Abstract
Primary liver cancer (PLC) is one of the most common malignant tumours and has the third highest mortality rate worldwide. An active liver-targeting drug delivery system via the asialoglycoprotein receptors expressed in the hepatic parenchyma cells of mammals has become a research focus for the treatment of PLC. N-acetylaminogalactosyl-poly(lactide-co-glycolide)-succinyl-D-α-tocopherol polyethylene glycol 1000 succinate (GalNAc-PLGA-sTPGS) was synthesized to achieve active liver-targeting properties. Emodin (EMO)-loaded GalNAc-PLGA-sTPGS nanoparticles (EGPTN) were prepared with EMO which was selected for its potential antitumour efficacy. The in vitro cellular uptake, mechanism, cytotoxicity, and apoptosis of HepG2 cells were analyzed. The in vivo therapeutic effects of EGPTN were assessed in a PLC mouse model. The results showed that GalNAc-PLGA-sTPGS was successfully synthesized. The cellular uptake assay demonstrated that coumarin 6-loaded GalNAc-PLGA-sTPGS nanoparticles had superior active liver-targeting properties. The results of the cytotoxity and apoptosis studies indicated that EGPTN achieved the highest levels of cytotoxicity and cell apoptotic rate among the nanoparticles examined. Furthermore, EGPTN showed better in vivo therapeutic effects and anticancer efficacy in the PLC mice than did the other groups. Therefore, EGPTN enhanced the anticancer effect of EMO both in vitro and in vivo, making it a potential option for the treatment of PLC.
Graphical Abstract
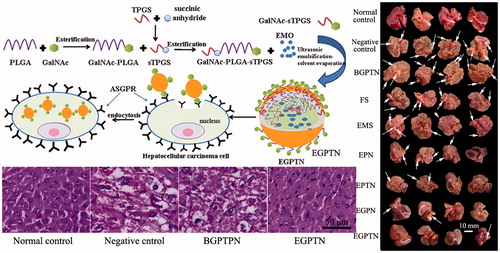
Introduction
Primary liver cancer (PLC), which occurs in liver cells or intrahepatic bile duct epithelial cells, respectively, is one of the most common malignant tumours around the world [Citation1] and has the second leading cause of cancer mortality [Citation2]. The aetiology and pathogenesis of PLC, which may be related to viral hepatitis, liver cirrhosis or drinking water pollution, have not yet been fully clarified. Surgery and radiotherapy are still the main clinical treatments for PLC, and the use of chemotherapy drugs has many drawbacks, such as poor selectivity and obvious side-effects. Currently, targeting therapy for cancer has attracted increasing attention due to its advantages of high specificity, remarkable therapeutic effects and minimal adverse reactions. In particular, cancer targeting agents such as Gleevec and Herceptin have been successfully developed, and they demonstrated significant clinical effects, resulting in their application in a liver cancer-targeting drug delivery system for the treatment of PLC with bright prospects [Citation3,Citation4]. Galactose-targeting drug delivery systems have been an area of intense focus in recent years. The inherent liver cells are mainly composed of hepatic parenchymal cells, non-parenchymal cells and bile duct epithelial cells. In addition, the surfaces of the different kinds of cells have different specific receptors, and there are a large number of asialoglycoprotein receptors (ASGPRs) in the parenchyma cellular membrane of the mammalian liver. Using the chemical structures of specific receptors or binding sites on the targeted cellular membrane, the active targeting delivery drugs can enter the cells through the biologically specific interaction of receptor-ligand binding [Citation5,Citation6]. ASGPR is a glycoprotein that can specifically recognize compounds containing terminal galactosyl or acetylaminogalactosyl units, and each liver cell contains approximately 200 thousand ASGPR binding sites [Citation7]. By modifying (or coupling) a drug, its carrier (or exogenous gene) can be successfully directed to the liver parenchymal cells via galactosyl (Gal) residues or N-acetylaminogalactosyl (GalNAc) residues to achieve a targeting effect.
Emodin (EMO, 1,3,8-trihydroxy-6-methyl anthraquinone, C15H10O5), which is an effective traditional Chinese medicine obtained from such plants as rhubarb giant knot weed and its chemical structure is shown in the Supplementary Materials, has many pharmacological effects, such as liver-protecting capabilities and anti-hepatic fibrosis, anti-viral, anti-bacterial and anti-inflammation functions. Recently, it was reported that EMO has a strong inhibition effect and induces apoptosis in liver, gallbladder and lung cancer cells, among others, and shows a time- and dose-dependence [Citation8,Citation9]. However, the structure of EMO contains many free phenolic hydroxyls, so it is easily oxidized in the air and has poor solubility and low oral bioavailability, which affect its application in clinical carcinoma therapy. In our previous work, EMO-loaded poly(lactide-co-glycolide)-d-α-tocopherol polyethylene glycol 1000 succinate nanoparticles (EPTN) were prepared to improve therapeutic efficacy by ameliorating the poor water solubility and lipid solubility of EMO [Citation10]. In this study, EMO was also dispersed in a newly synthesized biomaterial and formed into nanoparticles, which could increase its stability. At the same time, the newly synthesized material having a specific recognition ability for liver cells was used to impart an active targeting function and better utilize EMO for the treatment of liver cancer.
There are many materials used for the preparation of nanoparticles (NPs). Poly(lactide-co-glycolide) (PLGA) is a polymeric material that is biodegradable, has a non-immunogenic good biological compatibility and has been cleared for use by the Food and Drug Administration (FDA). In addition, PLGA can also slow drug release, extend the circulation times and half-lives of drugs in vivo, and increase their bioavailabilities [Citation11,Citation12]. Due to the strong hydrophilic nature of d-α-tocopherol polyethylene glycol 1000 succinate (TPGS), the drugs could be more completely released to improve the permeability, enhance the absorption in vivo and reduce P-glycoprotein (P-gp)-mediated multiple drug resistance (MDR) [Citation13,Citation14]. Additionally, because of the good emulsification properties of TPGS, NPs prepared with it had a smaller particle size and a higher drug-loading (DL) and encapsulation efficiency (EE) [Citation15,Citation16]. Because ASGPR has a high specificity and is highly expressed on the surface of liver parenchymal cells, the liver-targeting delivery drugs or the genes mediated by it have become a research focus in recent years [Citation16,Citation17]. The glycoprotein binding of Gal or GalNAc residues can be identified by the ASGPRs, and the affinity of GalNAc for ASGPRs is approximately 50 times higher than that of Gal [Citation18,Citation19]. This study used the characteristics of the specific binding of GalNAc to ASGPRs to synthesize a new biomaterial containing GalNAc, PLGA and TPGS, N-acetylaminogalactosyl-poly(lactide-co-glycolide)-succinyl-d-α-tocopherol polyethylene glycol 1000 succinate (GalNAc-PLGA-sTPGS) via an esterification reaction. The GalNAc residues could be specifically identified by the ASGPRs, so the EMO-loaded GalNAc-PLGA-sTPGS NPs (EGPTN) had better active targeting effects for liver cells. The process of targeting cell internalization of nanoparticles prepared with GalNAc-modified polymers is schematically illustrated in Scheme 1.
