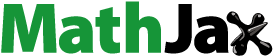
Abstract
Different forms of biopolyester PHBVHHx microspheres were prepared so as to compare the mammalian cell behaviors in suspension cultivation system. Based on a microbial terpolyester PHBVHHx consisting of 3-hydroxybutyrate (HB), 3-hydroxyvalerate (HV), and 3-hydroxyhexanoate (HHx), solid microspheres (SMSs), hollow microspheres (HMSs), and porous microspheres (PMS) were successfully prepared by a modified solvent evaporation method involving gas-in-oil-in-water (G1/O/W2) double emulsion, water-in-oil-in-water (W1/O/W2) double emulsion and oil-in-water (O/W) single emulsion, respectively. Generally, PMSs have diameters ranging from 330 to 400 μm with pore sizes of 10 to 60 μm. The pores inside the PMSs were found well interconnected compared with PHBVHHx prepared by the traditional solvent evaporation method, resulting in the highest water uptake ratio. When inoculated with human osteoblast-like cells lasting 6 days, PMS showed much better cell attachment and proliferation compared with other less porous microspheres due to its large inner space as a 3 D carrier. Cell migration towards surface and other interconnected inner pores was clearly observable. Dead or apoptotic cells were found more common among less porous SMSs or HMSs compared with highly porous PMSs. It is therefore concluded that porous PHBVHHx microspheres with larger surface open pores and interconnected inner pores can serve as a carrier or scaffold supporting more and better cell growth for either injectable purposes or simply supporting cell growth.
Introduction
In tissue engineering and regeneration medicine, ideal biomaterial scaffolds should meet requirements including good biocompatibility, reasonable biodegradability, and porous structure with well inter-connected space for cell growth [Citation1–3]. More and more biomaterials and their scaffolds have been developed, prepared and evaluated recently [Citation3]. A novel member of microbial polyhydroxyalkanoates (PHA) terpolyester, namely, poly(3-hydroxybutyrate-co-3-hydroxyhexanoate-co-3-hydroxyvalerate) (PHBVHHx), was reported successfully used in tissue engineering [Citation4–6], PHBVHHx was found to stimulate a rapid increase of cytosolic calcium influx in HaCaT cells, leading to enhanced cell growth. The in vitro and in vivo studies on PHBVHHx showed that PHBVHHx promoted cell attachment and proliferation of fibroblast cell line L929 and osteoblast cell line MC3T3 [Citation5]. PHBVHHx also exhibited better proliferation of mesenchymal stem cells (MSCs) and osteogenic differentiation when compared with other PHA and PLA [Citation4]. Since other similar PHA have been used to prepare smooth/rough-surface microspheres as cells or drugs carriers [Citation7–12], PHBVHHx had not yet been used for the same purposes.
Except films and scaffolds [Citation13–15], polymer microspheres have been exploited as micro-carriers for cell therapy and supporting growth of mammal cell [Citation16–19]. Micro-carriers are small spherical beads that can be maintained in a suspended state and provide a large overall surface area onto which cells can attach [Citation20–22]. At present, there are several types of micro-carriers that are available, each with different materials, such as PLA, chitosan and polystyrene [Citation23–28]. However, traditional micro-carriers do not provide sufficient space for cell proliferation, due to the lack of metabolite and substrate exchanges, risks including cell abnormal growth, apoptosis or even cell detachment are observable. Thus, porous structures of 3 D tissue engineering scaffolds that can enhance cell attachments through the increased surface area are highly desirable [Citation29,Citation30]. The interconnected porous structure can not only enlarge the surface and internal areas, but also facilitates in- and out-transport of nutrients and metabolites for subsequent cell proliferation and differentiation [Citation30,Citation31]. A highly open porous microsphere based on PLGA were reported prepared using a modified double emulsion solvent evaporation method [Citation32–35]. The method replaces other aqueous phase with ammonium bicarbonate aqueous solution, which releases ammonia and carbon dioxide as a pore-foaming agent after emulsification. The gas bubbles led to the formation of the highly open porous structures of PLGA microspheres. When loaded with chondrocytes and hepatocytes, these porous PLGA microspheres injected into animals showed improved in vivo results compared with other microspheres [Citation36,Citation37], although the release of lactic acid accompanied with in vivo biodegradation of PLGA or PLA could be a potential risk for medical application [Citation38,Citation39].
In this study, for the first time, we attempted to fabricate porous microspheres (PMS) based on PHBVHHx using the two gas pore-foaming agents decomposed from ammonium bicarbonate. Simultaneously, PHBVHHx solid microspheres (SMSs) and hollow microspheres (HMSs) as controls were also prepared. Cell growth behaviors on these microspheres were studied and evaluated.
Materials and methods
Materials
PHBVHHx was produced and donated from the Shandong Lukang Pharmaceutical Group (Jinan, China). Pure ammonium bicarbonate was purchased from Damao Co. Ltd., (Tianjin, China). Poly(vinyl alcohol) (PVA) and Phalloidin-TRITC were obtained from Sigma-Aldrich (Los Angeles, CA, USA). Dulbecco's modified eagle medium (DMEM), fetal calf serum (FBS) phosphate buffer (PBS), penicillin and streptomycin for cell culture were purchased from Thermo Fisher Scientific Inc. (Waltham, MA, USA). Triton X-100, paraformaldehyde, glutaraldehyde solution and all other chemicals were of analytical grade. Deionized water was used throughout the experiments.
