditions, the amine (-NH) group of DTX get protonated resulting in the partial dissociation of hydrogen-bonding interaction, hence the amount of released DTX from NC is much higher.
Following the experiment, further work was carried out on BI-CHI-DTX-NC at pH 5.3 and it was found that a lower amount of drug was released, which is due to the BI-CHI conjugate that was used to encapsulate DTX-NC. This observation offers two possibilities that can explain the decrease in drug release in the presence of CHI. First, there is a strong intermolecular force between DTX and NC, while secondly the outer shell of biopolymeric CHI offers an additional path the drug has to travel across the macromolecular chains.
The effect of the encapsulation with BI-CHI conjugate on the drug release of DTX from DTX-NC and BI-CHI-DTX-NC can be seen, during the first 6 h, a significant amount of drug has been released from the DTX-NC at both the pH, while encapsulating with BI-CHI conjugate the release rate of DTX occurred very slowly.
This suggests that the DTX is released due to the degradation of CHI chains besides diffusion through the CHI shell. Furthermore, the electrostatic interaction between DTX-NC and CHI could also take place and responsible for slower release of drug from BI-CHI-DTX-NC. Since both DTX-NC and BI are negatively charged, the possibility of DTX-NC with BI can be ignored. Moreover, factors like hydrophobic/hydrophilic interactions and resonance effects that can facilitate DTX-NC to form a complex with BI, stable enough to affect DTX release can be completely ignored.
In addition, the release of DTX from the BI-CHI-DTX-NC and DTX-NC was also probed and it was found that after 72 h, the amount of release was greater at pH of 5.3 was ∼80% and 88% from the BI-CHI-DTX-NC and DTX-NC, respectively. This higher release of drug is due to the partial dissociation of hydrogen bonding interaction between DTX and NC as described below.
The release of DTX depends upon the hydrogen bonding interaction with NC and is a function of pH. Under acidic conditions, hydrogen bonding can be formed between − CO of DMPG and − OH of DTX. Furthermore, at low pH, the H+ in solution would compete with the hydrogen bond forming group and weaken the above outlined hydrogen bonding interaction, which may lead to a greater release of DTX. Also, at the relatively acidic pH of 5.3, degradation of CHI could be greater. At pH 7.4, the three possibilities of hydrogen bonding include (a) −CO of DMPG and − OH of DTX, (b) −CO of DMPG and the − NH of DTX and (c) −OH of cholesterol and − NH of DTX. Thus, cumulative hydrogen bonding interaction between NC and DTX is higher at pH of 7.4, resulting drug release in a controlled manner.
In vitro anticancer study
The in-vitro anticancer activity of free DTX, DTX-NC, BI-CHI-DTX-NC and blank nanocochleates (BNC) were investigated against BI over expressed human breast cancer MCF-7 cells using in-vitro SRB assay. The advantages of the NC formulation of DTX were quantified by the GI50 value which is defined as the drug concentration needed to inhibit 50% of the incubated cells growth in a designed time period [Citation42]. The results illustrated in . indicated that BI-CHI-DTX-NC demonstrated superior anticancer activity with lower GI50 (0.2 μg/ml) than that of DTX-NC (1.17 μg/ml), free DTX (1.8 μg/ml) and BNC (>4 μg/ml), respectively.
Figure 11. (a) Microphotograph of MCF-7 cancer cell. (b), (c), (d) and (e) DTX, DTX-NC, BI-CHI-DTX-NC and BNC treated MCF-7 cancer cell. (f) GI50 value of DTX, DTX-NC, BI-CHI-DTX-NC and BNC on MCF-7cancer cell. Each point represents an average ± SD (n = 3). NC nanocochleates, DTX docetaxel, DTX-NC DTX-loaded nanocochleates and BI-CHI-DTX-NC biotin-chitosan decorated DTX-loaded nanocochleates, BNC blank nanocochleates.