Methods
Synthesis and characterization of GalNAc-PLGA,GalNAc-sTPGS, GalNAc-PLGA-sTPGS and PLGA-sTPGS
First, TPGS was acylated with succinic anhydride, and the catalysts in the reaction were DMAP. The complete methods used for the synthesis (illustrated in Scheme 2) and characterization of these polymers are delineated in the Supplementary Materials.
Preparation and characterization of the EMO-loaded NPs
The EGPTN were prepared by an ultrasonic emulsification-solvent evaporation method [Citation20,Citation21]: a certain amount of EMO (∼30 mg) and 100 mg of GalNAc-PLGA-sTPGS were dissolved in 8 mL of ethyl acetate. The solution was slowly added to 120 mL of an aqueous solution containing 0.05% (w/v) GalNAc-sTPGS as an emulsifier. The complete methods used for the preparation and characterization of these NPs are described in the Supplementary Materials.
In vitro cellular uptake of NPs
Human hepatocellular carcinoma cell line HepG2 cells were cultured in high-glucose Dulbecco’s modified Eagle’s Medium (H-DMEM, Thermo Fisher Scientific, Waltham, MA), supplemented with 10% foetal bovine serum (FBS, Thermo Fisher Scientific) and 1% antibiotics. Then, the cells were maintained at 37 °C in an incubator with an atmosphere of 5% CO2. C6-loaded nanoparticles were used for the qualitative observation and quantitative analysis of cellular uptake, and the complete methods used for these analyses are delineated in the Supplementary Materials.
Mechanism for cellular uptake of NPs
HepG2 cells in logarithmic growth phase were adjusted to a suspension of 1 × 105 cells/mL and seeded in 6-well plates, which were divided into CGPTN, CGPN, CPTN and CPN groups. After incubation at 37 °C in a humidified atmosphere (5% CO2) for 24 h, the medium was aspirated, replaced with fresh serum-free medium and incubated for 15 min. Then, the cells in the CGPTN, CGPN, CPTN and CPN groups were pre-incubated with the following inhibitors: 20 µg/L GalNAc, 450 mmol/L sucrose, 3 nmol/L methyl-β-cyclodextrin (M-β-CD), 200 μmol/L genistein, 25 mmol/L sodium azide (NaN3) and 10 µg/L cytochalasin B. Each group had a well without inhibitors to which only serum-free medium was added. The complete methods used for these analyses are described in the Supplementary Materials.
In vitro cytotoxicity assays
HepG2 cells were plated in 96-well transparent plates at an initial density of 1 × 104 cells/well. After 24 h of incubation, the medium was replaced with fresh medium containing EGPTN, EGPN, EPTN, EPN or EMO solutions (EMS, 0.1% v/v dimethyl sulfoxide culture medium as the solvent) at 2.5, 5, 10, 20 and 40 μg/mL equivalent EMO concentrations; fluorouracil solutions (FS, commercial fluorouracil for injection) at 2.5, 5, 10, 20 and 40 μg/mL equivalent FS concentrations; and BGPTN suspensions with the same GalNAc-PLGA-TPGS concentrations as EGPTN, which were 8.3, 16.6, 33.3, 66.5 and 133 μg/mL, for 24, 48 and 72 h. The complete methods used for these analyses are delineated in the Supplementary Materials.
In vitro cell apoptosis
The apoptosis of HepG2 cells caused by the EMO-loaded nanoparticles was quantitatively analyzed using the Annexin V-fluorescein isothiocyanate (FITC)/propidium iodide (PI) double staining assay. The apoptosis of HepG2 cells induced by the EMO-loaded NPs was studied qualitatively using the terminal deoxynucleotidyl transferase (TdT)-mediated deoxyuridine triphosphate (dUTP) nick end labelling (TUNEL) assay. To further investigate the apoptosis and active liver-targeting property of EGPTN, human hepatic sinusoidal endothelial cells (HHSECs), which are hepatic non-parenchymal cells without ASGPRs on their surface, were used as a control and disposed using the same protocol. The complete methods used for cell apoptosis are also described in the Supplementary Materials.
In vivo liver targeting properties and therapeutic effects of EGPTN
A total of 190 special pathogen-free (SPF) 6-week-old healthy male mice weighing approximately 20–22 g were obtained from the Dalian Medical University Laboratory Animal Center in order to establish the primary liver cancer (PLC) mouse model. All of the animal experiments in this study were executed according to the guidelines from the Institutional Animal Care and Use Committee (IACUC). The mice were all maintained at a constant temperature (22 ± 1 °C) environment with a 12 h dark-light cycle and fed granular food and purified water before sacrifice. The complete establishment methods for the PLC mouse model [Citation22] are delineated in the Supplementary Materials.
Ninety-six mice were selected randomly from the PLC mouse model group and divided into two groups (EGPTN group and EMS group), which were administered therapeutics by tail vena injection. The complete methods used for evaluating the liver targeting properties of EGPTN are described in the Supplementary Materials.
Eight normal mice were selected randomly as a normal control group and given a tail vein injection of 20% v/v PEG 400-normal saline solution (0.2 mL per mouse). Moreover, 64 mice were selected randomly from the PLC mouse model group and divided into eight groups (n = 8). The complete methods used for evaluating the therapeutic effects of EGPTN are delineated in the Supplementary Materials.
Statistical analysis
The results are presented as the mean ± SD. The statistical comparisons were made by Student’s t-test (SPSS13.0 statistical software) (International Business Machines Corporation, Armonk, NY), and the accepted level of significance was p < .05.