Preparations of PHBVHHx microspheres
PHBVHHx porous microspheres (PMSs) were prepared by an improved method of gas-in-oil-in-water (G1/O/W2) double emulsion assisted with a gas pore-foaming agent, allowing carbon dioxide and ammonia to be released from ammonium bicarbonate degradation [Citation32]. In details: 0.75 g of PHBVHHx was dissolved in 15 ml methylene chloride for 5 wt% concentration in the oil phase (O). The gas phase (G1) of pro-aqueous solution contained 15 g of ammonium bicarbonate and 100 ml of de-ionized water. 5 ml of phase G1 and 15 ml of aforementioned PHBVHHx/methylene chloride solution (phase O) were mixed to form a primary emulsion (G1/O) using homogenizer (T10, IKA, Germany) under 10000 rpm for 2 min. Subsequently, the primary emulsion was added dropwise to 100 ml of 0.1 wt% PVA solution under magnetic stirring at 400 rpm to form the secondary emulsion (G1/O/W2), which was continuously stirred for at least 6 h, to allow complete evaporation of the organic solvent.
In addition, PHBVHHx solid microspheres (SMS) and hollow microspheres (HMSs) were prepared by a typical oil-in-water (O/W) simple emulsion method [Citation12] and water-in-oil-in-water (W1/O/W2) double emulsion method [Citation9], respectively. For SMS, 15 ml of the aforementioned PHBVHHx/methylene chloride solution (phase O) was fed drop wise into 100 ml 0.1 wt% PVA solution under magnetic stirring at 400 rpm to form the emulsion (O/W2). For HMS, 15 ml of aforementioned PHBVHHx/methylene chloride solution as oil phase (O) was mixed with 5 ml de-ionized water as an internal phase (W1) to prepare the primary emulsion using a homogenizer at 10000 rpm. Subsequently, the primary emulsion (W1/O) was flown dropwise into 100 ml 0.1 wt% PVA solution magnetically stirred at 400 rpm to form the secondary emulsion (W1/O/W2).
All the above generated PHBVHHx PMSs, SMSs, or HMSs, were washed four times using at least 500 ml de-ionized water to completely remove residual PVA.
Section of PHBVHHx microspheres
To view internal structure of microspheres, a novel SEM sample preparation method named WEI Frozen Orderly Sectioning (WEI FOSM) was developed for this study. Briefly, the wet PHBVHHx microspheres dispersed onto glass plate were frozen at −80 °C for 5–8 min to become a complex of microspheres and ice. Subsequently, the upper part of the complex was sectioned with scalpel and the lower part was maintained in residual ice. The treated complex was frozen dried to remove water. Finally, sections of PHBVHHx microspheres were ready for SEM observation.
Morphology and structure analysis
PHBVHHx microspheres surface morphology was observed under scanning electron microscopy (SEM, Quanta 200, FEI, Czech) at an accelerated voltage of 15 kV. The diameter distribution of surface and internal pores of microspheres were recorded and analyzed by SEM image analysis software Image J (NIH, Bethesda, MD, USA), respectively. Approximately 50–300 surface pores in 20 randomly selected microspheres as parallel experimental groups were used for calculation of quantity and size. Attentively, for PMS’s size calculation, surface pores smaller than 5 μm were ignored in this study as reported by similar studies [Citation32].
The particle size analysis of PHBVHHx microspheres were carried out using a Malvern Particle Sizer (Mastersizer 2000, Malvern, Malvern, UK). All microspheres were dispersed in an aqueous solution and size analysis was conducted after sample stirring.
Water uptake ratio
Originally, dried microspheres were first wetted by immersing in 75% (v/v) ethanol, followed by being washed three times with deionized water, and subsequently incubated in phosphate buffer solution (PBS, pH = 7.0) for 12 h. The water uptake by the microspheres was measured by weighing wet microspheres after wiping the excess water on the surface. For measuring interior space or porosity of PHBVHHx PMS, the water uptake ratios (WURs) of microspheres were defined, and calculated from the following EquationEquation (1)(1)
(1) according to previous study [Citation32].
(1)
(1)
Where Wwet and Wdry are the wet weights and dry weights of the PHBVHHx microspheres, respectively. (Wwet–Wdry) is the weight of microspheres minus water uptaken by the microspheres. Dry weights of PHBVHHx porous microspheres were measured after lyophilization to completely remove residual water. Each study had 6 parallel samples (n = 6). The water uptake ratios of PHBVHHx SMSs and HMSs were also recorded using similar methods.
Cell culture
To investigate cell attachments on PHBVHHx PMSs, SMSs and HMSs as carriers in suspension cultivation system, a commonly used model human osteoblast-like cell, namely MG63 (from Shanghai Cell Bank, Chinese Academy of Sciences), was employed. The cells were cultivated in a culture medium in a carbon dioxide incubator (Heal Force, HF240, Beijing, China) supplied with 5.0% of CO2 at 37 °C. The culture medium consisted of DMEM supplemented with 10% (v/v) FBS, 100 units/ml penicillin, and 100 g/ml streptomycin.
Cell attachments
Ten milligrams (dry weight) of PHBVHHx PMSs, SMSs and HMSs were transferred to 48-well tissue culture plates (TCPs) coated with sterile agarose, respectively. The use of agarose effectively separated cells and TCPs, promoting cell adherence on microspheres and avoiding non-specific cell proliferation on TCPs, to simulate suspension culture system [Citation40]. Prior to the cell cultivation process, PHBVHHx PMSs and the controls including SMSs and HMSs were sterilized via immersing the microspheres in 75% (v/v) ethanol overnight. Subsequently, all samples were washed at least three times with phosphate buffered saline (PBS) and at least twice with DMEM without FBS.