BI-CHI-DTX-NC showed superior activity, attributed active targeting effects to the cancer cells which may suggest that the BI-CHI-DTX-NC was endocytosed via BI and SMVT receptors-mediated mechanism. Further, improved anticancer activity of DTX-NC attributed to higher accumulation of the drug via direct interaction or phagocytosis i.e. fusion between nanocochleates and the cancer cell membrane followed by controlled release of the drug [Citation30]. Many natural membrane fusion processes involve calcium-induced perturbations of membranes containing negatively charged lipids. The hypothesis for mechanism of action of nanocochleates can also be described in a similar way, where contact of the calcium-rich, highly ordered membrane of a nanocochleates with a natural membrane causes a perturbation and reordering of the cell membrane [Citation30]. A fusion occurs between the outer layer of the nanocochleates and the cell membrane. As a result, a small amount of the encochleated material is transferred into the cytoplasm of the target cell. The nanocochleates could then break free of the cell and be available for another fusion event, either with this or another cell. Alternatively, particularly with active phagocytic cells, the nanocochleates may be taken up by endocytosis and fuse from within the endocytic vesicle. This tumour retention effect is apparently due to the nanocochleates extravasation through the porous capillary endothelium of the tumor caused by enhanced permeability and retention effect [Citation43]. The lower anticancer activity of DTX-NC as compared to BICHI-DTX-NC may be due to rapid release of DTX from formulations post membrane interaction. Also, phospholipids possess anticancer activity by apoptosis-inducing properties via the generation of reactive oxygen species or a direct perturbing effect on the cell membrane causing fluidity and leakage [Citation44]. This might be the reason for toxicity of synergizing the activity of BNC to cancer at a higher concentration.
Pharmacokinetic study
The extent of alteration in the bioavailability of DTX in case of DTX-NC and BI-CHI-DTX-NC was studied through in-vivo pharmacokinetic study in Wistar rats. Whenever a drug or formulation is administered through intravenous route, there is unavoidable tendency of the particle been trapped and retained by the RES via application of apolipoproteins. To ensure the precise calculation of the bioavailability and in order to prevent RES from interfering with the formulation, we selected intraperitoneal route to administer DTX [Citation40]. Plasma levels of DTX from DTX-NC and BI-CHI-DTX-NC were compared with free DTX (). BI-CHI-DTX-NC yielded higher DTX plasma concentrations as compared to DTX-NC and free DTX. The relevant pharmacokinetic parameters derived by noncompartmental analysis are listed in . It was observed around 1.2, 2.2, 10, 1.1 fold increase in Cmax, t1/2, AUC and MRT (p < 0.05), respectively. There was no significant difference in the mean residence time and tmax of the BI-CHI-DTX-NC, DTX-NC and free DTX.
Table 1. Mean non-compartment pharmacokinetic parameters of DTX after single i.p dose (5 mg/kg) administration of DTX and BI-CHI-DTX-NC in rat plasma.
BI-CHI-DTX-NC displayed higher Cmax and long terminal half-life. This would lead to long residence time with slow plasma elimination and consequent remarkable improvement in in-vivo anticancer effect. The enhanced DTX bioavailability is probably due to faster absorption and the slow drug release from BI-CHI-DTX-NC causing prolonged residence time in blood. Intraperitoneal administration also favours the lymphatic distribution of drugs, especially anticancer drugs which must access the lymph nodes that frequently harbour metastases [Citation45]. Similar results were reported for the hydrophobic anticancer paclitaxel where i.p. administration led to a significant improvement in its bioavailability [Citation46].
Tissue distribution study
Following bioavailability studies, the tissue distribution of the free DTX and BI-CHI-DTX-NC were examined in Wistar rats. shows that BI-CHI-DTX-NC administration resulted in significantly lower tissue DTX concentrations. Thus, a 3.3 and 2-fold low distribution was observed in heart and other organs in comparison with free DTX. BI-CHI-DTX-NC demonstrated lower tissue distribution of DTX probably owing to its lower volume of distribution as revealed in bioavailability study. The distribution in cardiac tissues is decreased which also helps to reduce the cardiotoxicity. Nevertheless, this lower tissue distribution also contributed to the remarkable decrease in vivo tissue toxicity in rats.