Results
Synthesis and characterization of GalNAc-PLGA-sTPGS, GalNAc-PLGA, PLGA-sTPGS and GalNAc-sTPGS
The successful conjugation of GalNAc-PLGA-sTPGS was further confirmed by performing a 1H nuclear magnetic resonance (1H-NMR) study of all components (as shown in ). The signals at 1.52 and 5.15 ppm (peaks g and b) were assigned to the methyl (–CH3) and CH protons of the PLA segment, respectively. The signal at 4.75 ppm (peak c) was assigned to the CH2 protons of the PGA segment. The peak at 3.58 ppm (peak e) was attributed to the –CH2 protons of the PEO part of TPGS. The peaks at 6.12 (peak a), 4.03 (peak d) and 1.96 (peak f) were assigned to C1–H, the other CH protons linked to hydroxyl groups, and the CH3 group of GalNAc, respectively. The 1H-NMR spectra of the PLGA, PLGA-sTPGS, GalNAc-PLGA and GalNAc-PLGA-sTPGS copolymers also exhibited peaks in similar regions because they contained PLGA groups. The molecular weight of GalNAc-PLGA-sTPGS was calculated using the ratio between the peak areas of the peaks at 5.15 (peak area 7.33), 4.75 (peak area 14.79) and 3.58 (peak area 3.10). The number average molecular weight (Mn) of the GalNAc-PLGA-sTPGS random copolymer was determined to be 28,736. The ratio of the PLGA and TPGS molecular masses integrated into the GalNAc-PLGA-sTPGS copolymer were approximately 92.43 and 6.36%, respectively. The complete results (as shown in Supplementary Figures S1–S3) of Fourier transform infrared (FTIR) spectra, gel permeation chromatography (GPC) and differential scanning calorimetry (DSC) analyses for these polymers are delineated respectively in the Supplementary Materials.
Preparation and characterization of EMO-loaded NPs
The particle size, particle size distribution (Supplementary Figure S4) and ζ-potential are important characteristics of NPs from which the stability of the EMO-loaded nanoparticles could be predicted, as listed in . The average particle sizes of EGPTN and EGPN were both less than those of EPTN and EPN. At the same time, all the NPs had a negative charge on the surface, and the absolute value of the ζ-potential of EGPTN and EGPN was greater than that of EPTN and EPN. The particle size, particle size distribution and ζ-potential of the C6-loaded nanoparticles were consistent with the trend observed for the EMO-loaded nanoparticles. High-resolution images of all NPs were obtained using a transmission electron microscope (TEM, electric voltage of 120.0 kV, JEM-2000EX, Japan Electron Optics Laboratory Co., Ltd, Tokyo, Japan), as shown in . The NPs had moderate uniformity with a spherical or quasi-spherical shape and were dispersed evenly without adhesion, and the particle size was uniform. The average particle sizes of all the NPs except for EPN and CPN were in the range of 100–200 nm, which were similar to the sizes obtained from the dynamic light scattering method. All the NPs were negatively charged and demonstrated ζ-potentials between −30 mV and −10 mV. The complete results of DLs, EEs, in vitro drug cumulative release profiles (as shown in Supplementary Figure S5), and DSC analysis (as shown in Supplementary Figure S6) for these NPs are described in the Supplementary Materials.
Figure 2. Characterization images of drug-loaded nanoparticles. A(a), A(b), A(c) and A(d) are TEM images of EMO-loaded PLGA nanoparticles (EPN), EMO-loaded PLGA-sTPGS nanoparticles (EPTN), EMO-loaded GalNAc-PLGA nanoparticles (EGPN) and EMO-loaded GalNAc-PLGA-sTPGS nanoparticles (EGPTN), respectively. B(a), B(b), B(c) and B(d) are TEM images of C6-loaded PLGA nanoparticles (CPN), C6-loaded PLGA-sPTGS nanoparticles (CPTN), C6-loaded GalNAc-PLGA nanoparticles (CGPN) and C6-loaded GalNAc-PLGA-sTPGS nanoparticles (CGPTN), respectively.
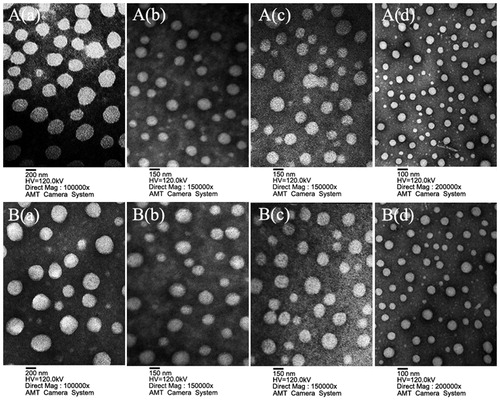
Table 1. Characterization of NPs. (n = 6, mean ± SD).
In vitro cellular uptake of NPs
To study the cellular uptake of the NPs, C6 as a fluorescent marker was encapsulated in the nanoparticles and observed by a confocal laser scanning microscopy (CLSM) (Leica TCS SP5; Leica Microsystems Co. Ltd., Solms, Germany) [Citation23]. As shown in , all the nuclei of HepG2 cells stained with DAPI exhibited blue fluorescence, and the fluorescence intensity of CGPTN in HepG2 cells was significantly higher than that of CPN, CPTN and CGPN. This result illustrated that the nanoparticles prepared with GalNAc-PLGA-sTPGS as a carrier was identified in HepG2 cells by the ligand receptor (ASGPRs). Furthermore, to verify the uptake efficiency of the C6-loaded nanoparticles in HepG2 cells, the concentration of C6 in the cells was determined by HPLC. The concentrations and cellular uptake rates of C6 in CGPTN, CGPN, CPTN, and CPN that was internalized by HepG2 cells after 30 and 60 min are also shown in . The results indicated that the cellular uptake rates of C6 in HepG2 cells incubated with CGPN and CGPTN increased by 43.6 and 58.1% (p < .01), respectively, and by 32.9 and 47.4% (p < .05) compared with CPN and CPTN at 60 min, respectively.
Figure 3. (A) The confocal laser scanning microscopy (CLSM) images of HepG2 cells after 30 and 60 min incubation with C6-loaded NPs suspensions, respectively. (B) The concentrations and cellular uptake rates of C6 in CPN, CPTN, CGPN and CGPTN internalized by HepG2 cells after incubation for 30 and 60 min, respectively (n = 6, mean ± SD; (a) p < .05 versus corresponding CPTN; (b) p < .01 versus corresponding CPN).