The 2 × 106 MG63 cells in 0.8 ml DMEM were seeded on PHBVHHx microspheres and cultivated for a half day at 37 °C under 5.0% of CO2. After 12 h of incubation, the non-adhesive cells were removed with original DMEM and washed with fresh DMEM for 3 times. Consequently, the microspheres loaded with cells were moved to a new 48-well TCP without agarose. All samples was immersed in 400 μl fresh DMEM containing 10% (v/v) CCK-8 solution (Fanbo, Beijing, China) for 1 h, allowing investigation on cell attachments to PHBVHHx PMSs, SMSs and HMSs, respectively, as reported previously [Citation41]. The 200 μl supernatant was then transferred to 96-well TCPs and the absorbance at 450 nm was determined using a Thermo Scientific Varioskan Flash (Thermo, Waltham, MA, USA). For each sample, 6 parallel experimental groups were measured to assess the cell viability.
Cell proliferation
To study cell proliferations, 10 mg (dry weight) PHBVHHx microspheres including PMS, SMS and HMS, were firstly seeded with 5 × 105 cells in 0.8 ml DMEM, followed by cultivation at 37 °C under 5.0% of CO2 for one day. After 24 h of cultivation, the non-adhesive cells were removed with original DMEM and washed with fresh DMEM only once. The PHBVHHx microspheres loaded with cells were removed to the 6-well TCPs coated with sterile agarose from 48-well TCP, so as to obtain more space and more DMEM for cell proliferation. The cells were cultivated uninterruptedly on PHBVHHx microspheres for 6 days, including the first 24 h in 48-well TCP. The DMEM was replaced using fresh DMEM, and all samples were re-suspended under shaking of 6-well TCP every 6 h to facilitate mass transport of nutrients and oxygen for better cell proliferation. Similarly to cell attachments, all microspheres loaded with cells were removed to a new 48-well TCP without agarose for cell proliferation study. All samples were immersed in 400 μl fresh DMEM containing 10% (v/v) CCK-8 solution for an hour. 200 μl supernatant was then transferred to a new 96-well TCP and the absorbance at 450 nm was determined using the Thermo Scientific Varioskan Flash (Thermo, Waltham, MA, USA). For each sample, 6 parallel experimental groups were used to assess the cell viability. 5 × 105 cells per 10 mg were used for cell proliferation study instead of 2 × 106 cells as this is a reasonable cell-seeding density for a long time cell growth to avoid over-growth of cells in a later period of time that may lead to overlapping cell layers.
Confocal laser scanning microscopy study
Distributions of MG63 cells on PHBVHHx microspheres including PMS, SMS and HMS were observed under confocal laser scanning microscopy (CLSM, Leica, TCS SP5, Wetzlar, Germany). Briefly, the cells on PHBVHHx microspheres were firstly removed to a new 48-well TCP without agarose and washed with PBS twice. These PHBVHHx microspheres loaded with cells were fixed using 4% paraformaldehyde solution (in PBS) for 20 min at room temperature, and then washed three times with PBS. Subsequently, cells were immersed in 0.5% Triton X-100 solution (in PBS) for 20 min and then washed 3 times with PBS. To stain actin, cells were incubated with Phalloidin-TRITC (Sigma-Aldrich, Los Angeles, CA, USA) for 25 min at room temperature, and removed residual reagents with PBS to reduce the noisy backgrounds. Finally, the cell distributions (cell actins) on and in PHBVHHx microspheres for day 2, day 6 and day 10 were observed under CLSM at 516 nm (excitation wavelength).
To directly view cell distributions in internal spaces of microspheres, the PHBVHHx microspheres loaded with cells were also treated with WEI FOSM. After fixation, permeabilization and staining, respectively, the PHBVHHx microspheres loaded with cells dispersed onto glass plates were frozen and sectioned with scalpel sequentially. The treated complex of cells-microspheres was dried to remove water, allowing sections of PHBVHHx microspheres to become visible under CLSM.
Scanning electron microscopy study
Morphological studies of MG63 cells grown on PHBVHHx microspheres were performed under SEM. The PHBVHHx microspheres loaded with cells were washed three times with PBS to remove culture medium and unattached cells, and then the cells were fixed for 12 h with 5% glutaraldehyde solution overnight in a new 48-well TCP. The cells on microspheres were then dehydrated in ethanol solution with a series of concentrations of 30%, 50%, 60%, 70%, 80%, 90%, 95% and 100% (v/v), each for 15 min, respectively. They were subsequently treated under frozen drying overnight to remove residual ethanol. The morphology of MG63 cells on PHBVHHx PMSs, SMSs and HMSs were observed, respectively, under SEM at an accelerated voltage of 15 kV.
Apoptosis study
Using a Cell Apoptosis Detection Kitx (Beyotime, Beijing, China), the apoptosis of MG63 cells on PMS, SMS and HMS were examined with FITC annexin V-PI staining, respectively. The cells were treated via trypsinization for 3 min and separated from microspheres. Following collection and washing with PBS, the cells were then stained with FITC annexin V-PI based on manufacturer’s instructions and analyzed in a flow cytometer (BD Calibur, BD Biosciences, Sparks, NV, USA). The data were collected with Cell Quest Pro Software (BD Calibur, BD Biosciences, Sparks, NV, USA) for 10,000 cells for each sample and subsequently analyzed with WinMDI software (BD Calibur, BD Biosciences, Sparks, NV, USA).