Conclusions
In this study, biotinylated chitosan (BI-CHI)-decorated docetaxel (DTX)-loaded nanocochleates (BI-CHI-DTX-NC) were successfully prepared. The BI-CHI-DTX-NC displayed smaller in size, smooth surfaces with cylindrical and cochlear shape, good encapsulation efficiency, pH-dependent DTX release with no sign of tissue accumulation. Further, the BI-CHI-DTX-NC demonstrated active targeting effect on biotin over expressed human breast cancer MCF-7 cells and displayed 10-fold enhanced intraperitoneal bioavailability as compared to DTX solution which may bring about reduction in dose as well as cost and increase patient compliance. Our results indicate that BI-CHI-DTX-NC may be promising vehicles for delivering DTX to cancer cells that overexpress this receptor. Further studies will focus on the in vivo antitumor efficiency and the optimization of the drug dose to obtain the most effective dose with less side effects during the treatment.
Disclosure statement
The authors declare that they have no conflicts of interest to disclose.
References
- Nabholtz J-MA, Reese DM, Lindsay M-A, et al. Docetaxel in the treatment of breast cancer: an update on recent studies. Semin Oncol. 2002;29:28–34.
- Lee SW, Yun MH, Jeong SW, et al. Development of docetaxel-loaded intravenous formulation, Nanoxel-PM™ using polymer-based delivery system. J Control Release. 2011;155:262–271.
- Wang DK, Zhang CX, Zhang WT, et al. Characteristics and pharmacokinetics of docetaxel liposome containing Tween 80 or PEG-DSPE. J Drug Deliv Sci Technol. 2008;18:253–257.
- Ma J, Zhuang H, Zhuang Z, et al. Development of docetaxel liposome surface modified with CD133 aptamers for lung cancer targeting. Artif Cells Nanomedicine Biotechnol. 2017 [Oct 30]; [8 p.]. DOI:10.1080/21691401.2017.1394874
- Esmaeili F, Dinarvand R, Ghahremani MH, et al. Docetaxel-albumin conjugates: preparation, in vitro evaluation and biodistribution studies. J Pharm Sci. 2009;98:2718–2730.
- Zeng X, Tao W, Mei L, et al. Docetaxel-loaded nanoparticles of dendrimer-like amphiphilic copolymer for cancer therapy. J Control Release. 2015;213:e119.
- Ahmad N, Alam MA, Ahmad R, et al. Preparation and characterization of surface-modified PLGA-polymeric nanoparticles used to target treatment of intestinal cancer. Artif Cells Nanomedicine Biotechnol. 2018;46:432–446.
- Mi Y, Zhao J, Feng SS. Targeted co-delivery of docetaxel, cisplatin and herceptin by vitamin E TPGS-cisplatin prodrug nanoparticles for multimodality treatment of cancer. J. Control. Release. 2013;169:185–192.
- Tong SW, Xiang B, Dong DW, et al. Enhanced antitumor efficacy and decreased toxicity by self-associated docetaxel in phospholipid-based micelles. Int J Pharm. 2012;434:413–419.
- Valicherla GR, Dave KM, Syed AA, et al. Formulation optimization of docetaxel loaded self-emulsifying drug delivery system to enhance bioavailability and anti-tumor activity. Sci Rep. 2016;6:26895.
- Thotakura N, Sharma G, Singh B, et al. Aspartic acid derivatized hydroxylated fullerenes as drug delivery vehicles for docetaxel: an explorative study. Artif Cells Nanomedicine Biotechnol. 2017 [Oct 25]; [10 p.]. DOI:10.1080/21691401.2017.1392314
- Park MH, Keum CG, Song JY, et al. A novel aqueous parenteral formulation of docetaxel using prodrugs. Int J Pharm. 2014;462:1–7.