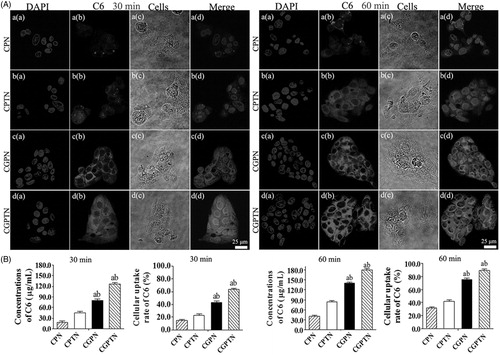
Mechanism for the cellular uptake of NPs
The relative fluorescence intensity (RFI) of each group was plotted, as shown in and Supplementary Figure S7. The fluorescence intensity of CGPTN without any inhibitors was used as the control (the fluorescence intensity was 100%), and the RFIs of CPTN and CPN were significantly decreased (reduced by 33.9% and 58.7, respectively, p < .05 or p < .01). The complete results of a mechanism for these NPs are delineated in the Supplementary Materials.
Figure 4. Effects of inhibitors on relative fluorescence intensity of CGPTN, CGPN, CPTN, CPN groups in HepG2 cells (n = 6, mean ± SD. (a) p < .05, (b) p < .01 versus corresponding CGPTN control group; (c) p < .05, (d) p < .01 versus corresponding CGPN control group; (e) p < .05, (f) p < .01 versus corresponding CPTN control group; (g) p < .05, (h) p < .01 versus corresponding CPN control group).
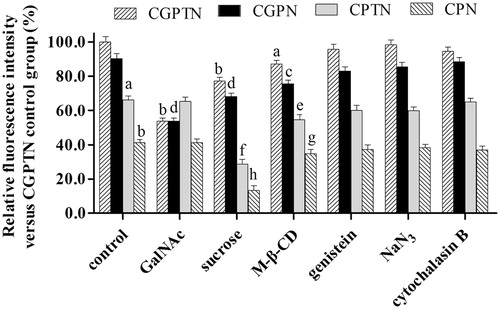
In vitro cytotoxicity assays
The in vitro cytotoxicities of FS, EMS, EPN, EPTN, EGPN and EGPTN against HepG2 cells were determined using the WST-1 assay, and the results are shown in Supplementary Figure S8. The viability ratio (VR, %) of cells for the EMO-loaded nanoparticles decreased gradually with increasing incubation time and drug dose. In addition, after 24, 48 and 72 h, the VR of cells for BGPTN exhibited no significant change, which showed that the newly synthesized biomaterial GalNAc-PLGA-sTPGS had no toxicity to cells. The complete results of in vitro cytotoxicity assays and the half inhibitory concentration (IC50) values for these NPs (listed in Supplementary Table S1) are described in the Supplementary Materials.
In vitro cell apoptosis
The proportion of cells undergoing apoptosis was increased significantly in the NP group compared with the negative control group, and the content of apoptotic cells was relatively low in the positive control FS group, as listed in and Supplementary Figure S9. The results of the TUNEL assay demonstrated that all cellular nuclei were stained with DAPI and exhibited blue fluorescence in all groups, as shown in . The apoptotic results in HHSECs, determined with the TUNEL assay and decreased significantly compared with those HepG2 cells (as shown in Supplementary Figure S10). The complete results of in vitro cell apoptosis are also delineated in the Supplementary materials.
Figure 5. The fluorescence inversion microscope (FIM) images of apoptotic HepG2 cells induced by positive control (added DNase I), negative control (without any drug), BGPTN, FS, EMS, EPN, EPTN, EGPN and EGPTN after incubation for 24 and 72 h using TUNEL assays. Only apoptotic cellular nuclei displayed green fluorescence while all the nuclei were blue stained by DAPI.
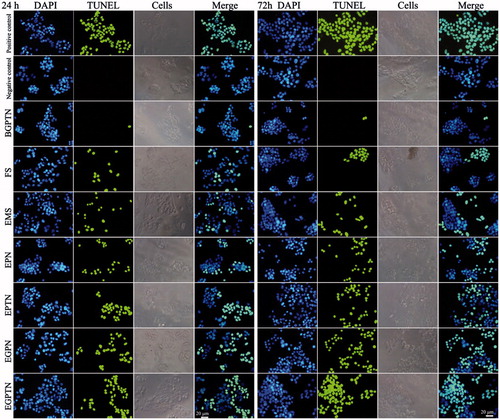
Table 2. Effect on apoptosis of HepG2 cells induced by FS, EMS, EPN, EPTN, EGPN and EGPTN after 24 and 72 h of incubation time in vitro (n = 3, mean ± SD).
In vivo liver targeting properties and therapeutic effects of EGPTN
The concentrations of EMO in the different organs and plasma of EGPTN group and EMS group mice were measured by HPLC. As shown in Supplementary Figure S11A, the concentrations of EMO reached maxima after injecting EGPTN for 1 h, and EMO was released slowly over 24 h into the liver of the mice. However, EMO could not be detected after approximately 4 h in the plasma and liver after injecting EMS (shown in Supplementary Figure S11B). These results illustrated that EGPTN could effectively prolong the retention time and significantly increase the concentration of EMO in the liver. As listed in Supplementary Tables S2 and S3, the four detection indicators of TI, SI, Re and Te were used to measure the liver targeting of EMO. TI and SI were greater than 3.0 except for the SI in plasma and heart at 0.08 h (Supplementary Table S2). These results illustrated that EGPTN had good liver targeting property at different timepoints. More also observed in Supplementary Table S3, the Te of EGPTN was all greater than 22.0 and ReEMO of the liver was the largest (74.7-fold than AUCEMS), which further indicated that EGPTN had good liver-targeting property.
The morphologies of the sections and tumour nodules of the livers of each group are illustrated in , respectively. The liver surface of the normal control group was smooth, reddish in colour, and sharp edges. However, the liver surfaces of the model groups had hard, grey nodules varying in size from 3 to 10 mm. The determination results of the liver function are listed in and complete results are described in the Supplementary Materials. In addition, a lower body weight was observed in the negative control group due to liver damage and a decrease in the synthetic and metabolic liver functions during the treatment. However, the body weight gain ratio in the EGPTN group increased rapidly because EGPTN had a certain therapeutic effect on the mouse liver (as shown in Supplementary Figure S12).