On the other hand, all samples loaded with cells were stained directly with fluorescein diacetate (FDA) and PI for in-situ distribution observation of live or dead cells on PHBVHHx microspheres under a fluorescence microscope (IX83, Olympus, Tokyo, Japan).
Statistical analysis
All data are presented as mean ± SD in this study, and were analyzed by Student’s t-test using p values < .05 to determine statistical significance.
Results and discussion
Preparation and evaluation of PHBVHHx microspheres
Poly(3-hydroxybutyrate-co-3-hydroxyhexanoate-co-3-hydroxyvalerate) (PHBVHHx) together with several other PHA were found to have biocompatibility and biodegradability for some implant applications [Citation39,Citation42]. Using an improved gas-in-oil-in-water G1/O/W2) double emulsion method, PHBVHHx highly open porous microspheres (PMSs) were successfully prepared using gas pore-foaming agents as inner water phase (G1) including ammonia and carbon dioxide released from ammonium bicarbonate (). PHBVHHx PMSs had diameters ranging from 330 to 400 μm ( and ), with an open porous surface structure with large pore sizes ranging from 10–60 μm ( and ). Small pores of approximately 1–3 μm in size were found located in the vicinity of these large pores. Similar open porous structures of microspheres made from PLGA and PLA were reported [Citation32–35]. Yet this study was the first successful attempt to prepare PHA (PHBVHHx) PMSs.
Figure 1. Preparation and cell proliferation studies of solid, hollow and porous PHBVHHx microspheres, respectively (abbreviated as SMSs, HMSs and PMSs). (1) a1, b1 and c1 demonstrate how PHBVHHx SMSs, HMSs and PMSs were prepared via methods of oil-in-water (O/W), water-in-oil-in-water (W1/O/W2) double emulsion, and improved gas-in-oil-in-water (G1/O/W2) double emulsion in this study, respectively. (2) a2, b2 and c2 reveal section structures of the SMS, HMS or PMS. (3) a3, b3, a4 and b4 show cells grow only on surface of SMS and HMS, while c3 and c4 indicate cells grow both on the surface and into the internal structures of PMSs via surface open pores. Frame (1#) indicates a model of cell’s migration from surface to inner of PMS along these big pores. Frame (2#) indicates another model of cell’s carpetliked coverage on surface of PMSs. DCM: dichloromethane.
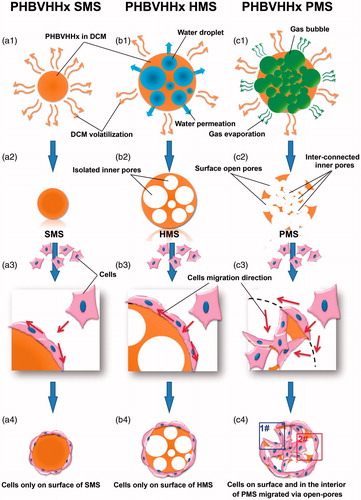
Figure 3. Characterizations of PHBVHHx SMSs, HMSs and PMSs, respectively. (a–c) Microsphere size distribution; (d) Microsphere diameter distributions of internal pores and (e) Microsphere water uptake ratio (WUR). The WURs of microspheres were calculated using 6 parallel samples for each data, ***p < .005 (n = 6 or 300). The diameters of approximately 300 surface pores and 300 inner pores from 20 randomly selected microspheres were collected for each sample (n = 300). Surface pores below 5 μm in size of PMSs were ignored.
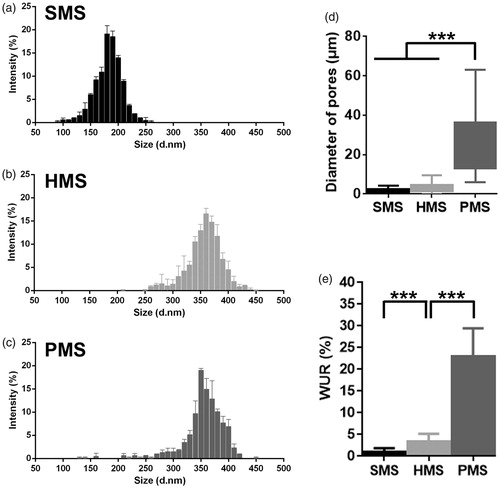
As controls, PHBVHHx solid microspheres (SMSs) and hollow microspheres (HMSs) contained porous structures with diameters of 150–220 μm and 330–400 μm, respectively, due to typical oil-in-water (O/W2) emulsion preparation () and water-in-oil-in-water (W1/O/W2) double emulsion method (), respectively. Without the internal water phase (W1), sizes of SMSs were smaller than HMSs ().
Most mammalian cells or cell agglomeration are 2–5 μm in size, they could enter large surface pores of PHBVHHx PMSs that are most >20 μm. In contrast to PMSs, SMSs and HMSs have smooth surfaces with some small pores with sizes below 2 μm (). The pore sizes were too small for the mammalian cells to enter the SMSs and HMSs.
Water uptake ratio (WUR) calculated by dividing the wet weight by the dry weight of microspheres was employed to evaluate the internal space of microspheres. WUR of PHBVHHx PMSs, HMSs and SMSs were 22.98 ± 6.49, 3.44 ± 1.75 and 0.98 ± 0.88, respectively (). PMSs had the most WUR, approximately 6.6-folds of HMSs and 23-folds of SMSs, clearly indicating that PHBVHHx PMSs had very large internal space per dry weight of microspheres. It is also consistent with SEM studies showing that PMSs had more porous spaces than that of HMSs and SMSs ( and ).