- Rezaei M, Abbasi A, Varshochian R, et al. NanoMIL-100(Fe) containing docetaxel for breast cancer therapy. Artif Cells Nanomedicine Biotechnol. 2017 [Aug 24]; [12 p.]. DOI:10.1080/21691401.2017.1369425
- Engels FK, Mathot RAA, Verweij J. Alternative drug formulations of docetaxel: a review. Anticancer Drugs. 2007;18:95–103.
- Pawar A, Bothiraja C, Shaikh K, et al. An insight into cochleates, a potential drug delivery system. RSC Adv. 2015;5:81188–81202.
- Liu M, Zhong X, Yang Z. Chitosan functionalized nanocochleates for enhanced oral absorption of cyclosporine A. Sci Rep. 2017;7:41322.
- Asprea M, Leto I, Bergonzi MC, et al. Thyme essential oil loaded in nanocochleates: encapsulation efficiency, in vitro release study and antioxidant activity. LWT - Food Sci Technol. 2017;77:497–502.
- Soininen SK, Lehtolainen-Dalkilic P, Karppinen T, et al. Targeted delivery via avidin fusion protein: intracellular fate of biotinylated doxorubicin derivative and cellular uptake kinetics and biodistribution of biotinylated liposomes. Eur J Pharm Sci. 2012;47:848–856.
- Yang W, Cheng Y, Xu T, et al. Targeting cancer cells with biotin-dendrimer conjugates. Eur J Med Chem. 2009;44:862–868.
- Pulkkinen M, Pikkarainen J, Wirth T, et al. Three-step tumor targeting of paclitaxel using biotinylated PLA-PEG nanoparticles and avidin-biotin technology: formulation development and in vitro anticancer activity. Eur J Pharm Biopharm. 2008;70:66–74.
- Chen H, Nan W, Wei X, et al. Toxicity, pharmacokinetics, and in vivo efficacy of biotinylated chitosan surface-modified PLGA nanoparticles for tumor therapy. Artif Cells Nanomedicine Biotechnol. 2017;45:1115–1122.
- Zempleni J, Wijeratne SSK, Hassan YI. Biotin. Biofactors. 2009;35:36–46.
- Vadlapudi AD, Vadlapatla RK, Mitra AK. Sodium dependent multivitamin transporter (SMVT): a potential target for drug delivery. Curr. Drug Targets. 2012;13:994–1003.
- Yuan Q, Venkatasubramanian R, Hein S, et al. A stimulus-responsive magnetic nanoparticle drug carrier: magnetite encapsulated by chitosan-grafted-copolymer. Acta Biomater. 2008;4:1024–1037.
- Ahmadi Nasab N, Hassani Kumleh H, Beygzadeh M, et al. Delivery of curcumin by a pH-responsive chitosan mesoporous silica nanoparticles for cancer treatment. Artif Cells Nanomed Biotechnol. 2018;46:75–81.
- Wang F, Wang Y, Ma Q, et al. Development and characterization of folic acid-conjugated chitosan nanoparticles for targeted and controlled delivery of gemcitabinein lung cancer therapeutics. Artif Cells Nanomedicine Biotechnol. 2017;45:1530–1538.
- Pandit J, Sultana Y, Aqil M. Chitosan-coated PLGA nanoparticles of bevacizumab as novel drug delivery to target retina: optimization, characterization, and in vitro toxicity evaluation. Artif Cells Nanomedicine Biotechnol. 2017;45:1397–1407.
- Balan V, Redinciuc V, Tudorachi N, et al. Biotinylated N-palmitoyl chitosan for design of drug loaded self-assembled nanocarriers. Eur Polym J. 2016;81:284–294.
- Pons M, Foradada M, Estelrich J. Liposomes obtained by the ethanol injection method. Int J Pharm. 1993;95:51–56.