Figure 6. (A) Representative images of excised livers from the normal control and primary liver cancer (PLC) mice treated with 20% (v/v) PEG400-normal saline solution (negative control), BGPTN, FS, EMS, EPN, EPTN, EGPN and EGPTN groups, respectively. (B)Tumour nodules in liver of PLC mice treated with 20% (v/v) PEG400-normal saline solution (negative control), BGPTN, FS, EMS, EPN, EPTN, EGPN and EGPTN groups after 30 days (n = 8, mean ± SD. (a) p < .05 versus corresponding EGPN group; (b) p < .01 versus corresponding EGPTN group). (C) The representative liver slices of each group stained with H&E and observed using microscope (400×). C(a–i) are H&E images of the normal control, negative control, BGPTN, FS, EMS, EPN, EPTN, EGPN and EGPTN groups, respectively.
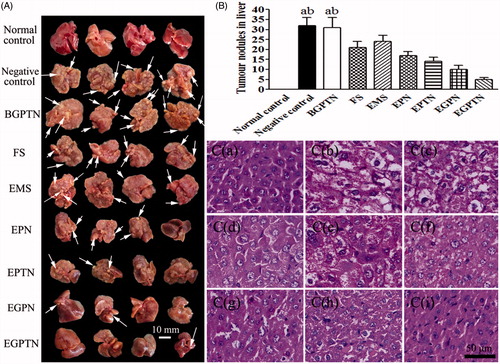
Table 3. Liver functional indexes in the serum and liver of the primary liver cancer mice (n = 8, mean ± SD).
In the hepatic slices after H&E staining, the lesions of internal structure, cell differentiation, nuclear and cytoplasmic changes were observed under an optical microscope. Typical H&E staining images are shown in . The hepatocarcinoma tissues were graded according to the Edmonson-Steiner classification method [Citation24]. In Grade I, eosinophilic staining was observed, the tumour cells were similar to normal liver cells, and the cytoplasm was abundant. Grade II had cancer cells with abundant cytoplasm, increased eosinophilic staining, irregular shapes and slightly larger nuclei. Grade III had basophilic staining, the shape of the cancer cells was polymorphic, and the nuclei were large and irregular. Moreover, tumour giant cells began to appear. Grade IV had obviously polymorphic cancer cells, which were arranged loosely and were without structure. Furthermore, Grade IV was also associated with increased levels of tumour giant cells, and the karyoplasm proportion increased significantly. The tissues from the mice in the normal control group had structural integrity, and the cells were uniform and regular ()). There was no significant difference between the negative control group and the BGPTN group () and (C-c)), which were assigned to Grade IV. The cancer cells in the FS group partially belonged to Grade II ()). Compared with the EMO-loaded NP groups ()–()), a number of the cancer cells in the EMS group belonged Grade III ()). It was illustrated that the anticancer effect of EMS was poor. However, the majority of the cancer cells in the EGPTN group belonged to GradeI ()), which further showed that EGPTN not only had an inhibitory effect on the proliferation and differentiation of liver cancer cells but also protected against liver cancer.
Discussion
For the first time, the GalNAc-PLGA-sTPGS, GalNAc-PLGA, GalNAc-sTPGS, PLGA-sTPGS copolymers were synthesized as NP carriers, and FTIR, 1H-NMR, DSC and GPC experiments were used to confirm that these copolymers had been successfully synthesized.
All nanoparticles prepared in this study belonged to nanospheres. In brief, EMO (or C6) and materials were dissolved in ethyl acetate with molecular form. TPGS or GalNAc-sTPGS was used as emulsifiers to make the water phase (120 mL of 0.05% w/v emulsifier aqueous solution) and oil phase (8 mL of ethyl acetate) become O/W emulsion. Then the emulsion was stirred for 24 h at room temperature to get rid of ethyl acetate, and the drugs were encapsulated into materials and separated out from the water phase. EGPTN (or CGPTN) prepared with GalNAc-sTPGS as emulsifier was in a structure with the inner hydrophobic tocopherol group in TPGS unit encapsulating EMO and the outer hydrophilic GalNAc group in GalNAc-sTPGS and GalNAc-PLGA unit point to the outside of the NPs (as shown in Scheme 1), so that the GalNAc groups on the surface of EGPTN (or CGPTN) could combine with ASGPRs in hepatocellular membrane via receptor-ligand-mediated active targeting which could more easily be internalized by hepatic cancer cells.
Drugs incorporated in NPs can be delivered to the targeted site to the maximum extent, resulting in a higher concentration of the drug in the targeted site relative to the traditional preparation methods, a significantly improved treatment effect, and a reduction in the adverse reactions of the drug [Citation25–27]. In the present study, a small molecular drug, EMO, was used as a model to prepare to EMO-loaded nanoparticles, and the particle sizes of these NPs, except for EPN, were in the range from 100 to 200 nm. It was previously reported that the size of the NPs could affect their tissue distribution in vivo, in which the NPs from 150 to 200 nm were retained in the liver and spleen [Citation26]. The average particle size of EGPTN was smaller than that of EGPN, and the average particle size of EPTN was also smaller than that of EPN. This was because TPGS was an excellent emulsifier and had a substantial emulsifying effect in the GalNAc-PLGA-sTPGS and PLGA-sTPGS copolymers, resulting in the reduction of the surface energy of the particles and prevention of aggregation and flocculation of the particles [Citation15]. The ζ-potential of the NPs was decided jointly by the particle surface charge and the surrounding solution environment. The ζ-potential could affect the stability of NPs formulation, and the greater the absolute value of the ζ-potential was, the better the stability of the dispersed NP system was [Citation27]. As listed in , the absolute values of the ζ-potential of EGPTN and EGPN were significantly larger than that of the other NPs, which could increase the stability and be conducive to the application of the NPs.