Although more internal space or better porosity of PMS is better for cell ingrowth and differentiation, a less internal space HMS is a typical form of drug carrier to package drug or protein for drug delivery purpose. Its closed inner space allows a higher and stable drug encapsulation efficiency and controllable release [Citation13–16].
Mechanisms of formation of porous microspheres and section morphology
Gas generated from decomposition of ammonium bicarbonate was surrounded by viscous PHBVHHx in organic solvent solutions prior to complete evaporation of organic solvent (). More and more gas bubbles escaped from the decomposition of ammonium bicarbonate aqueous solution droplets and entered the oil phase to increase the inner hollow space of PHBVHHx skeletal backbone. Some gas bubbles were also trapped in internal phases of oil (O) and water mixture (W2). At the end, crowded gas bubbles contributed to the highly open porous surface and internal structures with large sizes [Citation32].
If gas bubbles could not escape conveniently, water droplets as inner aqueous phase (W1) were stabilized in PHBVHHx organic solvent solution, leading to formation of HMSs (). Some small pores on HMS surfaces were results of few water droplets trapped on the interphase of oil (O) and water (W2).
To observe internal structures of PMSs, SMSs and HMSs, the microspheres were sectioned using a WEI Frozen Orderly Sectioning Method (WEI FOSM) developed in this study. Spherical hollow structures with an average inner pore size of 31.01 ± 16.70 μm were abundantly distributed all over the interior and surface of PMSs that were also interconnected (). Such highly open porous structures can effectively facilitate in- and out-transport of nutrients and metabolic wastes, thus promote cell growth on and in the microspheres (). Such open and interconnected structures could even allow cells to migrate into different parts of the microspheres.
PHBVHHx SMSs and HMSs were also sectioned by the WEI FOSM method (). The solid structure of SMSs could not provide interior space for cell proliferation, even though their surfaces allow cell attachments. In contrast, many spheroidal pores with irregular diameters were observed inside the HMSs, together with some oversized diameters of over 70 μm that were not well interconnected, as only a few surface pores with an average size of 5.32 ± 1.22 μm were connected with a few large pores (). It is therefore obvious that SMSs and HMSs do not have suitable structures to support cell proliferation on and inside the microspheres as metabolic substrates exchanges cannot be carried out conveniently ( and ).
Cell attachment and proliferation of PHBVHHx microspheres
As micro-carriers loaded with cells in suspension cultivation, the amounts of cells (or cell viability) attached on/in various PHBVHHx microspheres were assessed after 12 h of seeding using a cell counting kit (CCK-8). PHBVHHx PMSs had the highest absorbance at 450 nm (), indicating more cells attachments on and in the HPMSSs than others under the same conditions. SMSs and HMSs did not demonstrate a significant difference in cell attachment, indicating that the primary difference of cell attachment was dictated by surface structures, pore sizes and possible internal spaces rather than by the sizes of microspheres.
Figure 5. Cell attachments and proliferation on/in PHBVHHx SMSs, HMSs and PMSs, respectively. (a) Laser scanning confocal images of cells cultivated on/in PHBVHHx SMSs, HMSs and PMSs on days 2, 4 and 6, respectively. (b) Cell attachments for 12 h and (c) Cell proliferations for 6 days. n.s.: no significant difference; *p < .05; **p < .001; ***p < .005 (n = 6). LC indicates a large proportion of cells leaving their original positions to expose the microsphere surfaces. The sections of PMSs, SMSs and HMSs loaded with cells were prepared via WEI FOSM.
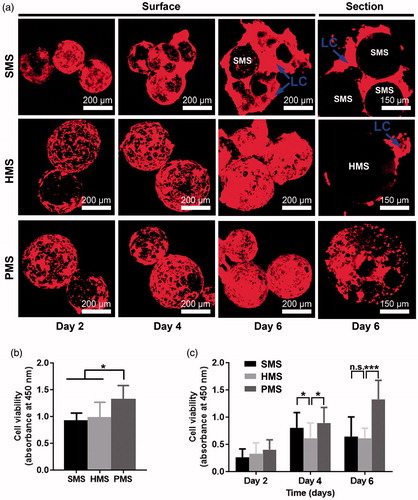
Cell proliferation in 10 days was also evaluated via CCK-8. The absorbance at 450 nm of only PMSs showed a continuous increase (). At early stage of cultivation, not significant difference among all samples was observed. Later over the cell growth period, the cell viability in or on PMSs was remarkably higher than that of HMSs and SMSs on day 4. Cell viability on SMSs and HMSs showed a typical parabola tendency, it increased during the early 4 days to reach its maximum, and weakly decreased after day 4. This is to be expected, as highly open porous structure of PMSs could afford more 3 D internal space for ingrowth of cells in addition to attachment on the surface. In contrast, the cell attachment areas of both SMSs and HMSs were limited only to their surface.
Observation of cell growth on PHBVHHx microsphere
Cells stained with phalloidin-TRITC were observed to exhibit sustained growth behaviors on surface of PMSs, HMSs and SMSs, respectively, under confocal laser scanning microscopy (CLSM) (). On the first day, cells inoculated with HMSs in 6-well TCPs showed a uniform cell distribution on microsphere surfaces, yet some cell aggregations were observed on PMSs, possibly due to rapid growth of cells both on surfaces and in pores under the surfaces. This assumption was supported by scanning electron microscopy (SEM) (). The amounts of cells on the surface of HMSs and PMSs showed increases as supported by red areas of cell groups during the 2nd day and 6th day. On day 6, some accumulated cells left from HMSs and SMSs were observed as indicated by LCs (), respectively. The phenomenon could be commonly observed for cells grown on solid cell carriers when subsequent cells showed excess growth [Citation9]. These accumulated or escaped cells are abnormal in terms of cell viability with potentially negative effects for a microsphere as a cell carrier. SEM images indicated the phenomenon (). Cell viability on SMSs increased rapidly yet turned to a weak decline. This was not observed in PMSs ().