- Zarif L. Drug delivery by lipid cochleates. Meth Enzymol. 2005;391:314–329.
- Pawar AP, Vinugala D, Bothiraja C. Nanocochleates derived from nanoliposomes for paclitaxel oral use: preparation, characterization, in vitro anticancer testing, bioavailability and biodistribution study in rats. Biomed Pharmacother. 2014 [Nov 20]; [9 p.]. DOI:10.1016/j.biopha.2014.11.014
- Chu C, Xu P, Zhao H, et al. Effect of surface ligand density on cytotoxicity and pharmacokinetic profile of docetaxel loaded liposomes. Asian J Pharm Sci. 2016;11:655–661.
- Pawar A, Singh S, Rajalakshmi S, et al. Development of fisetin-loaded folate functionalized pluronic micelles for breast cancer targeting. Artif Cells Nanomedicine Biotechnol. 2018 [Jan 15]; [15 p.]. DOI:10.1080/21691401.2018.1423991
- Chen H, Xie LQ, Qin J, et al. Surface modification of PLGA nanoparticles with biotinylated chitosan for the sustained in vitro release and the enhanced cytotoxicity of epirubicin. Colloids Surf B Biointerfaces. 2016;138:1–9.
- Pavia DL, Lampman GM, Kriz GS. Introduction to Spectroscopy third edition. Thomson Learn Inc 2001;15:579.
- Sugimoto M, Morimoto M, Sashiwa H, et al. Preparation and characterization of water-soluble chitin and chitosan derivatives. Carbohydr Polym. 1998;36:49–59.
- Umeyama M, Kira A, Nishimura K, et al. Interactions of bovine lactoferricin with acidic phospholipid bilayers and its antimicrobial activity as studied by solid-state NMR. Biochim Biophys Acta - Biomembr. 2006;1758:1523–1528.
- Li J, Wang X, Zhang T, et al. A review on phospholipids and their main applications in drug delivery systems. Asian J Pharm Sci. 2014;10:81–98.
- Du W, Hong L, Yao T, et al. Synthesis and evaluation of water-soluble docetaxel prodrugs-docetaxel esters of malic acid. Bioorganic Med Chem. 2007;15:6323–6330.
- Wang L, Liu Z, Liu D, et al. Docetaxel-loaded-lipid-based-nanosuspensions (DTX-LNS): preparation, pharmacokinetics, tissue distribution and antitumor activity. Int J Pharm. 2011;413:194–201.
- Pedersen UR, Leidy C, Westh P, et al. The effect of calcium on the properties of charged phospholipid bilayers. Biochim Biophys Acta - Biomembr. 2006;1758:573–582.
- Pawar A, Patel R, Arulmozhi S, et al. d-[small alpha]-Tocopheryl polyethylene glycol 1000 succinate conjugated folic acid nanomicelles: towards enhanced bioavailability, stability, safety, prolonged drug release and synergized anticancer effect of plumbagin. RSC Adv. 2016;6:78106–78121.
- Bothiraja C, Kapare HS, Pawar AP, et al. Development of plumbagin-loaded phospholipid-Tween(R) 80 mixed micelles: formulation, optimization, effect on breast cancer cells and human blood/serum compatibility testing. Ther Deliv. 2013;4:1247–1259.
- Bothiraja C, Yojana BD, Pawar AP, et al. Fisetin-loaded nanocochleates: formulation, characterisation, in vitro anticancer testing, bioavailability and biodistribution study. Expert Opin. Drug Deliv. 2014;11:17–29.
- Nishioka Y, Yoshino H. Lymphatic targeting with nanoparticulate system. Adv Drug Deliv Rev. 2001;47:55–64.
- Soma D, Kitayama J, Ishigami H, et al. Different tissue distribution of paclitaxel with intravenous and intraperitoneal administration. J Surg Res. 2009;155:142–146.