In this research, HepG2 cells were used as model cells to investigate the uptake characteristics of C6-loaded NPs because they are a type of hepatic parenchymal cells with a large amount of ASGPRs expressed on the surface, which are specific for the identification of terminal Gal or GalNAc groups, and thus, these cells could be better for evaluating the ability of CGPTN to be internalized easily and quickly via receptor-ligand-mediated active targeting [Citation7,Citation28]. CPN, CPTN, CGPN and CGPTN were prepared to discuss the cellular uptake efficiency by HepG2 cells, which greatly influenced the therapeutic effect of the drug-loaded NPs. Because the model drug EMO did not exhibit fluorescence in cells, C6, as a fluorescent probe, was encapsulated in the NPs to perform quantitative and qualitative analyses of the cellular uptake of drug-loaded NPs. C6 was selected for its perfect biocompatibility, high fluorescence ability and a low leakage rate [Citation23]. The results showed that the cellular uptake efficiencies of CGPTN and CGPN were much higher than those of CPTN and CPN at different timepoints; this was because GalNAc, as ligand, could combine specifically with ASGPRs to achieve active targeting of the drug-loaded nanoparticles to liver cancer cells [Citation29].
In this study, the RFI was also used as an evaluation index to investigate the effects of the addition of the exogenous inhibitor GalNAc on cellular uptake. After the addition of a GalNAc solution, the RFIs of CPTN and CPN were almost unchanged, while those of CGPTN and CGPN decreased significantly (p < .01). These results illustrated that the pathway of CGPTN and CGPN into HepG2 cells was related to the receptor-ligand-mediated active transport process, and the presence of GalNAc in the materials of CGPTN and CGPN could improve the active targeting ability of the NPs, but the ASGPRs on the surface of HepG2 cells was inhibited competitively by the addition of exogenous GalNAc, leading to a relative reduction in the cellular uptake of CGPTN and CGPN. Moreover, the RFIs of the CPTN and CPN control groups were lower than those of the CGPTN and CGPN control groups, which further showed that HepG2 cells had a higher cellular uptake of CGPTN and CGPN (consistent with the results of In vitro cellular uptake of NPs in this study). After binding to the ASGPRs on the surface of HepG2 cells at a high level, CGPTN and CGPN entered via a variety of internalization pathways, and the cell internalization process of the NPs had energy dependence. Different inhibitors, such as sucrose and M-β-CD, were used to investigate the cellular uptake mechanism of NPs. Hypertonic sucrose can inhibit clathrin- and caveolin-mediated endocytosis via the destruction of caveolae formation [Citation30]. M-β-CD is a water solution of cyclic glucopyranose oligomers and has a certain effect on clathrin- and caveolin-mediated endocytosis (these two approaches require specific lipids and are dependent on cholesterol) [Citation31]. The RFI of each group decreased a certain extent with the addition of sucrose and M-β-CD. However, genistein, NaN3 and cytochalasin B had a minimal inhibitory effect on these NPs. These results indicated that the cellular uptake of CGPTN and CGPN by HepG2 cells was enhanced mainly on the targeting cells through the interaction of the GalNAc ligand with ASGPR, and this process could be mediated by caveolin and clathrin.
The rapid growth of cancer results from increased proliferation and decreased apoptosis of cancer cells and drug-induced cancer cell apoptosis is a feasible method for cancer control and therapy [Citation32]. In this study, apoptotic cells were detected with the Annexin V-FITC/PI assay. Cells that were stained only with PI were mechanically damaged, and those that were stained with Annexin V-FITC alone were early apoptotic cells; doubly stained cells were in the late stages of apoptosis [Citation33]. Cells in an apoptotic event activate a number of DNA enzymes that can cut genomic DNA between nuclei. When the genomic DNA is fractured, the exposed 3'-OH can be labelled with fluorescein-dUTP via mediation by TdT, and all apoptotic cellular nuclei exhibit green fluorescence under a fluorescence microscope. shows that the cell apoptotic ratio of EMO-loaded nanoparticles was higher than that of EMS, and the apoptotic ratios of FS and EMS at 72 h showed no obvious increase in comparison to those at 24 h. This result was because the NPs were directly internalized into HepG2 cells without a biological membrane transport process, and FS and EMS had no sustained release properties [Citation15]. Therefore, there were many NPs in the cells, and EMO could be released through the function of the lysosomes and have a stronger effect in promoting apoptosis. Meanwhile, flow cytometry and a TUNEL assay showed that EGPTN had the highest cell apoptotic ratio. This high ratio was mainly because the HepG2 cellular membrane surface expresses substantial amounts of ASGPRs, and the GalNAc-PLGA-sTPGS synthesized by us could achieve active targeting on liver cancer cells due to the presence of GalNAc as one of the specific ligands of ASGPR [Citation34]. Compared with EGPN, EGPTN had several better properties, such as a higher cellular uptake and complete drug release, which were also confirmed by the In vitro cellular uptake of NPs and cytotoxicity assays in this study. Consequently, EGPTN exhibited a stronger effect than EGPN on the apoptosis of HepG2 cells. The apoptotic ratio in HHSECs determined with the TUNEL assay (shown in Supplementary Figure S10) decreased significantly at 72 h because no ASGPR was expressed in HHSECs, while the ASGPR present in HepG2 cells could recognize and bind to the GalNAc residues [Citation35] in EGPTN. This difference further illustrated qualitatively and quantitatively that EGPTN, prepared with GalNAc-PLGA-sTPGS as a carrier, could not only improve the drug uptake by HepG2 cells to enhance the inhibitory effect on cancer cells but could also induce a higher apoptotic ratio; thus, EGPTN had a stronger therapeutic effect in the treatment of liver cancer.
AST, ALT, TP, ALB, γ-GT and T-BIL are commonly used indicators in clinical liver function assessments. Among these indicators, AST and ALT mainly reflect the damage to liver cells, TP and ALB mainly reflect the synthetic function of the liver, and T-BIL mainly reflects the metabolic function of liver cells. Γ-GT is often associated with the malignant transformation of, cells which is not expressed in mature liver cells. In the course of animal liver cancer, γ-GT is regarded as a positive marker of early hepatocellular variation and as the characteristic index of a precancerous liver lesion [Citation36]. Compared with the normal control group, the contents of TP and ALB significantly decreased and that of T-BIL increased (p < .01), which indicated that the synthetic and metabolic functions of the liver seriously declined in the negative control group. The content of γ-GT clearly increased (p < .01), which indicated that serious pathological changes were promoted in the liver, and γ-GT met the requirements for the PLC model. In contrast, the activity of γ-GT in the EGPN and EGPTN groups was significantly lower than that in the negative control group. The results indicated that EGPN and EGPTN reduced the activity of γ-GT in the serum and liver and inhibited the formation of liver cancer in mice.