Figure 6. SEM studies of cells proliferating on PHBVHHx SMSs, HMSs and PMSs, respectively. C indicates cells adhering on surface of microspheres. CC shows carpet-liked cell growth on surface of PMS on day 6. MC indicates cell migration into internal spaces of PMS via large surface pores. R points to the rift-liked structures on surface of HMS and SMS. LC indicates a large proportion of cells leaving their original positions to expose the microsphere surfaces.
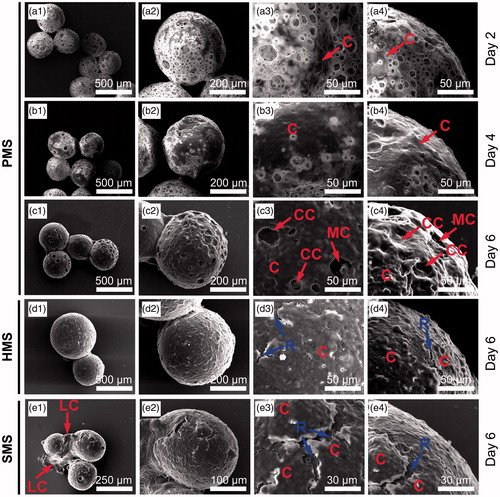
Many cells were found proliferating in PMSs but none in internal spaces of SMSs or HMSs in sections of their microspheres (), demonstrating that abundant, large and interconnected porous structures promoted ingrowth of the cells on PMSs, it is not the case with less open and small pore sizes SMSs and HMSs. This is a real 3 D spherical scaffold for cultivation and proliferation of suspended cells observed directly under CLSM.
SEM was used to study the morphology of cells on PMSs (). Initially suspended single cells were randomly adhered on surfaces of PMSs with some cells further migrated into the inner space of PMSs via large surface open pores (). After prolonged cultivation for 6 days, most cells were grown together to form confluent cell groups to cover the surfaces of microspheres (), others slowly migrated into the internal spaces of the microspheres (). Carpet-liked cells grew to cover the open pores on surfaces of microspheres (CCs in and red frame in ), while some surface pores were visible due to cell migration into inner spaces of PMS (MCs in and blue frame in ). Obviously, the confluent cells were always adhered tightly on PMSs. In contrast to PMSs, some abnormal cells aggregates (LC in ) appeared on surface of HMSs and SMSs were shown rift-liked structure (R in ) between cell confluences. Results agreed with CCK-8 study () and CLSM observation () that PMSs were better to support cell proliferation while cells tended to escape from HMSs and SMSs.
Both spherical and confluent cell morphologies on PLGA open and highly porous microspheres were reported without cell viability study using either MTT or CCK-8 [Citation32,Citation33]. The spherical cells may not be healthy enough as acid products from PLA or PLGA degradation could lower the surrounding pH. In this study, PMSs and two controls prepared from the same PHBVHHx material have better biocompatibility yet slower degradation behavior, it may be better compared with PLA or PLGA for application in tissue engineering, especially under certain circumstances of slow degradation demands [Citation4–6].
Apoptosis study of cells on PHBVHHx microspheres
Apoptosis of cells on PMSs, SMSs and HMS on day 6 of incubation was examined via FDA/PI staining under CLSM, and FITC-annexin V/PI staining for flow cytometry analysis, respectively. The live cells with FDA showed green fluorescence [Citation41,Citation43], while PI staining helps identify the necrotic and late apoptotic cells with damaged plasma membranes [Citation44]. Most red cells were visible on SMSs and HMSs while the least found on the PMSs ().
Figure 7. Apoptotic studies of cells proliferated for 6 days on solid, hollow and highly open porous PHBVHHx microspheres, respectively (abbreviated as SMSs, HMSs and PMSs). (a) The in-situ observation of living, dead or apoptotic cells on microspheres stained with FDA and PI under a fluorescence microscope and (b) comparison of percent of viable, necrotic, apoptotic cells on microspheres, respectively. n.s.: no significant difference; *p < .05; **p < .001; ***p < .005 (n = 4).
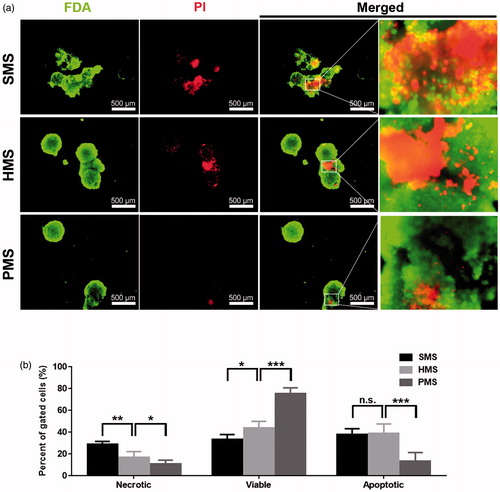
Viable, necrotic, apoptotic cells on all samples, respectively, were recorded using FITC-annexin V/PI and analyzed via flow cytometer (). Results showed the percentage of gated viable cells on PMSs was higher than that on HMSs and SMSs. In addition, the percentage of gated necrotic cells and late apoptotic cells on PMSs was lower than SMSs. In-situ observation demonstrated that vast majority of dead cells proliferated on SMSs or HMSs (). These results revealed that all microspheres could provide enough space for cell attachments and growth in the early culture stage. However, HMSs and HMSs could not provide sufficient spaces to support subsequent growing cells. Thus, cell apoptosis or even cell death could be induced, the lack of nutrient and metabolic waste exchange among these cells on HMSs and HMSs also promoted more apoptosis and cell death.