Simultaneously, the H&E staining results also showed that the cell grade in the EGPTN group more frequently belonged to Grade I compared with that in the EPN and EPTN groups (). This result was because a large number of ASGPRs exist on the cell membranes of liver parenchymal cells, and EGPTN, prepared with GalNAc-PLGA-sTPGS and GalNAc-sTPGS that contains GalNAc groups, could be specifically recognized by ASGPRs, which increased the active liver-targeting properties of EGPTN and facilitated the targeting of EMO to the liver. The EMO-loaded nanoparticles had a good inhibitory effect on liver cancer cells and had a partial therapeutic effect. The results of the liver function index determination also showed that, compared with EMS, EPN, EPTN and EGPN, EGPTN significantly inhibited tumour cells, and the inhibition efficiency of EGPTN was the highest, which was consistent with the results of In vitro cytotoxicity assays and cell apoptosis in this study. These results further proved that the GalNAc-modified EGPTN not only had a good liver targeting ability and in vivo anticancer activity but also had a better therapeutic effect on the PLC mice. In conclusion, the results indicated that the newly synthesized GalNAc-PLGA-sTPGS material had better cell and tissue compatibility both in vivo and in vitro, and the EGPTN prepared with the material had superior anticancer effects in vivo and in vitro as well as strong active liver-targeting properties. The therapeutic effects of EGPTN for PLC were significantly enhanced compared with those of EMS and EPN, and thus, EGPTN is expected to become a new choice for the treatment of PLC in the clinic.
In this research, GalNAc-PLGA-sTPGS was synthesized for the first time as a promising material, and at the same time, GalNAc-sTPGS as an emulsifier, GalNAc-PLGA and PLGA-sTPGS as controls were also synthesized. Compared with free EMO, EGPTN exhibited a stronger effect on the growth of HepG2 cells in vitro and in PLC mice in vivo, which indicated that EGPTN had a better active targeting function and a stronger inhibitory effect on liver cancer cells that have high levels of ASGPR expression. Consequently, EGPTN, prepared with the newly synthesized GalNAc-PLGA-sTPGS biomaterial, has potential to be an effective active targeting therapy for PLC in the clinic.
Abbreviations | ||
ASGPR | = | Asialoglycoprotein receptor |
BGPTN | = | Blank GalNAc-PLGA-sTPGS nanoparticles |
C6 | = | Coumarin-6 |
CGPN | = | C6-loaded GalNAc-PLGA nanoparticles |
CGPTN | = | C6-loaded GalNAc-PLGA-sTPGS nanoparticles |
CPN | = | C6-loaded PLGA nanoparticles |
CPTN | = | C6-loaded PLGA-sTPGS nanoparticles |
DAPI | = | 4,6-diamidino-2-phenylindole dihydrochloride |
DEN | = | Diethylnitrosamine |
DL | = | Drug loading |
DMAP | = | 4-dimethylaminopyridine |
DMF | = | Dimethylformamide |
EE | = | Encapsulation efficiency |
EGPN | = | EMO-loaded GalNAc-PLGA nanoparticles |
EGPTN | = | EMO-loaded GalNAc-PLGA-sTPGS nanoparticles |
EMO | = | Emodin |
EMS | = | EMO solutions |
EPN | = | EMO-loaded PLGA nanoparticles |
EPTN | = | EMO-loaded PLGA-sTPGS nanoparticles |
FS | = | Commercial 5-fluorouracil for injection solutions |
GalNAc | = | d-α-N-acetylaminogalactosyl |
GalNAc-PLGA | = | N-acetylaminogalactosyl-poly(lactide-co-glycolide) |
GalNAc-PLGA-sTPGS | = | N-acetylaminogalactosyl-poly(lactide-co-glycolide)-succinyl-d-α-tocopherol polyethylene glycol 1000 succinate |
GalNAc-sTPGS | = | N-acetylaminogalactosyl-succinyl-d-α-tocopherol polyethylene glycol 1000 succinate |
IC50 | = | Half inhibitory concentration |
MSA | = | Methanesulfonic acid |
NPs | = | Nanoparticles |
PDI | = | Polydispersity index |
PEG400 | = | Polyethylene glycol 400 |
PLC | = | Primary liver cancer |
PLGA | = | Poly(lactide-co-glycolide) |
RFI | = | Relative fluorescence intensity |
SDS | = | Sodium dodecyl sulfate |
sTPGS | = | Succinyl-d-α-tocopherol polyethylene glycol 1000 succinate |
TPGS | = | d-α-tocopheryl polyethylene glycol 1000 succinate |
VR | = | Viability ratio |
Supplemental Material
Download PDF (1.1 MB)Disclosure statement
The authors declare no conflicts of interest.
Additional information
Funding
References
- Parkin DM. Global cancer statistics in the year 2000. Lancet Oncol. 2001;2:533–543.
- Lafaro KJ, Demirjian AN, Pawlik TM. Epidemiology of hepatocellular carcinoma. Surg Oncol Clin N Am. 2015;24:1–17.
- O'Dwyer ME, Mauro MJ, Druker BJ. Recent advancements in the treatment of chronic myelogenous leukemia. Annu Rev Med. 2002;53:369–381.
- Sakamoto G, Mitsuyama S. New molecule-targeting therapy with herceptin (trastuzumab), an anti-HER2 (c-erB-2) monoclonal antibody. Breast Cancer. 2000;7:350–357.
- Ashwell G, Harford J. Carbohydrate-specific receptors of the liver. Annu Rev Biochem. 1982;51:531–554.
- Trere D, Fiume L, De Giorgi LB, et al. The asialoglycoprotein receptor in human hepatocellular carcinomas: its expression on proliferating cells. Br J Cancer. 1999;81:404–408.
- Schwartz AL, Fridovich SE, Lodish HF. Kinetics of internalization and recycling of the asialoglycoprotein receptor in a hepatoma cell. Lin J Biol Chem. 1982;257:4230–4237.
- Hsu CM, Hsu YA, Tsai Y, et al. Emodin inhibits the growth of hepatoma cells: finding the common anti-cancer pathway using Huh7, Hep3B, and HepG2 cells. Biochem Biophys Res Commun. 2010;392:473–478.
- Wang QJ, Cai XB, Liu MH, et al. Apoptosis induced by emodin is associated with alterations of intracellular acidification and reactive oxygen species in EC-109 cells. Biochem Cell Biol. 2010;88:767–774.
- Liu H, Gao M, Xu H, et al. A promising emodin-loaded poly (lactic-co-glycolic acid)-d-α-tocopheryl polyethylene glycol 1000 succinate nanoparticles for liver cancer therapy. Pharm Res. 2016;33:217–236.
- Jain SK, Gupta Y, Ramalingam L, et al. Lactose-conjugated PLGA nanoparticles for enhanced delivery of rifampicin to the lung for effective treatment of pulmonary tuberculosis. PDA J Pharm Sci Technol. 2010;64:278–287.
- Jain AK, Das M, Swarnakar NK, et al. Engineered PLGA nanoparticles: an emerging delivery tool in cancer therapeutics. Crit Rev Ther Drug Carrier Syst. 2011;28:1–45.
- Varma MV, Panchagnula R. Enhanced oral paclitaxel absorption with vitamin E-TPGS: effect on solubility and permeability in vitro, in situ and in vivo. Eur J Pharm Sci. 2005;25:445–453.
- Collnot EM, Baldes C, Schaefer UF, et al. Vitamin E TPGS P-glycoprotein inhibition mechanism: influence on conformational flexibility, intracellular ATP levels, and role of time and site of access. Mol Pharm. 2010;7:642–651.
- Guo Y, Luo J, Tan S, et al. The applications of Vitamin E TPGS in drug delivery. Eur J Pharm Sci. 2013;49:175–186.
- Liang M, Zheng X, Tu L, et al. The liver-targeting study of the N-galactosylated chitosan in vivo and in vitro. Artif Cells Nanomed Biotechnol. 2014;42:423–428.
- Craparo EF, Teresi G, Licciardi M, et al. Novel composed galactosylated nanodevices containing a ribavirin prodrug as hepatic cell-targeted carriers for HCV treatment. J Biomed Nanotechnol. 2013;9:1107–1122.
- Iobst ST, Drickamer K. Selective sugar binding to the carbohydrate recognition domains of the rat hepatic and macrophage asialoglycoprotein receptors. J Biol Chem. 1996;271:6686–6693.
- Rensen PC, Sliedregt LA, Ferns M, et al. Determination of the upper size limit for uptake and processing of ligands by the asialoglycoprotein receptor on hepatocytes in vitro and in vivo. J Biol Chem. 2001;276:37577–37584.
- Sengupta D, Chowdhury KD, Sarkar A, et al. Berberine and S allyl cysteine mediated amelioration of DEN + CCl4 induced hepatocarcinoma. Biochim Biophys Acta. 2014;1840:219–244.
- Bao X, Gao M, Xu H, et al. A noval oleanolic acid-loaded PLGA-TPGS nanoparticle for liver cancer treatment. Drug Dev Ind Pharm. 2015;41:1193–1203.
- Guan X, Gao M, Xu H, et al. Quercetin-loaded poly (lactic-co-glycolic acid)-d-α-tocopheryl polyethylene glycol 1000 succinate nanoparticles for the targeted treatment of liver cancer. Drug Deliv. 2016;23:3307–3318.
- Panyama J, Sahoo SK, Prabha S, et al. Fluorescence and electron microscopy probes for cellular and tissue uptake of poly(d,l-lactide-co-glycolide) nanoparticles. Int J Pharm. 2003;262:1–11.
- Edmondson HA, Steiner PE. Primary carcinoma of the liver: a study of 100 cases among 48,900 necropsies. Cancer. 1954;7:462–503.
- Arias JL, López-Viota M, Gallardo V, et al. Chitosan NPs as a new delivery system for the chemotherapy agent tegafur. Drug Dev Ind Pharm. 2010;36:744–750.
- Mehnert W, Mäder K. Solid lipid nanoparticles: production, characterization and applications. Adv Drug Deliv Rev. 2001;47:165–196.
- Ma Y, Huang L, Song C, et al. Nanoparticle formulation of poly(3-caprolactone-co-lactide)-D-α-tocopheryl polyethylene glycol 1000 succinate random copolymer for cervical cancer treatment. Polymer. 2010;51:5952–5959.
- Schwartz AL, Fridovich SE, Knowles BB, et al. Characterization of the asialoglyco- protein receptor in a continuous hepatoma line. J Biol Chem. 1981;256:8878–8881.
- Wang HX, Xiong MH, Wang YC, et al. N-acetylgalactosamine functionalized mixed micellar nanoparticles for targeted delivery of siRNA to liver. J Control Release. 2013;166:106–114.
- Hao X, Wu J, Shan Y, et al. Caveolae-mediated endocytosis of biocompatible gold nanoparticles in living Hela cells. J Phys Condens Matter. 2012;24:164207.
- Nynca A, Nynca J, Wąsowska B, et al. Effects of the phytoestrogen, genistein, and protein tyrosine kinase inhibitor-dependent mechanisms on steroidogenesis and estrogen receptor expression in porcine granulosa cells of medium follicles. Domest Anim Endocrinol. 2013;44:10–18.
- Choi SH, Park JY, Kang W, et al. Knockdown of HIF-1α and IL-8 induced apoptosis of hepatocellular carcinoma triggers apoptosis of vascular endothelial cells. Apoptosis. 2016;21:85–95.
- Zhang H, Hou X, Lin M, et al. The study on the preparation and characterization of gene-loaded immunomagnetic albumin nanospheres and their anti-cell proliferative effect combined with magnetic fluid hyperthermia on GLC-82 cells. Drug Des Devel Ther. 2015;9:6445–6460.
- Medina SH, Tekumalla V, Chevliakov MV, et al. N-acetylgalactosamine-functionalized dendrimers as hepatic cancer cell-targeted carriers. Biomaterials. 2011;32:4118–4129.
- Roggenbuck D, Mytilinaiou MG, Lapin SV, et al. Asialoglycoprotein receptor (ASGPR): a peculiar target of liver-specific autoimmunity. Autoimmun Highlights. 2012;3:119–125.
- Peng Q, Zhang Q, Xiao W, et al. Protective effects of Sapindus mukorossi Gaertn against fatty liver disease induced by high fat diet in rats. Biochem Biophys Res Commun. 2014;450:685–691.