The porous structure of PHBVHHx microspheres (PMSs) with well inter-connected pores has demonstrated to be better scaffolds to support more cell proliferation compared with traditional microspheres prepared as solid microspheres (SMSs) and hollow microspheres (HMSs). The structures of PMSs facilitate in- and out-exchanges of nutrients and metabolic products for maintaining cell viability and proliferation, avoiding cell apoptosis and cell death. They can be exploited for some tissue engineering applications in suspension cultivation system.
Conclusions
Porous microspheres (PMSs) based on a biopolyester PHBVHHx were successfully prepared using an improved gas-in-oil-in-water (G1/O/W2) double emulsion method. The PHBVHHx PMSs possess open porous surface structures with interconnected inner spherical spaces as 3 D spheroidal carriers. Cells proliferated much better on and in PMSs compared with traditional solid microspheres (SMSs) and hollow microspheres (HMSs) as the highly open porous structure and large 3 D inner space facilitate not only more cell proliferation but also reduce cell apoptosis and cell death. This is due to better substrate uptakes and metabolic waste discharges of PMSs compared with less capable SMSs and HMSs based from the same PHBVHHx. PMS should be developed as a potentially 3 D polymer carrier for applications in tissue engineering and drug delivery.
Acknowledgement
The Center of Medical Analysis at Tsinghua University provided assistances in all microscopy study.
Disclosure statement
No potential conflict of interest was reported by the authors.
Additional information
Funding
References
- Khademhosseini A, Lange R. A decade of progress in tissue engineering. Nat Protoc. 2016;11:1775–1781.
- Liu X, Wei D, Zhong J, et al. Electrospun nanofibrous P(DLLA-CL) balloons as calcium phosphate cement filled containers for bone repair: in vitro and in vivo studies. ACS Appl Mater Interfaces. 2015;7:18540–18552.
- Hollister SJ. Porous scaffold design for tissue engineering. Nat Mater. 2005;4:518–524.
- Hu YJ, Wei X, Zhao W, et al. Biocompatibility of poly(3-hydroxybutyrate-co-3-hydroxyvalerate-co-3-hydroxyhexanoate) with bone marrow mesenchymal stem cells. Acta Biomater. 2009;5:1115–1125.
- Liang YS, Zhao W, Chen GQ. Study on the biocompatibility of novel terpolyester poly(3-hydroxybutyrate-co-3-hydroxyvalerate-co-3-hydroxyhexanoate). J Biomed Mater Res. 2008;87:441–449.
- Ji Y, Li XT, Chen GQ. Interactions between a poly(3-hydroxybutyrate-co-3-hydroxyvalerate-co-3-hydroxyhexanoate) terpolyester and human keratinocytes. Biomaterials. 2008;29:3807–3814.
- Chen W, Tong YW. PHBV microspheres as neural tissue engineering scaffold support neuronal cell growth and axon-dendrite polarization. Acta Biomater. 2012;8:540–548.
- Coimbra PA, De Sousa HC, Gil MH. Preparation and characterization of flurbiprofen-loaded poly(3-hydroxybutyrate-co-3-hydroxyvalerate) microspheres. J Microencapsul. 2008;25:170–178.
- Zhu XH, Wang CH, Tong YW. Growing tissue-like constructs with Hep3B/HepG2 liver cells on PHBV microspheres of different sizes. J Biomed Mater Res. 2007;82:7–16.
- Li H, Chang J. Preparation, characterization and in vitro release of gentamicin from PHBV/wollastonite composite microspheres. J Control Release. 2005;107:463–473.
- Chen J, Davis SS. The release of diazepam from poly(hydroxybutyrate-hydroxyvalerate) microspheres. J Microencapsul. 2002;19:191–201.
- Zhang SL, Zheng DJ, Fan WZ, et al. Transient embolization with microspheres of polyhydroxyalkanoate renders efficient adenoviral transduction of pancreatic capillary in vivo. J Gene Med. 2012;14:530–539.
- Osswald CR, Kang-Mieler JJ. Controlled and extended release of a model protein from a microsphere-hydrogel drug delivery system. Ann Biomed Eng. 2015;43:2609–2617.
- Xu W, Wei X, Wei K, et al. A mesoporous silicon/poly-(DL-lactic-co-glycolic) acid microsphere for long time anti-tuberculosis drug delivery. Int J Pharm. 2014;476:116–123.
- Sun G, Wei D, Liu X, et al. Novel biodegradable electrospun nanofibrous P(DLLA-CL) balloons for the treatment of vertebral compression fractures. Nanomedicine. 2013;9:829–838.
- Indolfi L, Causa F, Giovino C, et al. Microsphere-integrated drug-eluting stents: PLGA microsphere integration in hydrogel coating for local and prolonged delivery of hydrophilic antirestenosis agents. J Biomed Mater Res A. 2011;97:201–211.
- Jiang W, Bian Y, Wang Z, et al. Hepatoprotective effects of poly-[hemoglobin-superoxide dismutase-catalase-carbonic anhydrase] on alcohol-damaged primary rat hepatocyte culture in vitro. Artif Cells, Nanomed Biotechnol. 2017;45:46–50.
- Petry F, Smith JR, Leber J, et al. Manufacturing of human umbilical cord mesenchymal stromal cells on microcarriers in a dynamic system for clinical use. Stem Cells Int. 2016;2016:4834616.
- Yao L, Phan F, Li Y. Collagen microsphere serving as a cell carrier supports oligodendrocyte progenitor cell growth and differentiation for neurite myelination in vitro. Stem Cell Res Ther. 2013;4:109.
- Leong W, Lau TT, Wang DA. A temperature-cured dissolvable gelatin microsphere-based cell carrier for chondrocyte delivery in a hydrogel scaffolding system. Acta Biomater. 2013;9:6459–6467.
- Chang TMS. Therapeutic applications of polymeric artificial cells. Nat Rev Drug Discov. 2005;4:221.
- Kang SW, Jeon O, Kim BS. Poly(lactic-co-glycolic acid) microspheres as an injectable scaffold for cartilage tissue engineering. Tissue Eng. 2005;11:438–447.
- Varani J, Josephs S, Hillegas WJ. Human diploid fibroblast growth on polystyrene microcarriers in aggregates. Cytotechnology. 1996;22:111–117.
- Zou Q, Li J, Li Y. Preparation and characterization of vanillin-crosslinked chitosan therapeutic bioactive microcarriers. Int J Biol Macromol. 2015;79:736–747.
- Fang J, Zhang Y, Yan S, et al. Poly(L-glutamic acid)/chitosan polyelectrolyte complex porous microspheres as cell microcarriers for cartilage regeneration. Acta Biomater. 2014;10:276–288.
- Chang TMS. Semipermeable microcapsules. Science. 1964;45:524–525.
- Lao L, Tan H, Wang Y, et al. Chitosan modified poly(L-lactide) microspheres as cell microcarriers for cartilage tissue engineering. Colloids Surf B Biointerfaces. 2008;66:218–225.
- Zhang L, Pan J, Li J, et al. Studies on the preparation of chitosan microcarriers cross-linked by oxidized lactose and culture of primary hepatocytes. Artif Cells Blood Substit Immobil Biotechnol. 2003;31:293–301.
- Rivet CJ, Zhou K, Gilbert RJ, et al. Cell infiltration into a 3D electrospun fiber and hydrogel hybrid scaffold implanted in the brain. Biomatter. 2015;5:e1005527.
- Marino G, Rosso F, Cafiero G, et al. Beta-tricalcium phosphate 3D scaffold promote alone osteogenic differentiation of human adipose stem cells: in vitro study. J Mater Sci Mater Med. 2010;21:353–363.
- Im GI, Kim HJ, Lee JH. Chondrogenesis of adipose stem cells in a porous PLGA scaffold impregnated with plasmid DNA containing SOX trio (SOX-5,-6 and -9) genes. Biomaterials. 2011;32:4385–4392.
- Kim TK, Yoon JJ, Lee DS, et al. Gas foamed open porous biodegradable polymeric microspheres. Biomaterials. 2006;27:152–159.
- Chou MJ, Hsieh CH, Yeh PL, et al. Application of open porous poly(D,L-lactide-co-glycolide) microspheres and the strategy of hydrophobic seeding in hepatic tissue cultivation. J Biomed Mater Res A. 2013;101:2862–2869.
- Kang SW, La WG, Kim BS. Open macroporous poly(lactic-co-glycolic Acid) microspheres as an injectable scaffold for cartilage tissue engineering. J Biomal Sci-Polym E. 2009;20:399–409.
- Shi X, Sun L, Jiang J, et al. Biodegradable polymeric microcarriers with controllable porous structure for tissue engineering. Macromol Biosci. 2009;9:1211–1218.
- Ishikawa K, Takagi S, Chow LC, et al. Behavior of a calcium phosphate cement in simulated blood plasma in vitro. Dent Mater. 1994;10:26–32.
- Takechi M, Ohta K, Ninomiya Y, et al. 3-dimensional composite scaffolds consisting of apatite-PLGA-atelocollagen for bone tissue engineering. Dent Mater J. 2012;31:465–471.
- Kim TG, Chung HJ, Park TG. Macroporous and nanofibrous hyaluronic acid/collagen hybrid scaffold fabricated by concurrent electrospinning and deposition/leaching of salt particles. Acta Biomater. 2008;4:1611–1619.
- Chen GQ. A microbial polyhydroxyalkanoates (PHA) based bio- and materials industry. Chem Soc Rev. 2009;38:2434–2446.
- Verrier S, Bareille R, Rovira A, et al. Human osteoprogenitor responses to orthopaedic implant: mechanism of cell attachment and cell adhesion. J Mater Sci Mater Med. 1996;7:46–51.
- Niu J, Lunn DJ, Pusuluri A, et al. Engineering live cell surfaces with functional polymers via cytocompatible controlled radical polymerization. Nature Chem. 2017;9:537–545.
- Chen GQ, Wu Q. The application of polyhydroxyalkanoates as tissue engineering materials. Biomaterials. 2005;26:6565–6578.
- Qiu X, Zhang Y, Zhao X, et al. Enhancement of endothelial differentiation of adipose derived mesenchymal stem cells by a three-dimensional culture system of microwell. Biomaterials. 2015;53:600–608.
- Allen RT, Hunter WJ, Agrawal DK. Morphological and biochemical characterization and analysis of apoptosis. J Pharmacol Toxico. 1997;37:215–228.